DOI:
10.1039/C2RA20567J
(Paper)
RSC Adv., 2012,
2, 7809-7823
NaA zeolite cubic crystal formation and deformation: cubes with crystalline core, simultaneous growth of surface and core crystals, and layer-by-layer destruction†
Received
4th March 2012
, Accepted 10th June 2012
First published on 13th June 2012
Abstract
The entire sequence of NaA zeolite crystal growth mechanism, involving the formation of initial inorganic precursor gel, NaA zeolite nucleation, primary intermediate crystalline building block formation followed by their successive oriented aggregation and then finishing with cubic NaA zeolite crystal formation, has been monitored by light scattering analysis (LSA), X-ray diffraction (XRD), Fourier-transform infrared (FT-IR) spectroscopy, scanning electron microscopy (SEM), transmission electron microscopy (TEM), atomic force microscopy (AFM) and N2 adsorption–desorption. The presented crystal growth mechanism in this work not only provides a prominent feature in NaA zeolite cubic crystal formation but also in the deformation of cubes. The deformation of micron-size NaA zeolite cubes first involves layer-by-layer destruction followed by complete breakage of cubes due to core–shell crystal expansion, resulting in nanosize cubic crystals having the same characteristics as the original NaA zeolite before destruction. Therefore, the present study provides a first demonstration about an occurrence of multiple phenomena during the NaA zeolite crystal growth process such as formation of intermediate crystalline building blocks which are structurally similar to NaA nuclei, multiple nucleation (crystalline island formation), heat facilitated fast oriented aggregation, formation of chamfered edged cubic crystals, successive layer-by-layer destruction and finally breakage of cubic crystals. Furthermore, LSA, SEM and TEM results reveals the formation of nanocrystals with size ranging from 50 to 500 nm during the crystal growth process.
Introduction
Several researchers and material scientists have proposed different mechanisms for zeolite nucleation and growth which involves the aggregation of particles.1–6 Much of this speculation arises from recent work on “clear solution” syntheses in the tetrapropylammonium (TPA)-silicalite system. The basic argument involved in their findings is the aggregation of primary sub-colloidal particles having diameter 3–4 nm and formation of amorphous clusters by densification in a series of steps. After this, the densified clusters aggregate to particles which display crystallinity.
Mintova et al.7 proposed the growth mechanism of zeolite A evolving from the nuclei inside the amorphous gel. According to them the particles gradually grow into larger crystals by consuming the surrounding amorphous gels and there was only one single zeolite crystal per amorphous gel particle. Thus as per their findings the aggregation of several nuclei is clearly not essential to achieve crystallization. On the other hand, Chen et al.8 suggested that crystal growth can also follow a reversed route or so-called NARS route (nanoparticles, aggregation, surface re-crystallization, single-crystal). According to this route, crystallization starts on the surface of aggregates of nanoparticles to form a crystalline polyhedral shell filled with disordered core particles and then extends from the surface to the core. This was first demonstrated in the step-by-step crystal growth of analcime.8 Later, this reversed crystal growth mechanism was also observed in the synthesis of hollow sodalite spheres and zeolite A crystals.9 Moreover, Yao et al.10 found that cubic zeolite A particles prepared in the presence of chitosan contain a very thin crystalline shell whereas their cores remain amorphous and the involved surface-to-core crystallization is induced by chitosan networks. As an extension of this work, Greer et al.11 has demonstrated that a characteristic polyhedral shape of zeolite particles is not necessarily an indication of their single crystal nature. According to them, a single crystalline cubic shell can be developed on the surface of amorphous aggregates. When the core shell structure forms, nucleation of other zeolite type (sodalite) can occur in the amorphous core, followed by the crystal growth of sodalite particles and the phase transformation from zeolite A to sodalite.
Traditional hydrothermal synthetic methods play an important role in the controlled synthesis of zeolites.12 There are two basic processes involved in crystal growth in solution systems. One is the so-called oriented aggregation process while the other is Ostwald ripening.13,14 In the latter, it is assumed that crystal growth occurs via atom-by-atom addition to the existing nuclei and the morphology of a polyhedron is normally the end result.13 According to the Bravais–Friedel–Donnay–Harker (BFDH) law15–17 and Hartman–Perdok approach,18 the as-grown crystal morphology is dominated by the slow-growing faces. However, in an oriented aggregation process, primary meso-, micro- or nanostructured building blocks are organized to share an identical crystallographic orientation and join at the plane interfaces to form single-crystalline architectures.14
Although a core–shell structure after surface crystallization was found in zeolite A synthesized in the presence of chitosan,10 and the microstructure analysis of the zeolite A specimens before and after the crystallization has also been investigated and reported,11 however, there was no direct proof in NaA zeolite synthesis which showed the detailed process of recrystallization. Moreover, it is also very difficult to conclude whether the recrystallization takes place from the surface of the aggregated primary particles to the core or in the reverse direction, or whether crystallization of individual primary particles occurs. Moreover, Subotic et al.19 have reported that during the synthesis of zeolite A with extended reaction time under constant synthesis conditions, the phase of zeolite A fully transforms to sodalite type zeolite. By using X-ray diffraction they proposed that this transformation includes several steps; dissolution of zeolite A particles, supersaturation of the liquid phase with aluminosilicate ions, nucleation of hydroxysodalite from the solution and crystal growth of sodalite. Greer et al.11 also made recent investigations on the crystal growth of zeolite A and the phase transformation mechanism from zeolite A to sodalite within the polymer network. In literature we have found some contradictory observations to the above findings which omit the concept of phase transformation during the crystal growth mechanism of zeolite A. In 1984 Burriesci et al.20 reported some degree of inter-conversion existing between zeolite A and hydroxysodalite. However, as the reaction proceeds further they found that hydroxysodalite is finally converted to zeolite A. Further, Tassopoulos and Tompson21 also suggested that the metastable transformation depends upon the batch composition and total residual content in the reaction mixtures. Their experimental results emphasize that in some batch compositions, transformation of NaA zeolite to sodalite might never occur, and those only favor NaA zeolite crystallization.
From the above it is clear that in the case of relatively simple zeolite A while there is still a certain consensus on its cubic crystal formation and crystallization, in the case of NaA zeolite cubic crystal synthesis using inorganic precursors it can be expected that the detailed process of crystal growth might be much more complex, complicated and different from that which has been discussed in the literature. This creates an urgent need to study the crystal growth mechanism to confirm whether any phase change occurs or whether simply there is a growth of primary aggregated particles to final NaA zeolite crystallites. For this it is most important to observe the morphology transformations of NaA zeolite at different crystal growth stages and record the detailed process of crystallization. The main reason to choose NaA zeolite as the target material is that this zeolite finds applications almost in every field such as petrochemical industries, dehydration of organic solvents, adsorption, membrane science, sensors, and even in medical diagnostics.
The prime objective of this work is to examine whether there exist any other zeolitic phases such as sodalite during the crystallization by keeping the hydrothermal solution for extended times under the same reaction conditions. Herein, we have reported the complete crystal growth process and efforts have also been made to explain changes occurring in the NaA zeolite particles at different stages of crystallization using light scattering, XRD, infrared, electron microscopic and N2 adsorption–desorption techniques. This paper also reports the simultaneous synthesis of nanosize and micron-size NaA zeolite. To the best of our knowledge this is the first reported work on NaA zeolite cubic crystal formation with fast crystallization rate, crystalline intermediate building blocks formation, aggregative growth, cubes with crystalline core, simultaneous growth of surface and core crystals, layer-by-layer destruction and breakdown of micron size cubic NaA zeolite crystals to nanosize zeolite crystals. The other authoritative feature of this study is that during the course of NaA zeolite cubes synthesis nowhere we have used any organic precursor, organic additives, structure-directing agents, nano-zeolitic seeds or polymeric networks.
Experimental section
Materials and synthesis process
The hydrothermal synthesis method was used to synthesize the desired NaA zeolite cubic crystals from a reaction mixture of molar chemical composition 2Na2O
:
Al2O3
:
1.7SiO2
:
132H2O. This NaA zeolite precursor mixture was produced by first mixing 500 mL of sodium aluminate (Wako Pure Chemical Ind. LTD., Japan) solution with the same volume of sodium silicate (Wako Pure Chemical Ind. LTD., Japan) solution in a specially designed Teflon-lined stainless-steel autoclave (ESI† SI1). Within a few seconds of this mixing, a thick hard gel gets formed which was stirred for 2 h at a speed of 1800 rpm with an anchor stirrer to ensure the homogeneous mixing of this highly viscous hydrogel solution. The prior 2 h mixing was made without adding alkali solution to ensure maximum nucleation. Then after 500 mL of sodium hydroxide (Junsei Chemical Co. Ltd., Japan) solution was added slowly to the resultant hydrogel mixture at the same stirring speed to result in sodium aluminosilicate gel, the resultant reaction mixture was then sealed in the same autoclave, and agitated for further 22 h in nitrogen atmosphere with 1800 rpm stirring at temperature (298 K) and pressure (1 bar). After 24 h of total aging, crystallization was performed by heating the sodium aluminosilicate gel at 373 K with 1000 rpm stirring speed at constant pressure. The heating profile during crystallization is presented in ESI† SI2.
Characterization
To study the crystal growth and the changes occurring in the reaction mixture, 25 mL aliquots were taken out from the precursor gel at different intervals during the aging as well as the crystallization process. A solid product was recovered from each aliquot by high speed centrifugation and redistribution in DIW in an ultrasonic bath. For each sample at least four washings were carried out. After that samples were dried at 333 K and stored in powder form for further use. The final specimens were abbreviated as per their aging (ag) and crystallization (cr) times in hours as follows; NaA0ag (0 h ag), NaA2ag (2 h ag), NaA3ag (3 h ag), NaA7ag (7 h ag), NaA22ag (22 h ag), NaA0cr (0 h cr), NaA0.5cr (0.5 h cr), NaA1cr (1 h cr), NaA2cr (2 h cr), NaA3cr (3 h cr), NaA4cr (4 h cr), NaA5cr (5 h cr), NaA7cr (7 h cr), NaA9cr (9 h cr), NaA24cr (24 h cr), NaA48cr (48 h cr), NaA72cr (72 h cr), NaA96cr (96 h cr), NaA120cr (120 h cr), NaA144cr (144 h cr), NaA168cr (168 h cr) and NaA192cr (192 h cr).
The particle size and particle size distributions were measured by light scattering analysis (Microtrac, Zetatrac NPA152) at 298 K. The light provides a beam of wavelength (λ) at 780 nm which is scattered by the velocities of the particles so that the signal frequency shifts according to the Doppler effect. The power spectrum of the interference signal was calculated by a high-speed fast Fourier transform digital signal processor and inverted to give the particle size distribution (PSD). The PSD values in volume percent were calculated by Microtrac FLEX 1053 operating software. Powder XRD studies of NaA zeolite were made on a Rigaku D/MAX 2500 diffractometer with Cu-Kα radiation (λ = 1.5418 Å) at 40 kV and 100 mA. The data was collected in 2θ range 5–60°, with a step size of 0.02° s−1. Phase identification was performed with the help of JCPDS file (PDF#97-002-4901) for inorganic compounds. The percentage crystallinity of the as-synthesized NaA zeolite material was calculated from the XRD pattern by using eqn (1):
| Crystallinity % = ([INaA − Ia]/[Ic − Ia]) × 100 | (1) |
where
INaA is the integrated intensity for as-synthesized NaA
zeolite at 2
θ 29.9° and
Ia is the integrated intensity when we have completely amorphous nature and
Ic is the intensity of commercial NaA
zeolite sample at 2
θ 29.9°. Commercially available NaA
zeolite (Molecular sieves, 4A, powder, <5 μm, #233668, Batch#12418MD, Sigma-Aldrich) was used as a standard to make comparative studies.
Additional information concerning the NaA zeolite framework formation during the growth process was obtained by further research using Fourier-transform infrared spectroscopy. FT-IR spectra of specimens were recorded on a JASCO FT/IR-6100, in the range 400–2000 cm−1. In addition surface morphological studies of NaA zeolite particles were also made with a scanning electron microscope (SEM, Hitachi, S-4700). Transmission electron microscopic (TEM), high-resolution transmission electron microscopic (HR-TEM) images and selected area electron diffraction (SAED) patterns were obtained on a FEI Tecnai™ G2 F30 electron microscope, operating at 300 K. AFM images were acquired using PSIA XE-100 scanning probe microscope. Images were recorded using scanning mode. N2 adsorption–desorption measurements were performed at 77.4 K with a Micromeritics ASAP 2010. Before measurements, samples were degassed at 523 K for 3 h. N2 adsorption–desorption measurements were recorded only on NaA1cr, NaA2cr, NaA48cr and NaA192cr crystalline samples. Brunauer–Emmet–Teller (BET) surface areas were calculated from the adsorption branch in the relative pressure range from 0.05 to 0.30. A 60 point N2 adsorption–desorption isotherm was measured in each case and used for calculation of total pore volume as well as the average diameter of mesopores. The total pore volume was calculated by measuring the amount of adsorbed nitrogen at 0.97 P/Po. The pore size distribution of mesopores in the NaA zeolite samples were calculated using the Barret–Joyner–Halenda (BJH) method. Apart from these adsorption studies the N2 adsorption data also fitted to the Langmuir isotherm to calculate the Langmuir surface area, the Harvath–Kawazoe (HK) method for maximum pore volume and median pore diameter studies, and Dubinin–Astakhov (DA) method for the estimation of limiting micropore capacity, equivalent surface area and mean equivalent pore diameter.
Results and discussion
Light scattering analysis (LSA)
The specimen suspensions in water were initially characterized by LSA and the results were reported in terms of mean diameter, %tile of particle size and PSD. From this compiled LSA data, here, an attempt was made to clearly understand the existence and formation of particles having different nature, size, shape and morphology caused by different phenomena such as agglomeration, crystallization, re-crystallization, phase changes, crystal packaging etc., occurring during crystal growth process. LSA data in terms of mean particle size diameter and %tile of particle size (ESI† SI3 and SI4) reveals that smaller size particles were observed at the initial stage of crystallization. This is due the formation of primary gel particles and agglomerates during aging. The particle population increases in the initial 1 h of crystallization. Between 2–3 h crystallization time, the particle size grows almost three times larger (ESI† SI3) than the primary particles observed at the start of crystallization. This sharp increase in particle size may occur due to fast agglomeration and subsequent formation of NaA zeolite cubic crystals. A close observation of mean particle size data (ESI† SI3) reveals that larger particles settled down very slowly (as these appear during the 4th and 5th scans of specimens NaA2cr and NaA3cr, ESI† SI3 and SI4) which in turn indicates the presence of low density, light weight aggregated particles along with cubic crystals. Further, a small decrease in particle size may be due to the dense packing of core primary particles of cubic crystals. Mean particle size data (ESI† SI3 and SI4-NaA7cr) shows that at 7 h crystallization time, the maximum particles were of same nature but after 9 h crystallization period, a large fraction was composed of nanosize crystals (ESI† SI3 and SI4; NaA9cr). The particle size data obtained after 72 h crystallization time (ESI† SI3 and SI4) reveals that majority of the specimens contain micron size crystalline particles (fast settling of suspension took place).
All these observations become clearer when the PSD data was analyzed. Curves representing PSDs of the specimens collected at different crystallization times are presented in Fig. 1. After 24 h aging or at the start of crystallization, bimodal distribution curves obtained for NaA0cr and NaA0.5cr show the existence of two kinds of particles, mainly nanosize primary particles along with different size agglomerates formed during the aging step. During the 0.5 to 1.0 h crystallization period i.e., in 30 min time duration, there is fast self-assembling of primary particles (intermediate crystalline building blocks) to form cubic NaA zeolite crystals. This is reflected by the bimodal curve (Fig. 1; NaA0cr and NaA0.5cr) changing to unimodal (Fig. 1; NaA1cr) at 1 h crystallization time. This unimodal nature of PSD curve continues up to 7 h crystallization with broadening which may be due to the growth of NaA zeolite cubes by the addition of primary crystalline intermediate particles (PCIP) to growing cubes. Further, the crystal growth mechanism of NaA zeolite is seen to become more interesting when light scattering data shows the presence of a large amount of nanosize particles in NaA9cr and NaA24cr specimens. The appearance of such a large amount of nanosize particles in these specimens (Fig. 1 and ESI† SI3 and SI4) leads to interest about their origin. Earlier, LSA data obtained at 5 and 7 h crystallization period does not show many nanosize particles but in case of NaA9cr and NaA24cr these particles were present in large amount (ESI† SI4-NaA9cr and SI4-NaA24cr). It therefore seems their appearance in the NaA9cr and NaA24cr specimens originates from the cubes. The supporting evidences for this assumption and mechanism are provided later in this paper. This kind of phenomenon has never seen before and this fact has not reported yet in any published data on NaA zeolite. In literature it has been found that during the crystal growth process, core side particles along with amorphous gel are released after breakage of cube crystals.9,10 However, in the present study it was observed that these particles were crystalline rather than amorphous and these were originated from the upper layer of cube after crystallization rather than core particles (later evidenced by XRD, SEM and TEM results). Further, LSA data (Fig. 1; NaA48cr) shows a decrease of these nanosize particles after 24 h crystallization period. This is presumably because of the growth and re-aggregation of these nanosize crystalline cubes during the progress of reaction. This cubic crystal growth process is nicely explained by Mintova et al.7 The specimens collected from the reaction mixture after 48 h crystallization show a narrow PSD (Fig. 1; NaA72cr–NaA192cr) in comparison to earlier collected specimens (Fig. 1; NaA3cr–NaA48cr). In the earlier collected specimens, there is a wide size distribution of cubic crystals but then after expansion of core–shell crystals, breakage of bigger cubic crystals results into the release of smaller inner side crystals. Thus from the LSA data it seems that many of the possibilities outlined above occur during the crystal growth process of NaA zeolite such as (i) aggregation and subsequent crystallization of partly ordered particles, (ii) repair of grain boundaries as well as aggregates to give overall crystallographic alignment and, (iii) cubic crystal formation and (iv) some random fusion as well as diffusion of crystallites. To explain these new observations about NaA zeolite crystal growth phenomenon, XRD, FT-IR, SEM, TEM and AFM studies have also been performed.
The XRD patterns of as-synthesized NaA zeolite samples collected at different time intervals during the aging and crystallization process are compiled in Fig. 2. The presence of small peaks (indicated by arrows) in the XRD patterns of NaA22ag and NaA0cr specimens (Fig. 2; NaA22ag and NaA0cr) signifies the existence of some crystalline phases in the hydrothermal gel which originates during aging. Freund22 have also observed some degree of crystallinity in the hydrogel during aging. At 0 h crystallization most of the product is amorphous but within 30 min of crystallization clear peaks in the XRD pattern (Fig. 2; NaA0.5cr) arises in spite of the low rate of heat transfer at that point of crystallization (ESI† SI2). After 1 h of hydrothermal treatment at 100 °C, the solid phase of the sodium aluminosilicate gel is transformed to crystalline product. Employing highly crystalline commercial NaA zeolite sample as reference, the evaluated crystalline content is found to be about 53% (Fig. 3). From Fig. 3 it can be observed that the crystallization of the amorphous gel takes place rapidly within a short time interval of 0.5 to 1 h at 100 °C. The observed large bump ranging from 2θ 17 to 35° in the background, due to the presence of amorphous character in NaA22ag, NaA0cr and NaA0.5cr is found to disappear with the appearance of sharp diffraction peaks with zero base line background in the XRD patterns (Fig. 2; NaA1cr-NaA192cr) of the specimens obtained at and after 1 h crystallization. This implies that the increase in temperature accelerates the crystallization process abruptly and >100% crystallinity (i.e. relative to commercial NaA zeolite sample) is obtained in 1–2 h crystallization time. The NaA zeolite phases are identified by comparing the obtained data with the standard reported data in the Inorganic Crystal Structure Database (ICSD). The indexing of different phases in each diffractogram was made with the help of JCPDS file (PDF#97-002-4901) and all the observed peaks in the XRD pattern (Fig. 2) were indexed to a face centered cube (fcc) structure with unit cell parameters a = b = c = 24.61 Å and Fm3
space group. The specimens obtained at different crystallization stages have similar XRD patterns which confirm that the product was pure fcc NaA zeolite without any other zeolitic phase and crystalline impurities. However, the XRD diffractograms of NaA144cr, NaA168cr and NaA192cr show two additional small intensity peaks (indicated by asterisks) at 2θ 13.9 and 24.3°. These peaks, which appear in the crystalline product after six days of continuous crystallization at 100 °C, may correspond to hydroxysodalite zeolite phase. Further these peaks may arise due to the crystallization of amorphous phase (which may have remained unreacted or unaccumulated alongside the NaA zeolite crystallites during the self-assembling and crystal growth process) after the breakage of the zeolitic cubes.
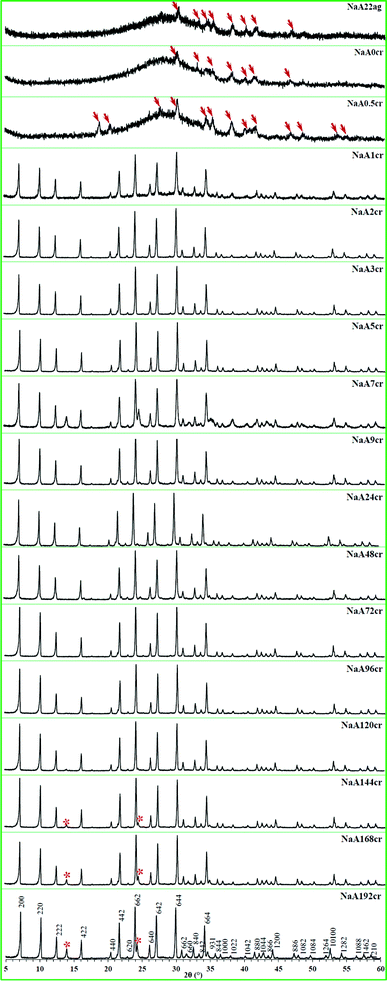 |
| Fig. 2 XRD patterns of NaA zeolite specimens taken out from the reaction mixture at different aging and crystallization times. | |
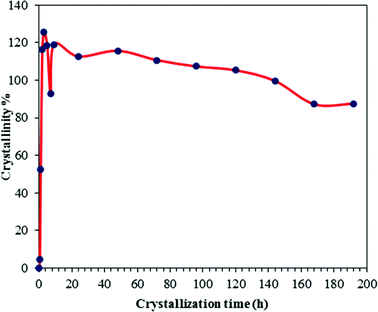 |
| Fig. 3 Crystal growth kinetics of NaA zeolite. | |
As earlier in this study we proposed that the crystalline building blocks i.e. PCIP are formed during the aging time before undergoing oriented aggregation to form NaA zeolite cubes. As such oriented aggregation would occur when crystals are attached at similar crystallographic surfaces to form a new crystal. Therefore, the series of specimens obtained during crystal growth process were examined by FT-IR, thus completing long-range order (XRD) studies with short-range FT-IR information. The FT-IR spectra of all the specimens are collectively presented in Fig. 4. The specimens collected at the initial stage of aging (0 h, 25 °C) and at 7 h of aging show similar spectra without characteristic NaA zeolite adsorption bands, indicating they are amorphous sodium aluminosilicates. These hydrogels show characteristic adsorption bands at 995 and 450 cm−1 of T–O (T = Al or Si) vibrational peaks. The band at 560 cm−1 connected with vibrations of D4R, the dominant secondary building unit in the NaA zeolite structure, was employed to evaluate the crystal growth of the NaA zeolite cubes. IR spectra of specimens obtained at 22 h of aging and at the start of crystallization (NaA0cr and NaA0.5cr) clearly show the development of the NaA zeolite characteristic absorption band at 464 (T–O bend), 560 (D4R) and 660 cm−1 (T–O–T symmetric stretching). Furthermore, the absorption bands at around 1440 and 1335 cm−1 weakened with crystallization time, indicating the amount of amorphous material in the specimen gradually decreases. This is due to the progressive transformation of amorphous phase into crystalline NaA zeolite phase. The band indicative of D4R units can be observed clearly after 1 h of crystallization time, which is in good agreement with XRD results. Furthermore, FT-IR study reveals that the crystallization of the amorphous gel into NaA zeolite starts during aging, and fast crystallization took place on thermal treatment. The hydrogel quickly crystallizes within 1 h of crystallization and all characteristic bands were intensified with crystallization time. The intensity increase of 560 cm−1 peak is accompanied by a decrease in the intensity of the 845 cm−1 band that can be assigned to Si–OH bending vibrations. The gradual disappearance of the Si–OH band is consistent with an increase in the number of framework atoms connected via oxygen bridges which is a further evidence of transformation of the amorphous material into NaA zeolite crystals.23 The IR spectra of specimens obtained between 1 to 72 h of crystallization (Fig. 4) shows a weak shoulder in the wavenumber range 550–600 cm−1, which might represent nanosize partially ordered particles.24 The LSA (Fig. 1 and ESI† SI3 and SI4) and SEM studies (Fig. 5 and 6 and ESI† SI5) also suggest similar behavior. FT-IR spectra of the NaA168cr and NaA192cr specimens show two very weak intensity peaks at 692 and 715 cm−1, which are characteristic peaks of the sodalite phase. The FT-IR studies not only corroborate the XRD results but are also in accord with the SEM and TEM observations as discussed later. The FT-IR study also confirms the formation of crystalline intermediate species with structure similar to NaA nuclei. According to the FT-IR study the crystallization of amorphous gel into NaA zeolite crystals starts at room temperature during aging. Further clear and precise evidence for the crystal growth mechanism using electron microscopic studies are given in the following section.
Scanning electron microscopic (SEM) analysis
To explain NaA zeolite crystal growth in an explicit manner, SEM images at different magnifications and areas were taken for the specimens obtained at different growth stages. Although there are some limitations of ex situ analysis techniques precautions were taken to minimize them. The obtained SEM micrographs are presented in Fig. 5 and also provided as ESI† SI5. SEM images (Fig. 5 and ESI† SI5) suggest a disparate mechanism and extraordinary phenomenon for NaA zeolite crystal growth. It is clear that after the initial mixing of precursors (Fig. 5; NaA0ag and NaA3ag) a visible gel is formed and it is envisaged as the primary amorphous phase in which NaA zeolite nucleation take place. SEM images of the sample collected at 22 h aging (Fig. 5; NaA22ag and ESI† SI5-NaA22ag) reveal that the hydrogel was composed of well dispersed isolated PCIP as well as their aggregates. Further, these images show that size of individual primary particle was about 30–100 nm. The main reason for the aggregation and self-assembling of primary intermediate particles is the use of alkaline solution for the synthesis which results in an increased electrolyte concentration and this causes condensation of PCIP present in the solution phase. The formulation of agglomerates having 4 to 6 PCIP occurs and subsequent densification of these agglomerates to bigger aggregates was clearly observable (Fig. 5; NaA0cr-NaA1cr and ESI† SI5). In these SEM micrographs we can also easily view the crystalline phase and accumulation of PCIP around these crystalline phases (cubes). This indicates the simultaneous crystallization in the core as well as on the cubic surface. This observation was also confirmed by the XRD and FTIR results (Fig. 2; NaA0cr-NaA1cr and Fig. 4; NaA0cr-NaA1cr). The crystalline parts in SEM micrographs (Fig. 5; NaA0cr-NaA1cr) of specimens obtained at 0, 0.5 and 1 h crystallization are indicated by circles. Figs. 5NaA2cr and NaA3cr show the formation of 2–3 μm sized cubic shaped crystals which are firmed up with nanosize crystalline intermediate building blocks. The chemical route by which such progressive ordering is brought about was highlighted by Flanigen and Breck25 and also by Chang and Bell.1 According to them, the anions do not act in isolation and a crucial role in the ordering process is played by the cations present in the synthesis system. These will act to attract around themselves energetically favorable coordination spheres of oxy-species and will generate certain preferred geometries. A close observation of SEM images taken at different areas and magnifications (ESI† SI5-NaA3cr) for NaA3cr shows that at this point of crystallization, the nanoparticles comprised of microcubes undergo conventional Ostwald ripening/oriented aggregation and continuous growth into cubes. SEM images obtained at 4 h crystallization (Fig. 5; NaA4cr and ESI† SI5-NaA4cr) shows the crystallization of individual upper shell primary particles. The sharp edged crystallites obtained in this specimen were shown by the inset magnified image of a selected cube at bottom-left of Fig. 5; NaA4cr. Moreover, the inset top-right image in the same micrograph shows the core shell particles in the microcube. Meanwhile the surface of the cube crystallizes into a single crystalline shell and results in the formation of a NaA zeolite cube with chamfered edges and corners. At 5 h of hydrothermal treatment unique smooth surfaced cubes with polycrystalline core (Fig. 5; NaA5cr and ESI† SI5-NaA4cr) were obtained in the reaction mixture and dimensions of cubic crystals were in the range of 1–3 μm. After 5 h crystallization, small cubic shape crystals start developing on the surface of the cubes (Fig. 5; NaA7cr-NaA24cr and ESI† SI5-NaA7cr-NaA24cr). The SEM images obtained during this crystallization time are quite interesting, informative, and very valuable to explain NaA zeolite growth mechanism. This kind of crystal growth on the upper layer or outer shell of NaA zeolite cubic crystals has never seen before and reported. The SEM results are also in good agreement with the PSD data obtained from LSA. We have seen in LSA the existence of nanosize particles in the specimens NaA9cr and NaA24cr (Fig. 1 and ESI† SI3 and SI4). Similarly, a close inspection of SEM images obtained at different magnification for these specimens (ESI† SI5-NaA9cr and SI5-NaA24cr) reveal that the surface grown crystals i.e. crystals coming out from upper layer of the microcube have cubic shape. The crystalline nature of NaA9cr and NaA24cr has already been confirmed from the XRD studies (Fig. 2; NaA7cr–NaA24cr and Fig. 3). This phenomenon occurs due to the expansion of nanosize NaA zeolite crystals lying on the surface of micron size cubic crystals by ingestion of small size NaA zeolite crystallites through an Ostwald ripening process. Therefore, under the present synthetic conditions no crystalline impurities are ever observed at any stage although layer-by-layer destruction of the large cube takes place. After separation from the surface layer of the cubic crystal these grown crystals move to solution phase which is clearly observable in the surrounding areas of bigger cubes at high magnification (top-right inset of Fig. 5; NaA9cr, and ESI† SI5-NaA7cr-NaA24cr). This process seems to be repeated again and again during the crystallization process. The SEM micrograph (Fig. 5; NaA24cr) obtained at 24 h resembles the SEM micrograph for NaA9cr (Fig. 5; NaA9cr) though at earlier time the population density of surface grown crystals is lower in comparison to the later image. After 7 h crystallization, the SEM micrographs of specimens (Fig. 5; NaA7cr–NaA72cr, and ESI† SI5-NaA7cr–NaA24cr) also shows the presence of surface embedded particles, core–shell crystallites as well as nanosize crystals present in the surrounding areas of micron size cubic crystals. An SEM image of sample collected at 48 h crystallization (Fig. 5; NaA48cr) shows the presence of two types of cubic crystals having distinctive sizes but similar chamfered edge shape. The dimensions of large cubic crystals range from 2 to 4 μm, and that of smaller ones are in the range of 0.5 to 1 μm, and both have smooth surfaces. In the same micrograph we have also noticed a small cube embedded in the bigger cube (represented by drawing a rectangle). After 72 h crystallization we again have cubes with crystals grown on the surface (Fig. 5; NaA72cr and ESI† SI5-NaA72cr) and its SEM image also confirms the co-existence of nanosize crystals in this specimen. Clearly the core–shell of the cube contains crystallites which grow with the passage of time, therefore, their simultaneous growth inside cubes affect the both the crystal size (distribution) as well as the crystal morphology (Fig. 5; NaA72cr). When the crystallization time is further increased from 72 h, cleavage of cubic crystals begins and at 96 h crystallization, the obtained SEM micrograph (Fig. 5NaA96cr and ESI† SI5-NaA96cr) indicates breakage of the cubic crystal. This segmentation of cubes is due to the expansion of core–shell crystals. When the core–shell NaA zeolite crystals expand in size, mass transformation across the crystalline shell decreases. When these core–shell crystalline particles extend outwards the pressure on the surface shell or layer will increase and results in diffusion of the outer cubic shell. The SEM image obtained for NaA120cr (Fig. 5; NaA120cr and ESI† SI5-NaA120cr) also indicates the breakage of cubic crystals from the corners. At this point of crystallization, there are only distorted shape micron size cubic crystals. Fig. 5; NaA144cr shows the complete breakage of cubic crystals. These broken cubic crystals resemble a lotus flower and further shows the three-dimensional growth of core crystals. This image also indicates that the core–shell crystallites were closely packed and arranged on one on one. Up to 192 h crystallization these cubic crystals continue to show diffusion or degradation. Smaller cubes break first in comparison to bigger ones. One of the most important observations is the multiple nucleation which occurs on the surface of cubes instead of single nucleation for each cube, and results into the formation of many crystalline islands as seen in the inset images in Fig. 5; NaA4cr, NaA9cr and NaA168cr, and ESI† SI5. In most of previous publications, multiple nucleation phenomena have not been observed.10,11,26,27
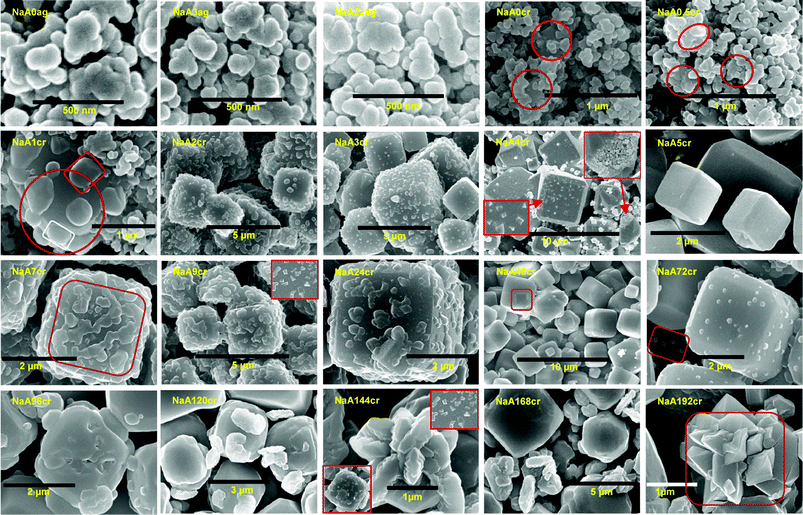 |
| Fig. 5 (NaA0ag and NaA3ag) SEM images of non-uniform aggregated gel particles at 0 and 3 h aging. (NaA22ag) SEM image showing well dispersed nanosize PCIP and aggregates after 22 h of aging. (NaA0cr, NaA0.5cr and NaA1cr) SEM images showing crystalline phase and accruement of PCIP around crystalline centres (marked by circles). (NaA2cr and NaA3cr) SEM images of NaA zeolite cubes composed of crystalline nanosize particles at 2 and 3 h of crystallization. (NaA4cr) Typical SEM image revealing the crystallization of individual PCIP (bottom-left inset) and many nanosize PCIP embedded in cube (top-right inset). (NaA5cr) Planar surface NaA zeolite cubes at 5 h of crystallization. (NaA7cr, NaA9cr and NaA24cr) Distinctive SEM images at 7, 9 and 24 h of crystallization demonstrating the growth of surface embedded nanosize crystals by Ostwald ripening process; an inset in NaA9cr shows contiguous sharp edges crystals on the cube faces. (NaA48cr) SEM image showing two different sized, smooth surface NaA zeolite cubes at 48 h of crystallization. (NaA72cr) Exemplary SEM image showing growth of surface embedded crystals and co-existence of nanosize particles as marked by rectangle. (NaA96cr) SEM image shows core crystal expansion which leads to complete cube breakage after growth for 96 h. (NaA120cr) SEM image of the specimen obtained at 120 h of crystallization, showing bigger cubic crystal breakage from corners. (NaA144cr, NaA168cr and NaA192cr) SEM micrograph evidence for the degradation of cubes, insets in NaA144cr show crystalline island formation due to multiple nucleation. | |
During the course of scanning electron microscopic studies of as-synthesized NaA zeolite samples we have also observed the existence of nanosize NaA zeolite particles along with the microsize NaA zeolite cubic crystals (shown in ESI† SI5). These scanning electron microscopic observations support the LSA data discussed earlier. SEM micrographs showing the existence of nanosize zeolite particles are collectively shown in Fig. 6 and further images are presented in ESI† SI5. These representative SEM images reveal that the particles were of nanosize with good morphology. These particles have cubic structure as generally NaA zeolite has. The size of these particles ranges from 50 to 500 nm and similar results were obtained from LSA. Furthermore, LSA data demonstrates that the samples collected at 9 and 24 h of crystallization were composed of more than 50% of nanosize particles (Fig. 1 and ESI† SI4-NaA9cr and NaA24cr). It could also be seen from the SEM images (Fig. 6) that the trend of the particle size of the nanosized NaA zeolite was the same as the results of the particle size distribution analyses presented in terms of %tile particle size in ESI† SI4. These nanosize particles are either surface grown cubic crystals from micron size cubes or unaccumulated PCIP during NaA zeolite cube formation. At the same time the possibility of their participation in the crystal growth process in the later stage by aggregation10,11 and by accumulating gel particles present in the solution phase can also not be discounted.4,7
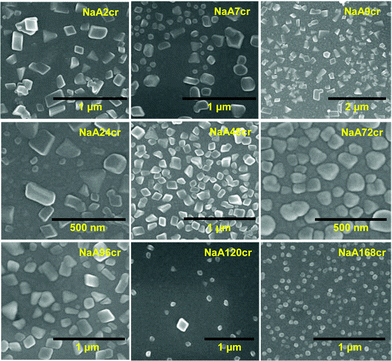 |
| Fig. 6 SEM micrographs of specimens collected at different stages of NaA zeolite crystallization revealing the formation of nanosize particles. | |
TEM investigation
Herein we have reported the formation mechanism of NaA zeolite cubes with a multiple crystalline shells along with crystalline core. This paper reports new findings in the LTA type zeolite synthesis and in contrast to previous observations reports the formation of NaA zeolite cubes with crystalline cores.
As we are reporting a new growth mechanism for NaA zeolite cubic crystals it is essential to provide as much evidence as possible to support the reported results and mechanism. Therefore in this context we have also made TEM, HRTEM and SAED studies of the specimens collected at different times of crystallization from the initial reaction system. The results of these studies corroborate the LSA, XRD, FTIR and SEM analysis. The NaA zeolite cube cores will be crystalline only if the initially aggregated primary particle are crystalline. Although already we have proved the formation of crystalline intermediate particles (PCIP), we further verify this from observed electron microscopic study results.
TEM image (Fig. 7; NaA0cr and ESI† SI6-NaA0cr) of a specimen collected at the start of crystallization or after 24 h aging at 25 °C reveals that the agglomerates were built of randomly connected particles with distorted spherical shape and size around 30–100 nm. The characteristic feature of these particles is their crystalline nature. The SAED analysis (Fig. 7; NaA0cr and ESI† SI6-NaA0cr, insets) shows the polycrystalline nature of the agglomerated gel particles. Moreover, the magnified HRTEM images (Fig. 7; NaA0cr, top-left inset) shows that NaA zeolite crystallites were embedded in these gel particles. Therefore, TEM studies confirm that the particle undergoes slow crystallization even during aging of the hydrothermal gel. The crystallization of the hydrothermal gel during aging was favored by the alkalinity of the reaction mixture. Furthermore, TEM images show that there is fast crystallization of primary particles. Within 30 min generation of crystalline particles occurs, which undergo oriented aggregation very quickly to form cubic crystals. If we closely observe the TEM, HRTEM images and SAED patterns reported in Fig. 7 then we can clearly demonstrate the NaA zeolite cubic crystal growth/formation mechanism. At 0 h of crystallization the TEM images (Fig. 7; NaA0cr and ESI† SI6-NaA0cr) shows that the specimens have both agglomerates and randomly distributed PCIP. However, at 0.5 h of crystallization, most of PCIP were in the aggregated form with different size agglomerates (shown by dotted squares in Fig. 7; NaA0.5cr). A close view and thorough analysis of these images (Fig. 7 and ESI† SI6) also reveals the existence of some PCIP with distorted cube morphology. The embedded PCIP were observable at the starting point of cubic crystal formation because of the low thickness of the outer cubic shell (Fig. 7; NaA1cr). The fringes obtained in the HRTEM images (top-left insets in Fig. 7; NaA0cr-1cr) and sharp circles and spotted patterns (bottom-right insets in Fig. 7; NaA0cr-1cr and ESI† SI6) obtained in SAED analysis prove the crystalline nature of PCIP. As HRTEM and SAED analysis have distinctly shown that the primary particles have crystalline character and these PCIP undergo aggregation to form NaA zeolite cubic crystals this affirms that the aggregated particles as well as the cores of the cubes have crystalline character. To reassert the above findings, further analysis of grown as well as broken cubes has been made. This establishes the crystalline character of broken cubes even after the breakage of cubic crystal. TEM image of NaA5cr specimen shows NaA zeolite cubes of two different sizes. The SAED (Fig. 7; NaA5cr, top-left inset) of the small cube reveals its single crystalline character whereas the SAED pattern (Fig. 7; NaA5cr, bottom-right inset) of the bigger one shows its polycrystalline nature. Because of the highly beam sensitive crystalline shell, it is very difficult to obtain HRTEM images of NaA zeolite, but after making several treatments as reported elsewhere11 we obtained good HRTEM images for these samples. The HRTEM images and SAED patterns of NaA144cr and NaA192cr (Fig. 7) specimens show that the cubic crystals were composed of a large number of nanosize crystalline NaA zeolite crystals (shown by arrows and circle in Fig. 7) which further confirms that the oriented aggregation of PCIP leads to the formation of micron size single-phase NaA zeolite cubic crystals which were composed of crystalline nanosize NaA zeolite particles. Furthermore, TEM micrographs of the specimens collected at 4 h (NaA4cr), 9 h (NaA9cr), 72 h (NaA72cr) and 96 h (NaA96cr) of crystallization show micron-size cubes along with nanosize crystals (surface grown crystals) as also seen in ESI† SI6. As insets in ESI† SI6, SAED patterns reveal the polycrystalline nature of the cubes. These SAED patterns also demonstrate that the microsize NaA zeolite cubes were composed of nanosize NaA zeolite particles. The other inset in ESI† SI6-NaA96cr, the HRTEM image, demonstrates the intimately intergrown NaA zeolite crystals.
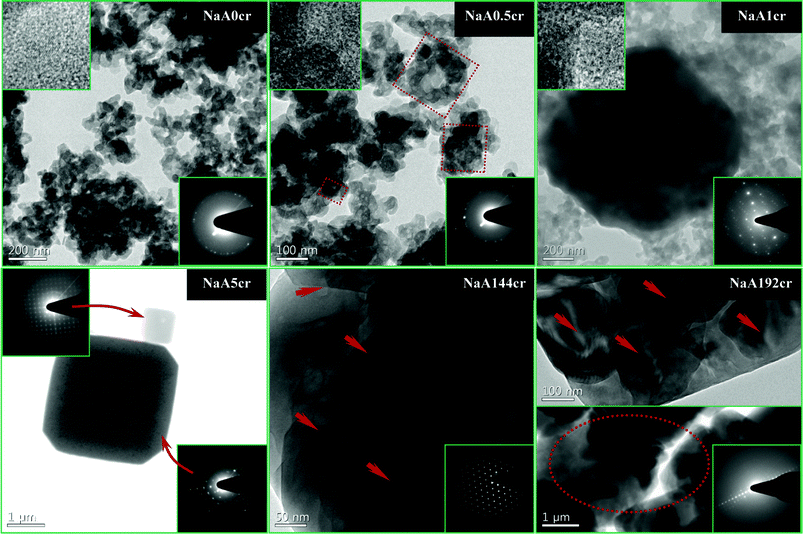 |
| Fig. 7 TEM, HRTEM images and SAED patterns of NaA zeolite specimens collected at different stages of crystallization. (NaA0cr, NaA0.5cr and NaA1cr) NaA zeolite specimens collected at 0, 0.5 and 1 h of crystallization, respectively; HRTEM images, top-left inset and SAED patterns, bottom-right insets, revealing the polycrystalline nature of particles. (NaA5cr) TEM image of specimen collected at 5 h of crystallization showing two different type of cubes; SAED patterns, top-left inset showing single crystal small cube and bottom-right inset showing polycrystalline nature of big cube. (NaA144cr) Typical HRTEM image showing that cube is composed of nanosize crystals and the corresponding SAED pattern bottom-right inset demonstrating the characteristic NaA zeolite electron diffraction pattern. (NaA192cr) TEM images of the specimen collected at 192 h of crystallization at two different magnifications show the nanosize NaA zeolite crystals embedded in the micron size cube; SAED (inset) of same solid identifying it as NaA zeolite. | |
AFM investigation
Scanning mode AFM images were used to study the surface morphology of NaA9cr specimens. As expected, phase image of the {100} surface of a cubic crystal of NaA zeolite obtained after 9 h of crystallization (Fig. 8) shows that there were surface grown particles. The corresponding AFM amplitude image reveals significant roughness. The higher peaks seen as brighter areas in the AFM image indicate the degree of surface roughness. Furthermore, the perspective-view AFM image of the {100} surface of NaA zeolite cubic crystal reveals that several fine crystals lie on the surface. The different size crystals were randomly located on the micron size cube and edges of all these particles found parallel to the micron size NaA zeolite cubic crystals on which they were grown.
N2 adsorption–desorption studies
As NaA zeolite does not absorb N2, adsorption–desorption measurements have only been made to study the changes in the morphology of NaA zeolite. Furthermore, the measured SBET will correspond to external surface area. The results of these measurements are summarized in tabular form (ESI† SI7). A narrow hysteresis at high pressure which is typical of a type IIB isotherm was observed for the NaA1cr specimen (Fig. 9; NaA1cr). The shape of the isotherm demonstrates the porous character of material consisting of agglomerates (nearly uniform) and regularly packed particles/agglomerates. The mesoporosity of the material was verified by the corresponding pore size distribution plot (see inset in Fig. 9; NaA1cr) calculated from the adsorption data using the BJH model. This mesoporous character may correspond to the interparticle spaces of aggregated PCIP. The formation of interparticle spaces or voids may be due to the loose packing of crystallites at the start of crystallization and low crystallinity of particles.28 The presence of voids and low crystalline particles in the NaA1cr zeolite specimen has been confirmed from TEM study (Fig. 10). The anomalous isotherms observed for NaA2cr and NaA48cr may be due to the existence of two different shapes, sizes and porous characteristics. The same interpretation about their nature have also predicted in LSA, SEM and TEM studies. The N2 adsorption–desorption process for NaA192cr is completely reversible with no observed hysteresis (Fig. 9; NaA192cr), characteristic of physical/surface adsorption of gases by non-porous or macroporous solids (see BJH plot inset in Fig. 9; NaA192cr). Moreover, the results of BET specific surface area measurements show a large decrease in SBET from sample NaA1cr to NaA48cr (ESI† SI7). In the earlier case the high specific surface area mainly results from the short-range primary particle organization and the observed decrease in SBET for NaA2cr and NaA48cr occurs because of the formation of large cubic crystals in the course of NaA zeolite crystallization. SBET of NaA192cr is higher than that of NaA2cr and NaA48cr because at 192 h crystallization the observed large cubic crystals break down to smaller crystals.
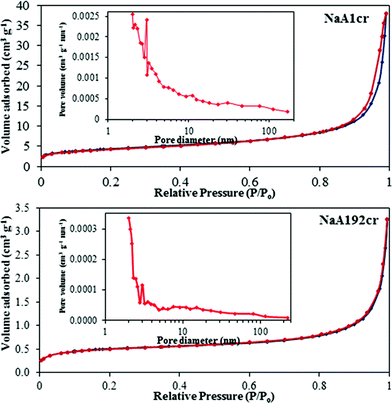 |
| Fig. 9 N2 adsorption–desorption isotherms of the as-synthesized NaA zeolite specimens with crystal growth time of 1 h (NaA1cr) and 192 h (NaA192cr). The insets are the BJH pore size distribution plots. | |
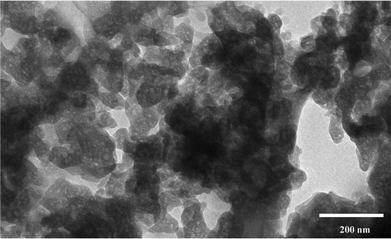 |
| Fig. 10 TEM image of NaA1cr specimen showing the presence of voids. | |
NaA zeolite cubic crystal growth mechanism
On the basis of above discussed investigations, the new crystal growth mechanism is schematically represented in Fig. 11 for NaA zeolite cubic crystal with crystalline core, and is detailed below.
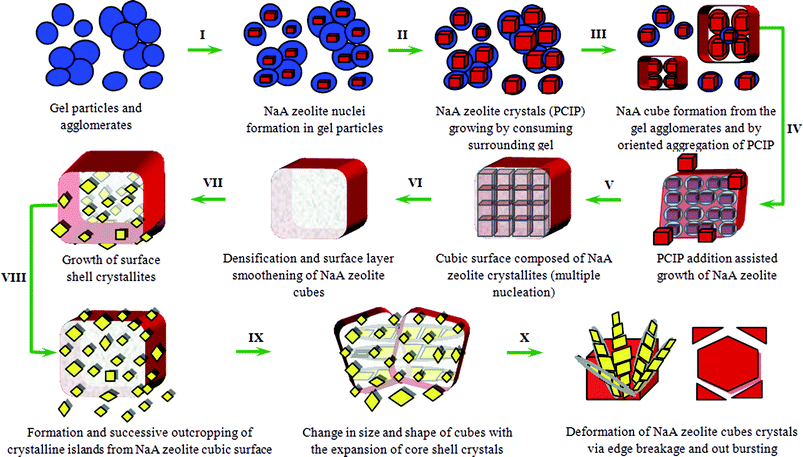 |
| Fig. 11 Schematic presentation for proposed crystal growth mechanism of NaA zeolite cubes and finally break-down to smaller size NaA zeolite crystals. | |
Step I.
Initial mixing of synthesis precursors results in a non-equilibrium and probably heterogeneous visible amorphous gel containing precipitated amorphous aluminosilicates, coagulated silica and alumina precipitates and unchanged reactants. Subsequently, the above product undergoes changes due to equilibration reactions27,29,30 with concurrent approach to equilibrium between the solid and solution phases. Under the present synthesis conditions, intermediate nanosize (30–100 nm) primary particles and agglomerates formed in the hydrogel which were clearly observable in the SEM micrograph of the NaA22ag specimen (Fig. 5 and ESI† SI5) and reflects the conversion of initially formed sodium aluminosilicate gel into apparently crystalline aluminosilicate intermediate particles via solution transport and these apparently crystalline aluminosilicate intermediate particles change to crystalline particles as the reaction proceeds.31
Step II.
The presence of sodium ions in the system leads to substantial aggregation of polymerized aluminosilicate species which in turn results a fairly open gel structure and agglomeration of PCIP.7,32 The discrete nanoparticles further aggregate into larger particles. The development of crystalline faces of primary particles was shown by the XRD patterns (Fig. 2; NaA22ag and NaA0cr) involving the appearance of {644}, {664} and {442} faces during aging. An appearance of crystalline NaA zeolite faces were also indicated by FT-IR (Fig. 4), SEM (Fig. 5 and ESI† SI5) and TEM (Fig. 7 and ESI† SI6) studies. The appearance of more crystalline faces with the passage of time indicates the uniform three-dimensional crystal growth of NaA zeolite (Fig. 5; NaA1cr and 7NaA1cr, and ESI† SI5-NaA1cr). X-Ray and electron diffraction studies were made by Tsuruta et al.33 to analyze the initially formed product during zeolite A synthesis. They isolated amorphous aluminosilicate under mild reaction conditions (60 °C, 15 min) which possesses a short-range order of Si and Al atoms similar to that in crystalline zeolite A.33 Krznarić and co-workers’ study on structural properties of aluminosilicate gel shows the presence of structurally ordered regions, or particles of a partly crystalline phase inside an amorphous matrix.34
Steps III and IV.
The agglomerated PCIP attain a crystalline cubic shape (Fig. 5; NaA1cr, 7NaA1cr, 10 and 11, and ESI† SI5) which keeps on accumulating unagglomerated PCIP by oriented arrangement on the surface (Fig. 5; NaA2cr and ESI† SI5-NaA2cr). Thus, the aggregation is a crucial step which affects the final cubic crystal size.
Preliminary discussed steps can be summarized as follows: (i) the system reaches a specific critical level of chemical evolution before the onset of crystallization; (ii) during the first crystallization stage, a crystal growth by propagation through the gel phase dominates; (iii) the second crystallization stage includes spontaneous aggregation of nano-PCIP around a crystalline center and; (iv) NaA zeolite cube formation from the agglomerates followed by an oriented aggregation/Ostwald ripening. As aggregation occurs around the crystalline part and also aggregated particles have crystalline character, so it eliminates any NaA zeolite cube having amorphous core. Hence, simultaneous crystallization of core and outer shell crystallites of NaA zeolite cubes takes place throughout the crystal growth process (Fig. 5; NaA4cr, ESI† SI5-NaA4cr and SI6-NaA4cr). The growth and crystallization rate of surface embedded crystallites is higher in comparison to core–shell crystallites because of direct heat transfer to surface crystallites and absence of any kind of surface hindrance to growing crystals.
Step V.
Polycrystalline cubic crystals composed of multiple NaA zeolite crystallites are obtained. SAED patterns presented in Fig. 7 and ESI† SI6 reveal the polycrystalline nature of the cubic crystals.
Step VI.
After surface saturation of cubic crystal further crystallization starts on the uppermost surface layer of NaA zeolite cube which results in the formation of a single crystalline cubic shell of zeolite through the involvement of multiple nucleations on the surface. An accumulation of PCIP on the surface also co-occurs with this process and finally we obtain smooth surfaced NaA zeolite cubic crystals (Fig. 5; NaA5cr and ESI† SI5-NaA5cr).
Step VII.
After obtaining smooth surfaced NaA zeolite cubes, we observe a new direction in the growth process i.e. a fresh appearance of some deposits on the cubic surface (Fig. 5; NaA7cr-NaA24cr, ESI† SI5-NaA7cr-NaA24cr and SI6). These deposits are entirely different in nature (have high crystallinity and good cubic shape morphology) than the primary amorphous particles.
Step VIII.
The concentration of the surface grown crystals increases and gives surface saturation and then movement to the solution phase (Fig. 5, 6 and 7, and ESI† SI3, SI4, SI5 and SI6). After that, once again the smooth surfaced NaA zeolite crystals are formed (Fig. 5; NaA48cr and ESI† SI5-NaA48cr). In such a way we can conclude that there is initially layer-by-layer removal of crystalline particles from the NaA zeolite cubic surface shell. Observed layer-by-layer destruction in NaA zeolite growth mechanism can be accounted for a non-negligible chance that diffused particles on cube surface have a tendency to egress back into the solution phase at any time after the initial accommodation on the surface. Thus, after surface attachment the PCIP still have a finite probability to leave the attachment sites. During crystal growth, PCIP either absorb or diffuse on the surface by finding an energetically favorable attachment site or return back to solution before binding to such a site. The latter may be caused due to disorientation of growth unit by neighboring crystallites and the probability of occurrence of any process depends on surface configuration. As the probability of the latter process increases, the surface becomes rougher and more crystals with smaller size will formed on cube surface. It should be noted that among the well-established classic crystal growth theories, the Bravais law relates the crystal facets to the crystal’s surface growth based on the assumption that crystal is developed from a single nucleus and extends outwards.
Step IX.
Simultaneous occurrence of two processes i.e. the removal of crystalline particles from the upper layer of the cubic shell of NaA zeolite cube, and the growth of core crystallites. The first process weakens the cubic NaA zeolite crystals while the second contributes to an expansion of inner crystals (Fig. 5; NaA72cr-NaA120cr, ESI† SI5-NaA72cr-NaA120cr, and ESI† SI6-NaA72cr and NaA96cr).
Step X.
The final step of NaA zeolite crystal growth mechanism corresponds to the breakage of the cubic crystals (Fig. 5; NaA144cr-NaA192cr, 7NaA144cr and NaA192cr, and ESI† SI5-NaA144cr-NaA192cr), as a result of the above two processes. This cubic crystal breakage in turn generates some deformed zeolite cubes with broken edges (and formation of hexagonal crystals) as well as some outburst NaA zeolite cubes. Due to such breakage of cubes the internal crystals pop outwards. Our study shows that the popped out crystals are NaA zeolite (Fig. 2 and 4, and SAED pattern insets in Fig. 7) which negates the existence of any kind of amorphous core and phase transformation. These obtained results indicate that our proposed crystallization mechanism is different than earlier reported mechanisms for analcime,8 zeolite ANA35 and zeolite A.11,26
The co-existence of nanosize crystals along with micron-size cubic crystals confirmed by LSA, SEM and TEM (Fig. 1, 5, 6 and 7, and ESI† SI3, SI4, SI5 and SI6) analysis coerce us to think more about these observations. As zeolite synthesis involves complex nucleation and crystal growth process, therefore, in recent years considerable progress has been made to understand zeolite crystal growth mechanisms by using advanced material characterization techniques, and correspondingly a number of growth mechanisms have been proposed for zeolite synthesis.10 Studies reported on hydrothermal synthesis of zeolite A unveil three main crystal growth mechanisms36,37 which primarily involve the formation of nanoparticles and thereafter, depending on the synthesis conditions and chemical composition, these primary nanoparticles adopt any one of three crystal growth mechanisms as schematically presented in Fig. 12. The model described in pathway I (Fig. 12) regards nanoparticles as the monomeric precursor species and the amorphous gel particles maintain their average size over the course of complete conversion into zeolite cubes which shows that the solution mass transfer is the dominant mechanism for substantial crystal growth.7 The other two mechanisms (pathways II and III) also consider the nanoparticles as zeolite precursors but these follow different growth mechanisms. Pathway II shows an addition of primary particles to growing crystals38 whereas pathway III indicates stepwise aggregation of primary nanoparticles as well as primary particles composed of crystallites resulting in large crystalline units.36 Our results suggest that (Fig. 5, 6 and 7, and ESI† SI3, SI4, SI5 and SI6) the large cubic crystals (1–3 μm) were composed of crystalline nanoparticles by aggregation (Fig. 12, pathways III). Individual small crystalline blocks with well-developed edges were clearly observable on the cube surface in SEM and TEM micrographs. At the initial stage of crystallization, SEM micrographs also reveal the existence of primary particles below a thin surface layer of cubic crystals. In other words, the initial layer-by-layer destruction of large cubes (Fig. 5 and 7, and ESI† SI5 and SI6) suggests that the formation of large cubic crystals follows a consecutive aggregation pathway. SEM and TEM micrographs for the specimens collected at different stages of NaA zeolite crystallization (Fig. 5 and 7, and ESI† SI5 and SI6) show the presence of nanosize rectangular crystals in the vicinity of large cubic crystals. These may also be intermediate building units or nano-NaA zeolite particles that follow the growth mechanism presented in pathways I or II (Fig. 12).
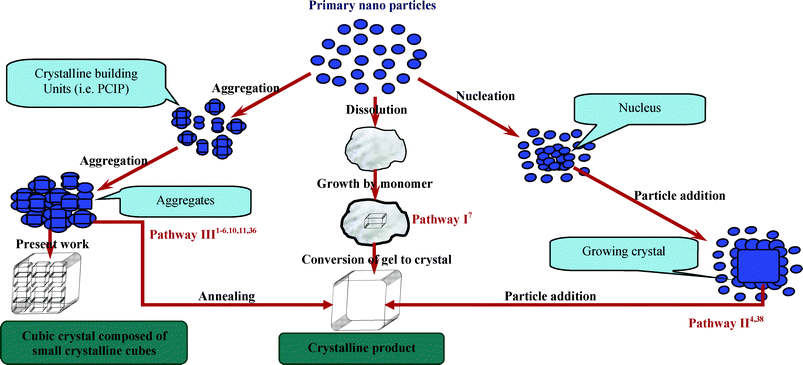 |
| Fig. 12 Schematic representation of three main models for zeolite crystal growth. | |
Conclusions
From LSA, XRD, FT-IR, SEM, TEM, AFM and N2 adsorption–desorption studies of specimens collected from reaction mixture at different stages during the growth process, it becomes clear that PCIP termed as building blocks are formed even at room temperature before their aggregation into large cubes of NaA zeolite. Thus, this fast oriented aggregation of intermediate building blocks to form cubic shaped crystals in just 30–60 min of crystallization can only take place if primary aggregated particles have crystalline character with similar crystallographic surfaces.38 The morphological studies also reveals the disappearance of the gel phase, the detection of small crystalline cubes, tilted cubes on the edges and surface of large cubic crystals and also in its surrounding area during the course of crystal growth, which clearly demonstrate that before aggregation the primary particles attain well develop crystallographic alignment and shape. As the aligning process of the agglomerated cubes is concerned this is facilitated by homogeneous heating. The results obtained in the present work provide a cornerstone to conclude that the consecutive aggregation mechanism (Fig. 12, pathway III) involving the formation of crystalline intermediate building units from primary particles and self-assembling of these into large cubic crystal is the dominating growth mechanism for NaA zeolite from inorganic precursors. The absence of any kind of zeolitic phase transformation throughout the crystal growth process is an important feature of the present work. The formation and then deformation i.e., first layer-by-layer destruction of cubes and finally their complete disintegration due to the expansion of core crystals is notable. This kind of phenomenon during NaA zeolite synthesis has never seen before in any reported paper on NaA zeolite synthesis. This work provides a new insight to study the complex NaA zeolite nucleation and crystal growth in a hydrothermal gel especially when the used synthesis precursors are inorganic monomeric silica and aluminum source. This work indicates that two prominent growth phenomena i.e. oriented aggregative and layer growth mechanism occur during NaA zeolite growth process.39,40 The formation of cubic crystals with chamfered edges and appearance of sharp crystalline peaks after just 1 h crystallization at 100 °C (Fig. 2; NaA1cr) indicates that aging of hydrogel makes the system more uniform and favors the crystallographic arrangement. The fast crystallization may occur due to homogeneous heat transfer to the hydrogel because of high speed stirring of the reaction mixture throughout the crystal growth process. Also, the aggregation processes occurring at low temperature becomes more effective as the hydrothermal treatment is adopted. Conversely, the true crystallization process will be encouraged by increasing temperature. The proposed cubic crystal NaA zeolite growth mechanism with crystalline core i.e. composed of nanosize crystalline NaA zeolite cubes (Fig. 13) has also been observed in other sets of experiments. In our recently published paper we have also demonstrated the formation of nanosize NaA zeolite crystals by using different precursor concentrations.28 Besides the above observations this paper also reveals the importance of post-cubic crystal formation studies as these studies may act as a pathfinder to develop well-shaped defect-free zeolite materials.
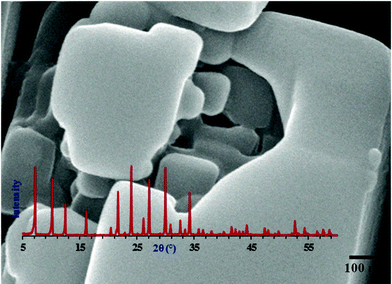 |
| Fig. 13 SEM micrograph of NaA zeolite cube synthesized in the presence of nanoseeds at very low concentration (aging temperature 25 °C, aging time 24 h, crystallization temperature 100 °C and crystallization time 3 h) obtained from the NaA9cr specimen of the present study demonstrating that the NaA zeolite cubic crystal has a crystalline core which is composed of nanosize cubes. Inset XRD diffractogram reveals that the specimen is pure NaA zeolite phase (PDF#97-002-4901). | |
Acknowledgements
The present work is financially supported by the Carbon Dioxide Reduction and Sequestration Center (CDRS), Ministry of Education and Science Technology, Republic of Korea.
References
- C. D. Chang and A. T. Bell, Catal. Lett., 1991, 8, 305 CrossRef CAS.
- S. L. Burkett and M. E. Davis, J. Phys. Chem., 1994, 98, 4647 CrossRef CAS.
- S. L. Burkett and M. E. Davis, Chem. Mater., 1995, 7, 1453 CrossRef CAS.
- P.-P. E. A. De Moor, T. P. M. Beelen, B. U. Komanschek, L. W. Beck, P. Wagner, M. E. Davis and R. A. van Santen, Chem.–Eur. J., 1999, 5, 2083 CrossRef CAS.
- P.-P. E. A. de Moor, T. P. M. Beelen, R. A. van Santen, L. W. Beck and M. E. Davis, J. Phys. Chem. B, 2000, 104, 7600 CrossRef CAS.
- V. Nikolakis, E. Kokkoli, M. Tirrell, M. Tsapatsis and D. G. Vlachos, Chem. Mater., 2000, 12, 845 CrossRef CAS.
- S. Mintova, N. H. Olson, V. Valtchev and T. Bein, Science, 1999, 283, 958 CrossRef CAS.
- X. Chen, M. H. Qiao, S. H. Xie, K. N. Fan, W. Z. Zhou and H. Y. He, J. Am. Chem. Soc., 2007, 129, 13305 CrossRef CAS.
- L. Han, J. F. Yao, D. Li, J. Ho, X. Y. Zhang, C. H. Kong, Z. M. Zong, X. Y. Wei and H. T. Wang, J. Mater. Chem., 2008, 18, 3337 RSC.
- J. Yao, D. Li, X. Y. Zhang, C. H. Kong, W. B. Yue, W. Z. Zhou and H. T. Wang, Angew. Chem., Int. Ed., 2008, 47, 8397 CrossRef CAS.
- H. Greer, P. S. Wheatley, S. E. Ashbrook, R. E. Morris and W. Zhou, J. Am. Chem. Soc., 2009, 131, 17986 CrossRef CAS.
- C. S. Cundy and P. A. Cox, Chem. Rev., 2003, 103, 663 CrossRef CAS.
- R. Boistelle and J. P. Astier, J. Cryst. Growth, 1988, 90, 14 CrossRef CAS.
- J. F. Banfield, S. A. Welch, H. Z. Zhang, T. T. Ebert and R. L. Penn, Science, 2000, 289, 751 CrossRef CAS.
-
A. Bravais, Études Cristallographic, Gauthier-Villars, Paris, 1866 Search PubMed.
- M. G. Friedel, Bull. Soc. Fr. Mineral. Cristallogr., 1907, 30, 326 Search PubMed.
- J. D. H. Donnay and D. Harker, Am. Mineral., 1937, 22, 446 CAS.
- P. Hartman and W. G. Perdok, Acta Crystallogr., 1955, 8, 521 CrossRef CAS.
- B. Subotic, D. Skrtic, I. Smit and L. Sekovanic, J. Cryst. Growth, 1980, 50, 498 CrossRef CAS.
- N. Burriesci, M. L. Crisafulli, N. Giordano and J. C. J. Bart, Mater. Lett., 1984, 2, 401 CrossRef CAS.
- M. Tassopoulous and R. W. Tompson, Zeolites, 1987, 7, 243 CrossRef.
- E. F. Freund, J. Cryst. Growth, 1976, 34, 11 CrossRef CAS.
- L. Itani, Y. Liu, W. Zhang, K. N. Bozhilov, L. Delmotte and V. Valtchev, J. Am. Chem. Soc., 2009, 131, 10127 CrossRef CAS.
- C. Kosanović, S. Bosnar, B. Subotić, V. Svetličić, T. Mišić, G. Dražić and K. Havancsák, Microporous Mesoporous Mater., 2008, 110, 177 CrossRef.
-
E. M. Flanigen and D. W. Breck, 137th Meeting of the
ACS, Division of Inorganic Chemistry, Cleveland, OH, April 1960, Abstracts, p. 33 Search PubMed.
- R. I. Walton, F. Millange, D. O’Hare, A. T. Davies, G. Sankar and C. R. A. Catlow, J. Phys. Chem. B, 2001, 105, 83 CrossRef CAS.
- C. Kosanović, T. A. Jelić, J. Bronić, D. Kralj and B. Subotić, Microporous Mesoporous Mater., 2011, 137, 72 CrossRef.
- P. Sharma, J. Yeo, D. K. Kim and C. H. Cho, J. Mater. Chem., 2012, 22, 2838 RSC.
-
S. P. Zhdanov, in Molecular Sieve Zeolites-I, ed. E. M. Flanigen and L. B. Sand, ACS Adv. Chem. Ser., 1971, vol. 101, p. 20 Search PubMed.
- G. T. Kerr, J. Phys. Chem., 1966, 70, 1047 CrossRef CAS.
-
C. L. Angell and W. H. Flank, in Molecular Sieves-II, ed. J. R. Katzer, ACS Symp. Ser., 1977, vol. 40, p. 194 Search PubMed.
- S. Mintova, N. H. Olson and T. Bein, Angew. Chem., Int. Ed., 1999, 38, 3201 CrossRef CAS.
-
Y. Tsuruta, T. Satoh, T. Yoshida, O. Okumura and S. Ueda, in New Developments in Zeolite Science and Technology, ed. Y. Murakami, A. Iijima and J. W. Ward, Kodansha–Elsevier, Tokyo–Amsterdam, 1986, p. 1001 Search PubMed.
- I. Krznarić, T. Antonić, B. Subotić and V. Babić-Ivančić, Thermochim. Acta, 1998, 317, 73 CrossRef.
- Y. Wang, X. Li, Z. Xue, L. Dai, S. Xie and Q. Li, J. Phys. Chem. B, 2010, 114, 5747 CrossRef CAS.
- D. Liang, L. R. A. Follens, A. Aerts, J. A. Martens, G. V. Tendeloo and C. E. A. Kirschhock, J. Phys. Chem. C, 2007, 111, 14283 CAS.
- V. P. Valtchev and K. N. Bozhilov, J. Am. Chem. Soc., 2005, 127, 16171 CrossRef CAS.
- T. M. Davis, T. O. Drews, H. Ramanan, C. He, J. S. Dong, H. Schnablegger, M. A. Katsoulakis, E. Kokkoli, A. V. McCormick, R. L. Penn and M. Tsapatsis, Nat. Mater., 2006, 5, 400 CrossRef CAS.
- M. W. Anderson, J. R. Agger, N. Hanif and O. Terasaki, Microporous Mesoporous Mater., 2001, 48, 1 CrossRef CAS.
- T. Wakihara, Y. Sasaki, H. Kato, Y. Ikuhara and T. Okubo, Phys. Chem. Chem. Phys., 2005, 7, 3416 RSC.
Footnote |
† Electronic supplementary information (ESI) available: Supporting LSA data, SEM, TEM images and N2 adsorption–desorption results of specimens. See DOI: 10.1039/c2ra20567j/ |
|
This journal is © The Royal Society of Chemistry 2012 |