DOI:
10.1039/C2RA20739G
(Paper)
RSC Adv., 2012,
2, 5789-5799
Synthesis, characterization and curing properties of a novel cyclolinear phosphazene-based epoxy resin for halogen-free flame retardancy and high performance†
Received
20th April 2012
, Accepted 23rd April 2012
First published on 23rd May 2012
Abstract
A novel cyclolinear phosphazene-based epoxy resin has been synthesized through a four-step synthetic route. The curing behaviors of this epoxy resin with methyl tetrahydrophthalic anhydride, 4,4′-diaminodiphenylmethane, and novolak as hardeners were investigated by differential scanning calorimetry (DSC). The thermal behaviors and stabilities were also evaluated with DSC and thermogravimetric analysis. These thermosets achieved high glass transition temperatures over 150 °C and also gained good thermal stabilities with high char yields. The flammability characteristics of the cyclolinear phosphazene-based epoxy thermosets were investigated by limiting oxygen index (LOI) and UL-94 vertical burning experiments. The high LOI values and UL-94 V-0 classification of these epoxy thermosets indicate that the incorporation of phosphazene rings into the molecular backbone imparts non-flammability to the epoxy resin as a result of the unique combination of phosphorus and nitrogen following by a synergistic effect on flame retardancy. The analysis of the residual chars collected from the UL-94 test demonstrates that cyclotriphosphazene moieties of this epoxy resin can enhance char formation during combustion serving as a barrier against heat and oxygen diffusion, and consequently the flame retardancy of the thermosets is improved significantly.
Introduction
Epoxy resins have many outstanding characteristics such as high heat, solvent and chemical resistance, low moisture absorption, good mechanical and electrical properties, excellent dimensional stability and strong adherence to many substrates. They have already gained wide applications in advanced composite matrices, surface coating, semiconductor packaging and fabrications of copper-clad laminates and printed circuit boards.1,2 In many high-tech electronic industrial fields, however, the versatile epoxy resins with excellent flame retardancy as well as high performance are necessarily required to reduce or to avoid the fire threats.3,4 In past decades, halogen-containing epoxy resins like brominated ones have been developed to meet the considerable secure requirements. Although the effectiveness of flame retardancy for halogenated epoxy resins is absolutely undoubted, a possible threat to the environment and human bodies should not be neglected. For example, flame-retardant epoxy resins usually contain brominated organic chemical structures like brominated aromatic ether, and highly toxic and potentially carcinogenic substances may form during combustion. These toxic brominated substances are very harmful to human health and also pose pollution problems when released into the environment.5 Furthermore, the combustion reaction of brominated epoxy resins can generate lots of gaseous toxic products like hydrogen bromide. In fact, all the other toxic materials coming off from a fire are in much larger concentrations than any hydrogen bromide released during an accidental fire event. In this case, considerable environmental effects and health restrictions have been considered in recent years in regard to controlling the inherent flammability of epoxy resins by incorporation of flame-retardant additives.6,7 Simultaneously, the European Union has proposed restricting the use of brominated flame retardants in electric and electronic fields.8,9 The World Health Organization and the US Environmental Protection Agency also recommended exposure limits and risk assessment of halogenated compounds.10,11
Most of the halogen-free flame-retardant materials contain phosphorus compounds,12–14 because organophosphorus molecules are efficient radical scavengers and flame quenching materials. Combustion processes are essentially exothermic free-radical reactions, and the existence of radical stabilizers impedes combustion by the quenching mechanism. Other types of flame retardants include nitrogen-containing moieties that release inert gaseous by-products to form a highly porous char that provides thermal insulation and prevents the combustion from spreading.15,16 However, the addition of these halogen-free flame retardants results in a deterioration in thermal, electric and mechanical properties, which is unacceptable for the fabrication of electronic parts. Therefore, the reactive approach, i.e. incorporation of chemical units containing phosphorus, or nitrogen, or both directly into the macromolecular backbone, is considered to be a more effective route, with the main advantage to impart permanent flame retardancy as well as maintain the original physical properties of the epoxy resins more effectively.17 Many studies have been reported for the design and synthesis of flame retardant epoxy resins by incorporating phosphorus-containing flame retarding units into their backbones such as phosphine oxide, phosphates, and the other phosphorylated and phosphonylated derivatives.18–20 However, these phosphorus-containing epoxy resins hardly gain high weight fraction of phosphorus, resulting in poor flame retardancy. Some investigations indicated that a combination of phosphorus-containing epoxy resins and nitrogen-containing curing agents led to dramatically high flame retarding efficiency due to a synergistic effect.
Phosphazenes are a unique class of chemical materials that contain alternating phosphorus and nitrogen atoms in a conjugative mode, which indicates their high non-flammability. With highly replaceable chlorine atoms linked to the phosphorus atoms of a phosphazene ring, the phosphazenes can offer a high degree of tailorability by variations in the synthetic procedures, and they can be functionalized with a broad range of polymers, which causes the originally highly flammable organic materials with auto-extinguishing.21,22 An alternative promising area of research involves the incorporation of phosphazene units into organic polymers. The properties of organic polymers can be modified significantly to improve their fire resistance, ionic conductivity, biological compatibility, or other properties by the incorporation of a small amount of a specifically tailored phosphazene.23,24 This makes phosphazenes particularly good candidates for fire-resistant materials in electric and electronic applications.25 The most widely studied phosphazene polymer systems have a linear backbone of alternating phosphorus and nitrogen atoms with two organic groups linked to each phosphorus atom, which have been employed as flame resistant materials, elastomers, membranes, solid ionic conductors, and inert biomaterials.26–28 The currently available phosphazene fire retardant additives mostly include amino or hydroxy derivatives of methyl or phenyl substituted cyclotriphosphazenes. These compounds are relatively easy to synthesize and have been reported to have useful fire retardant properties.29,30 There is also limited information in the open literature regarding epoxy thermosetting resins containing cyclotriphosphazene units, which exhibit a potential application in the electric industry for their good flame retardancy. However, when used in microelectronics, these thermosetting resins hardly obtain the overall fire resistance, because, in most cases, only a few cyclotriphosphazene units as an effective flame-retardant component can be incorporated into the molecules of the thermosetting resins through the inter-reaction.31,32 In this paper, we reported the synthesis and characterization of a novel cyclolinear epoxy resin with phosphazene rings. The introduction of cyclotriphosphazene moieties in the backbone is expected to enhance the thermal resistance, thermal stability, and most importantly, the fire resistance of the resulting epoxy materials. A complementary study on the curing properties of the prepared epoxy resin was also performed and described in this article.
Experimental
Materials
Hexachlorocyclotriphosphazene (1) with a purity of 99.6 wt% was purchased from Shanghai Yagu Chemical Co. Ltd., China. It was recrystallized from n-heptane and sublimed at 40 °C with a vacuum degree of 0.1 mm Hg before use. Phenol (2), sodium hydride (NaH) (70% suspension in mineral oil), boron tribromide (BBr3), 4-methoxyphenol (4), hexadecyl trimethyl ammonium bromide, epichlorohydrin (7), dichloromethane (CH2Cl2), tetrahydrofuran (THF), toluene, and acetone were purchased from Beijing Chemical Reagent Co., China. THF was distilled from sodium benzophenone ketal prior to use, and the other chemicals and reagents were chemical pure and used as received. A conventional epoxy resin (diglycidyl ether of bisphenol-A, DGEBA) with an epoxy equivalent weight (EEW) of 208.4 g/equiv. was kindly supplied by Wuxi Dic Epoxy Co., Ltd. Methyl tetrahydrophthalic anhydride (MeTHPA), 4,4′-diaminodiphenylmethane (DDM), and novolak were selected as hardeners and also commercially obtained from Beijing Chemical Reagent Co., China.
Synthesis and reactions
Synthesis of N3P3(OC6H5)4Cl2 (3).
1 (25.00 g, 0.072 mol) was dissolved in 100 mL of THF. 2 (31.00 g, 0.330 mol) was dissolved in 100 mL of THF and then added dropwise to a suspension of NaH (11.40 g, 0.332 mol) in 100 mL of THF. This reaction mixture was stirred at room temperature for 24 h. The resultant sodium phenoxide solution was added dropwise to the stirred solution of 1 in THF at 0 °C. This mixture was allowed to warm to room temperature to perform the reaction for 12 h. After the reaction was completed, the solvent was removed by reduced pressure rotary evaporation, and then the oily resultant solution was dissolved in 500 mL of CH2Cl2. This solution was washed four times with 500 mL of 3 wt% aqueous NaHCO3, dried with Na2SO4, and concentrated by rotary evaporation. Column chromatography was carried out in a 55 vol.% CH2Cl2/45 vol.% hexanes mixture to separate the 3 and penta-substituted compound. 3 is an off-white oil, (15.60 g, 37.6 wt% yield). 1H NMR (DMSO-d6): 6.73 (d, 8H), 6.82 (t, 4H), and 7.09 ppm (t, 8H). 31P NMR (DMSO-d6): 19.78 (d, 2P) and 4.72 ppm (t, 1P). Elemental analysis found: C, 50.03; H, 3.28; N, 7.45; Cl, 12.17. Calculated for N3P3O4C24H20Cl2: C, 49.8; H, 3.5; N, 7.3; Cl, 12.3.
Synthesis of N3P3(OC6H5)4(OC6H5OCH3)2 (5).
A solution of 4 (13.65 g, 0.110 mol) in 200 mL of THF was added dropwise to a solution of NaH (3.95 g, 0.115 mol) in 200 mL of freshly distilled THF to perform the reaction with vigorous stirring at room temperature for 24 h. The reaction mixture was added dropwise to the solution of 3 (31.20 g, 0.055 mol) in 200 mL of THF to perform the reaction with vigorous stirring at room temperature for 24 h, and then the reaction mixture was maintained at reflux at 63 °C for 48 h with stirring. After the reaction was completed, the solvent was removed from the reaction mixture by rotary evaporation. The residue was dissolved in CH2Cl2, the solution was washed with water to remove NaCl, and the solvent was removed by rotary evaporation. Column chromatography was carried out in a 20 vol.% CH2Cl2/80 vol.% hexanes mixture to get 3, which is yellow oil, (21.25 g, 51.3% yield). 1H NMR (DMSO-d6): 3.71 (s, 6H) and 7.3–6.6 ppm (m, aromatic 28H) including 7.28 (t, 8H), 7.28 (t, 4H), 6.88 (d, 8H), 6.78 (s, 4H), and 6.77 ppm (s, 4H). 31P NMR (DMSO-d6): 9.50 (d, 2P) and 9.38 ppm (s, 1P). Elemental analysis found: C, 60.29; H, 4.41; N, 5.63. Calculated for P3N3C38H36O8: C, 60.5; H, 4.5; N, 5.6.
Synthesis of N3P3(OC6H5)4(OC6H5OH)2 (6).
A solution of boron tribromide (14.15 g, 0.055 mol) in 150 mL of CH2Cl2 was added dropwise to the solution of 5 (21.25 g, 0.028 mol) in 150 mL of CH2Cl2. The reaction mixture was stirred at room temperature for 3 h and then poured into 100 mL of water. The obtained residue was washed with water three times. The solvent was finally removed by reduced pressure rotary evaporation. 6 was obtained as a brown viscous oily liquid. Yield: 83% (16.85 g). 1H NMR (DMSO-d6): 9.42 (s, 2H) and 7.3–6.6 ppm (m, aromatic 28H) including 7.28 (t, 8H), 7.29 (t, 4H), 6.86 (d, 8H), 6.65 (d, 4H), and 6.62 ppm (d, 4H). 31P NMR (DMSO-d6): 8.97 (d, 2P) and 8.87 ppm (s, 1P). Elemental analysis found: C, 59.78; H, 4.05; N, 5.67. Calculated for P3N3C36H32O8: C, 59.6; H, 4.1; N, 5.8.
Synthesis of cyclolinear phosphazene-based epoxy resin (8).
7 (99 mL, 1.260 mol) and 6 (75.0 g, 0.105 mol) was mixed in a three-necked flask with a stirrer, reflux condenser, and a nitrogen inlet, and 2% hexadecyl trimethyl ammonium bromide as a catalyst was added. The reaction mixture was stirred at 90 °C for 2 h, and then the NaOH solution (12.60 mL, 40 wt%) was added. The reaction mixture was continuously stirred for 3 h at 98 °C. The mixture containing the final product was dissolved in toluene, and washed with distilled water three times. The separated organic layer was dried over magnesium sulfate, and the solvent was removed by reduced pressure rotary evaporation. This solution was finally vacuum-distilled, and a dark brown viscous liquid as 8 was obtained. 1H NMR (CDCl3): 7.3–6.6 (m, aromatic H), 4.20 (d, 4H) 4.17–3.85 (m, 4H), 4.09 (m, 1H), 3.58 (s, 1H), 3.34 (m, 2H), and 2.91–2.75 (m, 4H). 31P NMR (DMSO-d6): 9.09 (d, 2P) and 8.98 ppm (s, 1P).
Curing procedure of cyclolinear phosphazene-based epoxy resin
Both the synthesized cyclolinear phosphazene-based epoxy resin and DGEBA as a control sample were cured using MeTHPA, novolak, and DDM as hardeners. The epoxy resins were dissolved in appropriate amount of acetone, and then the hardener was added with an equivalent ratio to the epoxy resin of 1
:
1. 2-Methylimidazole (0.2 wt%) as a curing accelerator was also added into this solution. The mixture was stirred constantly to be a homogeneous solution and then was kept in a vacuum oven at 90 °C for 3 h to remove the solvent. A two-step curing procedure was carried out in a mold to obtain the thermosetting resins. The epoxy formulations containing MeTHPA, novolak, and DDM were first precured at 125, 150, and 150 °C, respectively, for 2 h, and then were further postcured at 175 for 3 h. At the end of the curing procedure, the cured system was cooled gradually to room temperature to avoid stress cracking.
Characterization and measurement
1H and 31P NMR spectra of synthesized cyclic phosphazene precursors and final product were obtained using a Bruker AV-400 400 MHz spectrometer in dimethyl sulfoxide-d6 (DMSO-d6) or deuterochloroform (CDCl3) solution, and were referenced to external tetramethylsilane (TMS) and external 85% H3PO4 with positive shifts recorded downfield from the reference, respectively. The 31P NMR spectrum was proton decoupled. Fourier transform infrared (FTIR) spectra were obtained using a Bruker Tensor–27 FTIR spectrometer with the scanning number of 50. Elemental analysis was performed with a Vario–EL–cube CHNS elemental analyzer (Elementar Analysensysteme GmbH).
The EEW of synthesized cyclolinear phosphazene-based epoxy resin was determined by the HCl/acetone chemical titration method. The molecular weight and its distribution were obtained through steric exclusion chromatography (SEC) using a Waters GPC515-2410 gel permeation chromatographer with THF as solvent at a flow rate of 1.0 mL min−1. The chromatographic column was calibrated with polystyrene standards.
Thermal curing study of the synthesized epoxy resin with various hardeners was carried out on a TA Instruments Q20 differential scanning calorimeter (DSC) equipped with a thermal analysis data station, operating at a heating rate of 10, 15, and 20 °C min−1 under a nitrogen atmosphere. The glass transition temperature was also determined by DSC. Thermogravimetric analysis (TGA) experiments were performed under a nitrogen atmosphere using a TA Instruments Q50 thermal gravimetric analyzer. The samples with a mass of about 10 mg were placed in an aluminium crucible, and ramped from room temperature up to about 800 °C at a heating rate of 10 °C min−1, while the flow of nitrogen was maintained at 50 mL min−1. The TGA analysis data were obtained from the average values of the data collected through triplicate measurements.
The limiting oxygen index (LOI) test was performed using a HD-2 oxygen index apparatus with a magneto-dynamic oxygen analyzer, according to the ASTM D-2863 standard. The mixture of oxygen and nitrogen gas was continuously sent through the combustion chamber at a flow rate of 170 mL min−1. The sample bar with a dimension of 65 × 3.0 × 1.6 mm was clamped vertically in the holder in the center of the combustion column. The top of the sample bar was ignited using a butane gas burner so that the sample bar was well lit and the entire top was burning. The relative flammability of the sample bar was determined by measuring the minimum concentration of oxygen, which would just support flaming combustion of the sample bar. The UL-94 vertical burning test was carried out based on the testing method proposed by Underwriter Laboratory according to ASTM D1356–2005 standard. Five test sample bars with a dimension of 127 × 12.7 × 1.6 mm suspended vertically over surgical cotton were ignited using a butane gas burner. The end of the sample bar was ignited twice, and each ignition was carried out for 10 s. The classification of V-0 is obtained if the burning time of each sample bar after 10 s ignition does not exceed 10 s, and the total burning time for five samples does not exceed 50 s; at the same time, the surgical cotton below the specimen cannot be ignited by flaming drippings. Scanning electron microscopy (SEM) observation was performed on a Hitachi S-4700 scanning electron microscope to investigate the morphologies of the residual chars. The char samples for SEM were obtained after combustion in the vertical burning tests, and were made electrically conductive by sputter coating with a thin layer of gold-palladium alloy. The images were taken in high vacuum mode with 20 kV acceleration voltage and a medium spot size. The residual chars were also investigated by means of FTIR spectroscopy, and the spectra were obtained at 1 cm−1 resolution in the 400–4500 cm−1 region.
Results and discussion
Synthesis and characterization
The overall reaction sequences for the synthesis of cyclolinear phosphazene-based epoxy resin are outlined in Fig. 1. 3 was firstly synthesized through the reaction of 1 with 2 to form a tetra-substituted cyclotriphosphazene. This reaction was monitored by 31P NMR spectroscopy. The 31P NMR spectrum presents an A2B spin pattern with chemical shifts of 4.72 ppm for the biphenoxy-substituted phosphorus atom, and the intensive resonance signals at 19.72 ppm are attributed to the other two phosphorus atoms bearing a phenoxy group and a chloride atom. These two different environmental phosphorus atoms, i.e. P–(OC6H5)2 and P–(OC6H5)Cl, give an integration ratio of 1
:
2. The elemental analysis results are also in good agreement with the data calculated in terms of the expected formula of 3. These data indicated that four phenoxy groups were successfully substituted on this cyclotriphosphazene monomer, and no side products were detected.
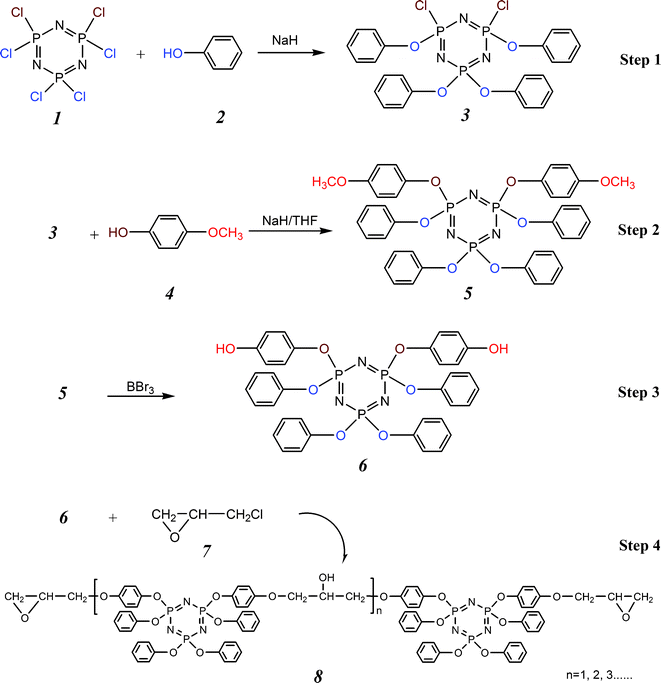 |
| Fig. 1 General synthetic route for cyclolinear phosphazene-based epoxy resin. | |
A cyclic phosphazene precursor, 6 was synthesized from 3 and 4 followed by the deblocking of methoxy groups of 5 with the catalysis of BBr3. The structure of 5 was confirmed by 1H and 31P NMR spectroscopy. The 1H NMR spectrum shows an intensive resonance signal at 3.71 ppm, which is assigned to six methoxy protons with disappearance of phenolic hydroxy protons, as well as four sets of resonance frequencies at 7.3–6.6 ppm corresponding to twenty eight aromatic protons, indicating the two chloride atoms remained on the tetra-substituted cyclotriphosphazene monomer have been successfully substituted by 4-methoxy phenoxy groups. The 31P NMR spectrum of 5 clearly displays two intensive resonance signals at 9.50 and 9.38 ppm, corresponding to two different environmental phosphorus atoms [P–(OC6H5)(OC6H5OCH3) and P–(OC6H5)2] on the phosphazene ring. It is notable that the resonance signal for the phosphorus atom geminally substituted with phenoxy groups is shifted downfield from ones with phenoxy and 4-methoxy phenoxy groups. The element analysis was also used to confirm the various element ratios in 5. The 1H NMR spectrum of 6 shows a single chemical shift at 9.42 ppm corresponding to the phenolic hydroxy protons. The other four sets of resonance signals are attributed to 28 aromatic protons. The 31P NMR spectrum contains two intensive resonance signals appearing at 8.97 and 8.87 ppm, assigned to the phosphorus atoms substituted with (OC6H5)(OC6H5OH) and (OC6H5)2 groups, respectively. Due to the similar substituent environments between OC6H5 and OC6H5OH groups, the chemical shifts for the two types of phosphorus atoms only demonstrates a slight gap of 0.1 ppm. These results can be considered as an indication of the reduction reaction from 4-methoxy phenoxy group to phenolic hydroxy group. Moreover, the elemental analysis data were consistent with the calculated one based on the formula of 6. This further confirmed the predicted structure of 6.
8 was synthesized via the polycondensation of 6 with 7. The characterization was performed by 1H and 31P NMR spectroscopy, FTIR spectroscopy, and SEC. The 1H NMR spectrum presents several sets of complicated resonance signals, which are attributed to all the protons on the molecular chains of 8 as shown in Fig. 2. As is also seen in Fig. 2, two sets of multiplet resonance signals at around 4.17–3.85 ppm corresponding to the protons (labeled c) of the methylene connected with oxirane ring are indicative of reaction at this site to form the final epoxy resin. The other two sets of multiplet resonance signals at around 2.91–2.75 ppm are attributed to the protons (labeled a) of the methylene on oxirane ring while a set of multiplet resonance signals are observed at around 3.34 ppm as the assignment for the proton (labeled b) of methine on oxirane ring. However, only very weak resonance signals at 4.20, 4.09, and 3.58 ppm, assigned to the protons of methylene [–CH2*–CH(OH)–CH2*–], methine [–CH2–CH*(OH)–CH2–], and hydroxy [–CH2–CH(OH*)–CH2–] on the molecular backbone, respectively, are observed for the synthesized epoxy resin, which contains very small amount of [–CH2–CH(OH)–CH2–] moieties. Some small signals below 2.0 ppm appeared at the 1H NMR spectrum due to a small amount of catalyst and solvents remaining in the synthesized epoxy resin. The 31P NMR spectrum illustrated in Fig. 3 also supports the chemical structure of 8, and the chemical shifts for the substituent phosphorus atoms are in good agreement with those found for 6.
FTIR spectrum of 8 shows a distinct absorption peak at 1265 cm−1 due to P
N stretching (see Fig. 4), which indicates the presence of the phosphazene rings. The characteristic peaks at 1174 and 880 cm−1 is attributed to the P–O–C bonding while the asymmetrical stretching C–O band appears at 1026 cm−1. As an important feature of the spectrum, the absorption bands at 3360 and 2920 cm−1 are attributed to the hydrogen-bonded O–H and –CH2– stretching, respectively, and an intensive absorption peak at 951 cm−1 corresponds to C–O–C stretching vibration. This provides the evidence for the introduction of epoxy groups. In addition, the characteristic absorption peaks for the aromatic C–H are also found at 1591, 1504, 765, and 688 cm−1. The GPC data reveals that 8 achieves a number-average molecular weight no more than 892, as well as a weight-average molecular weight of 974 with a polydispersity of 1.092. The GPC trace also indicates that the most (85.2 wt%) of 8 only contains one cyclotriphosphazene unit, and a little (9.4 wt%) contains two, but a very small amount contains three or more units. The EEW of 8 was determined by the HCl/acetone chemical titration method, and its result was 509.21 g/equiv. It is noteworthy that the EEW of 8 is very close to its theoretical value of 487.0 g/equiv, equal to a half of the weight-average molecular weight based on the GPC measurement, indicating that the reaction between 6 and 7 proceeded completely.
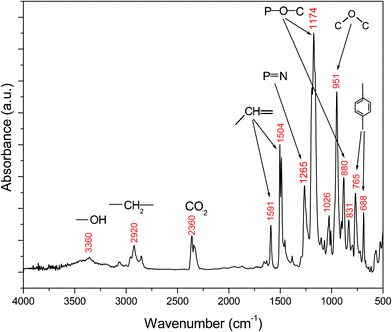 |
| Fig. 4 FTIR spectrum of cyclolinear phosphazene-based epoxy resin. | |
Curing behaviors
The thermal curing behaviors of the cyclolinear phosphazene-based epoxy resin with three hardeners (i.e. MeTHPA, novolak, and DDM) were investigated by DSC at a heating rate of 10 °C min−1, and their DSC thermograms were represented in Fig. 5. All the curing systems display a single exothermic peak corresponding to the curing reactions of the synthesized epoxy resin, which implies that the thermal curing has performed completely for each curing system without any postcuring as a result of homopolymerization. However, as shown by Fig. 5, the thermal curing reaction of this epoxy resin with novolak occurs initially at a lower temperature than the other two curing systems and shows the lowest exothermic temperature at peak as well. This indicates that novolak has much higher chemical reactivity with the synthesized epoxy resin than the other two hardeners, however, the other two curing systems have close curing temperatures, indicating that the difference of chemical reactivity towards the synthesized epoxy resin is not very obvious. It is well known that the epoxy curing is carried out through the nucleophilic substitution reaction, but its activation of the oxirane-ring opening is achieved by proton donors such as the generated hydroxyl groups during the course of reaction. Fig. 6 shows the schematic curing reactions of the cyclolinear phosphazene-based epoxy resin with these three hardeners. Novolak contains a few proton donors like hydroxyl groups within the molecule, and can catalyze the curing reaction by itself, which resulted in a higher reactivity than the other two hardeners. It is notable that MeTHPA is more reactive toward the synthesized epoxy resin than DDM owing to its higher exothermic temperature at peak. DDM as an aromatic amine has a great steric hindrance, and benzene ring group also decreases the basicity of amine, which causes a less propensity towards nucleophilic attack in oxirane ring than the other two hardeners. In addition, it is interesting to note that, compared with the other two systems, the curing system with DDM shows a narrow exothermic peak on the DSC trace, which indicates that its curing reaction proceeds faster than those of the other two in the initial stage of the curing reaction. It is understandable that the reaction rate of the nucleophilic substitution reaction is determined by the electron density in the reaction site. Owing to a direct connection with amine groups, the aromatic unit in DDM has more electron withdrawing character than the other two hardeners, thus enhancing the nucleophilicity of amine groups. Therefore, DDM reacts faster with the oxirane ring than MeTHPA and novolak.
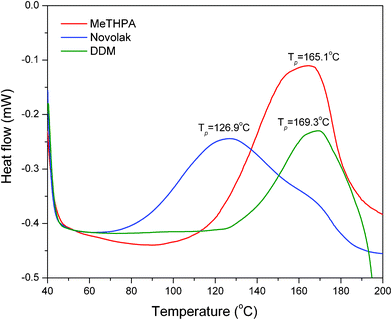 |
| Fig. 5 DSC thermograms of the curing reactions of cyclolinear phosphazene-based epoxy resin with three hardeners. | |
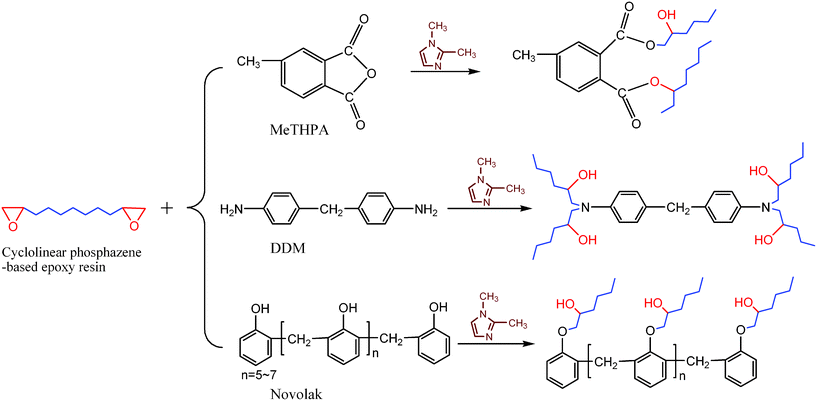 |
| Fig. 6 Scheme of the curing reactions of cyclolinear phosphazene-based epoxy resin with three hardeners. | |
Thermal behaviors and stabilities
Thermal resistance is one of the most important properties of epoxy thermosets because it establishes the service environment for the epoxy-based functional materials. Usually, the glass transition temperature (Tg) represents the limit working temperature of epoxy thermosets, which are only used well, in most cases, at a temperature below Tg. Therefore, it is very important for the epoxy resin to achieve a high Tg when designing its molecular structure. The Tg of the cyclolinear phosphazene-based epoxy thermosets were evaluated by DSC. Fig. 7 shows their DSC thermograms, and the results are listed in Table 1. It is noteworthy that all the thermosets display a high Tg more than 150 °C as expected in comparison with the conventional epoxy thermosets reported in the literature.33,34 It is known that glass transition is generally ascribed to the segmental motion of the polymeric networks, and the Tg is determined by the degree of freedom for the segmental motion, crosslinking and entanglement constraints, and the packing density of the segments.35 In this work, phosphazene rings were incorporated into the backbone chain of the epoxy resin as well as the presence of aromatic substituents on the phosphazene ring, leading to a great steric hindrance, and thus Tg of the cyclolinear phosphazene-based epoxy thermosets increased as a result of the confinement to the segmental motion. It is also observed that the epoxy thermosets achieves a higher Tg when cured with novolak compared to the other two hardeners. It is well known that novolak has more reactive groups for crosslinking than MeTHPA and DDM. This results in a high packing density for the networks, and therefore, the Tg is improved. Nevertheless, the thermoset cured with DDM gives a higher Tg than that with MeTHPA. It is reasonable to believe that the rotational hindrance of the phenyl groups on the DDM molecules restricts the segmental motion, and thus increases the Tg of the epoxy thermoset.
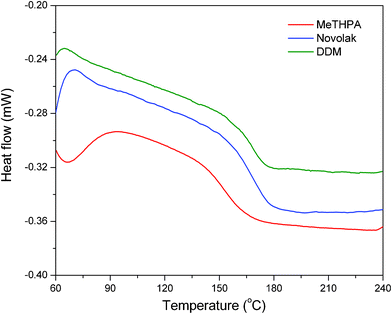 |
| Fig. 7 DSC thermograms of cyclolinear phosphazene-based epoxy thermosets cured with three hardeners. | |
Table 1 The thermal analysis data obtained from DSC and TGA measurements for the cyclolinear phosphazene-based epoxy thermosets curing with three hardeners
Thermoset sample |
T
g (°C) |
Nitrogen atmosphere |
Air atmosphere |
T
onset
b (°C) |
T
max1
c (°C) |
T
max2
c (°C) |
Char yield at 750 °C (wt%) |
T
onset
b (°C) |
T
max1
c (°C) |
T
max2
c (°C) |
Char yield at 750 °C (wt%) |
Abbreviation of the cyclolinear phosphazene-based epoxy.
The onset decomposition temperature, at which the thermoset undergoes 3 wt% weight loss.
The characteristic temperature, at which maximum rate of weight loss occurs.
|
CL-PN epoxya/MeTHPA |
156.8 |
385.7 |
448.5 |
— |
42.19 |
390.9 |
451.7 |
669.3 |
57.64 |
CL-PN epoxya/novolak |
170.4 |
435.9 |
456.1 |
540.6 |
48.32 |
434.2 |
458.3 |
542.5 |
54.18 |
CL-PN epoxya/DDM |
166.1 |
404.1 |
490.2 |
507.4 |
42.85 |
410.9 |
526.4 |
623.6 |
56.73 |
The thermal stabilities of the cyclolinear phosphazene-based epoxy thermosets cured with three hardeners were investigated by TGA under both air and nitrogen atmospheres. Typical TGA thermograms of these thermosets are presented in Fig. 8, and the analysis data are summarized in Table 1. All the TGA traces exhibit a typical two-stage degradation as a result of the fact that the thermosets undergoes two-stage decompositions for the different components of the thermoset molecules. The first stage of thermal degradation is caused by the major fragmentation of the polymeric networks, and the second one is attributed to the decomposition of cyclotriphosphazene moieties due to its higher thermal stability. The TGA results demonstrate that the onset decomposition temperatures of all the thermosets corresponding to 3 wt% weight loss is around 380–440 °C under both air and nitrogen atmospheres, with maximum thermooxidative decomposition occurring mainly beyond 450 °C, at which the weight loss occurs at a maximum rate. These thermosets also achieved very high char yields more than 40 wt% in both air and nitrogen. These results indicate that the cyclolinear phosphazene-based epoxy resin synthesized in this work has a very good thermal stability. It is very surprising to note that all the thermosets show higher decomposition temperatures at the maximum rate in air than those in nitrogen, indicating that the thermooxidative decomposition is restrained in a rich oxygen atmosphere. This interesting feature may be ascribed to the fact that the oxygen in air can promote the formation of a phosphorocarbonaceous solid phase, which resists the oxidation taking place inside the thermosets. Furthermore, it is noteworthy that the thermosets show much higher char yields in air than those in nitrogen. The thermoset cured with MeTHPA shows the highest char yield of 56.64 wt% at 750 °C in air among these tree samples. Similar high char yield was obtained for the thermosets cured with novolak and DDM. The achievement of such high char yields in air may be explained due to the formation of compact char layer as a result of the reaction of phosphazene rings in the thermosets and the oxygen in air, which prevents the thermooxidative decomposition. In addition, the thermoset cured with novolak shows the highest char yield in nitrogen among these three thermosets, which indicates that the high crosslinking density of the thermoset dominates the formation of char in the absence of oxygen. From these results, it is apparent that the cyclolinear phosphazene-based epoxy resin is superior in fire and heat resistance compared to other known phosphorus-containing epoxy resins that have been reported.2,3,18,19
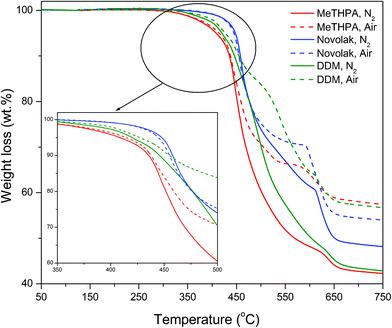 |
| Fig. 8 TGA thermograms of cyclolinear phosphazene-based epoxy thermosets cured with three hardeners. | |
Flammability characteristics
Flammability characteristics of the cyclolinear phosphazene-based epoxy thermosets cured with three hardeners have been evaluated by LOI measurements and vertical burning tests, the results are summarized in Table 2 as well as the test results of a conventional epoxy (DGEBA) thermoset as a control sample. As is well known, LOI measures the minimum oxygen concentration of flowing gas mixed by oxygen and nitrogen required supporting downward flame combustion, which can be used as an indicator to evaluate the flame retardancy of the thermosets. Since DGEBA did not contain any efficient flame retardant components, its thermosets only achieved very low LOI values of around 20–22 vol.%. It is expected that all the cyclolinear phosphazene-based epoxy thermosets exhibit high LOI values far beyond 29 vol.%, equal to the oxygen concentration of air. This means these thermosets should be completely non-flammable in air, although the LOI values vary a little when the thermosets were cured with different hardeners. This indicates that incorporating phosphazene rings into the backbones of epoxy resin is very effective in improving its flame retardancy, and thus makes the epoxy resin become a non-flammable functional polymer. The UL-94 vertical burning test determines the upward burning characteristics of the epoxy thermosets. As shown in Table 2, all the cyclolinear phosphazene-based epoxy thermosets achieved a UL94 V-0 classification in UL-94 test, and most of the cyclolinear phosphazene-based epoxy thermosets quenched with 1 or 2 s when flame agitator was removed during the tests, exhibiting an auto-extinguishable feature. Additionally, the burned residues of these thermosets did not fall off during vertical burning, indicating a good structural stability. However, all the DGEBA thermosets failed in UL-94 test. They seemed to be highly combustive, and almost every test bar had continuously burned for more than 50 s with serious flaming drips after the removal of fire during the vertical burning test. In order to visibly understand the difference of flammability characteristics between the cyclolinear phosphazene-based epoxy and DGEBA, the burning behaviors of the two types of epoxy thermosets have been recorded by a digital camera at different stages of vertical burning experiments and are presented in Fig. 9. It is clearly observed from these photographs that the DGEBA thermoset burned aggressively in application to a fire while the cyclolinear phosphazene-based epoxy one only exhibited a mild combustion. After application of a flame for 10 s, the DGEBA thermoset still burned for a long time with serious flaming drips, indicating a highly flammable property. However, in the case of the cyclolinear phosphazene-based epoxy thermoset, one of the fascinating characteristics of combustion is that it just combusted slightly with a small blaze and was extinguished quickly by itself within 5 s. At the end of burning experiment, the surface of the thermoset was covered with an expanded char network, indicating that this thermoset formed an effective char which was able to prevent the heat transfer and flame spread during combustion. These results give an evidence of non-flammability for the cyclolinear cyclotriphosphazene-linked epoxy thermosets.
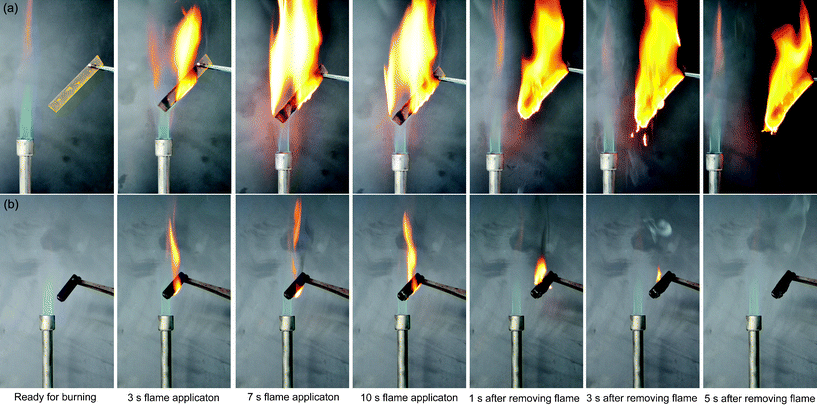 |
| Fig. 9 Photographs of the burning behaviors of (a) DGEBA and (b) cyclolinear cyclotriphosphazene-linked epoxy thermosets during a vertical burning experiment. | |
Table 2 The flammability characteristics of the thermosets of cyclolinear phosphazene-based epoxy and DGEBA cured with three hardeners
Thermoset sample |
LOI value (vol.%) |
Flammability from vertical burning testing |
UL-94 classification |
Flaming drips |
Total flaming time (sec) |
Maximal flaming time (sec) |
Abbreviation of the cyclolinear phosphazene-based epoxy.
|
CL-PN epoxya/MeTHPA |
36.5 |
V-0 |
None |
6.9 |
1.6 |
DGEBA/MeTHPA |
20.4 |
Failed |
Yes |
> 250 |
> 50 |
CL-PN epoxya/novolak |
38.7 |
V-0 |
None |
5.5 |
1.2 |
DGEBA/novolak |
21.97 |
Failed |
Yes |
> 250 |
> 50 |
CL-PN epoxya/DDM |
39.2 |
V-0 |
None |
3.7 |
0.9 |
DGEBA/DDM |
22.23 |
Failed |
Yes |
> 250 |
> 50 |
It is well known that the non-flammability of the cyclotriphosphazene moieties can be conferred to the resulting polymers, especially of low molecular weights. Gouri et al. reported the synthesis and flame retardancy of hexaglycidyl cyclotriphosphazene (HGCP) as a reactive flame retardant, and found that incorporation of 20 wt% HGCP into commercial DGEBA epoxy resin makes the thermoset gain a UL94 V-0 classification.28 Although this cyclotriphosphazene-containing thermoset has a same non-flammable property with the cyclolinear phosphazene-based epoxy thermosets in our work, its thermal stability is much lower. Moreover, the thermosets derived from the epoxy resin synthesized in this study also achieved much higher LOI values than that from the cyclotriphosphazene-containing epoxy resin reported by Chen-Yang et al. as well as our previously study.2,31 On the basis of these results, it is clear that the cyclolinear phosphazene-based epoxy has a virtually incombustible nature. Such high flame retardancy is attributed to the presence of unique combination of phosphorus and nitrogen in the thermosets as a result of embedding phosphazene rings on the backbone chain of epoxy resin in a cyclolinear mode. This characteristic molecular structure is highly advantageous to the reactive flame retardancy induced by the synergistic effect of phosphorus and nitrogen.
Analysis of residual char
In order to learn more about the flame-retardant mechanism of the cyclolinear phosphazene-based epoxy resin, the morphologies of the residual chars obtained from UL-94 vertical burning tests were investigated by SEM. Fig. 10 illustrates the SEM images of these residual chars. It is notable that the outline of a residual char exhibits some irregular-shaped bulk, and the inside of the chars shows a multiporous structure, indicating a typical morphology after the intumescent char formation. However, as is shown by the SEM images of each sample, all the residual chars illustrate a very gassy surface without any pores breaking through. It is well known that the physical structure of the charring layer plays a very important role in the performance of flame retardancy. From the observation of the residual chars of the cyclolinear phosphazene-based epoxy thermosets, the char layer formed during combustion is rigid and compact in nature, and there are lots of integrated closed honeycomb pores inside. Such a structural form favors the temperature grades in the char layer and protects the matrix inside. It is evident that the thermooxidative reaction of cyclotriphosphazene moieties enhances the char formation during combustion, which results in a protective char layer formed on the surface of thermosets serving as a barrier against heat and oxygen diffusion, and consequently the flame retardancy of the thermosets is improved significantly.
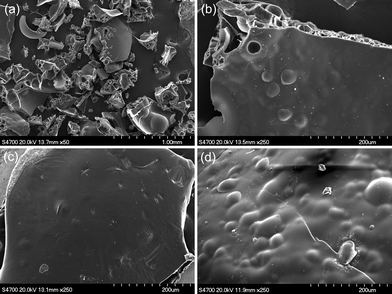 |
| Fig. 10 SEM images of the residual chars obtained from the vertical burning tests for cyclolinear phosphazene-based epoxy thermosets cured with three hardeners; (a) the outline of a residual char, (b) thermoset cured with MeTHPA, (c) thermoset cured with novolak, and (d) thermoset cured with DDM. | |
The chemical structures of residual chars were also studied by FTIR spectroscopy. Fig. 11 shows the FTIR spectra of the residual chars collected from three thermosets after UL-94 vertical burning test. It is visible that the three spectra present the similar patterns of highly carbonized compounds, indicating these residual chars have a similar chemical structure. In these spectra, a weak and broad peak at 3500–3000 cm−1 is assigned to the stretching vibration of OH bond. Two intensive absorption peaks appear at 1027 and 945 cm−1 due to P–O–C bond while the characteristic peak for P
N stretching vibration almost disappears at 1261 cm−1. Furthermore, a series of absorption peaks at 1591, 1497, 843, and 768 cm−1 can be attributed to the carbonized networks like aromatics and polyaromatics formed during the combustion. These results indicate that these residual chars consist of cross-linked phosphorocarbonaceous and phosphorooxidative solids as well as highly carbonized aromatic networks.
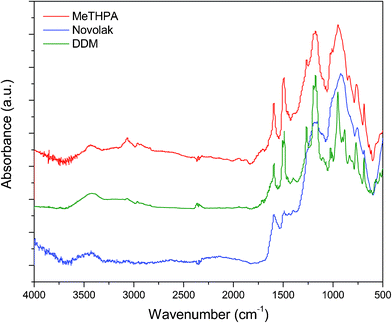 |
| Fig. 11 FTIR spectra of the residual chars obtained from the vertical burning tests for cyclolinear phosphazene-based epoxy thermosets cured with three hardeners. | |
Based on the physical and chemical structure investigation of the residual chars, it is concluded that the non-flammability of the cyclolinear phosphazene-based epoxy resin results from the inherent flame retardancy of cyclotriphosphazene moieties due to its synergistic effect of the phosphorus nitrogen combination. Although the flame-retardant mechanism of phosphazene-based polymers like the epoxy resin synthesized in this work is still not very clear, it is deduced that the presence of cyclotriphosphazene moieties on the backbone of the epoxy resin enhances the flame retardancy in the ways of both condensed and gaseous phases.36–40 The thermooxidative reaction of phosphazene rings with the other segments can form phosphorus-rich char on the surface as a barrier to inhibit gaseous products from diffusing to the flame and to shield the polymer surface from heat and air during combustion. Simultaneously, the pyrolysis of phosphazene rings can produce phosphoric or polyphosphoric acid, which acts in the condensed phase promoting char formation. Meanwhile, the cyclotriphosphazene moieties can release non-flammable gases such as CO2, NH3 and N2 during combustion to dilute the hot atmosphere and cool the pyrolysis zone at the combustion surface.28,41 These mentioned inflammable gases can cut off the supply of oxygen and can play a very important role in the auto-extinguishing behaviour of the thermosets. Nevertheless, the effect of the molecular structures of phosphazene-based epoxy polymers and their participation in all stages of combustion process are not fully understood. A further intensive study is still necessary to clarify their effecting mechanisms, so that the optimal molecular structure of the phosphazene-based epoxy resins can be designed for flame retardancy and high performance.
Conclusion
A novel cyclolinear structured flame-retardant epoxy resin based on phenol-substituted cyclotriphosphazene was synthesized through a four step reaction, and the chemical structure of all the intermediate and final products were confirmed by 1H and 31P NMR spectroscopy, elemental analysis, and FTIR spectroscopy. The thermosets prepared by this cyclolinear phosphazene-based epoxy resin with MeTHPA, novolak, and DDM as hardeners achieved a high thermal resistance due to their high Tg over 150 °C, and they also gained good thermal stabilities with high char yields. The incorporation of phosphazene rings into the backbone chain imparts non-flammability to this epoxy resin due to the unique combination of phosphorus and nitrogen followed by a synergistic effect on flame retardancy. This resulted in the high LOI values and UL-94 V-0 classification of this epoxy resin when prepared for thermosets. The epoxy resin obtained in this work will become a potential candidate as a non-halogen functional material for fire- and heat-resistant applications in electronic and microelectronic fields with better safety and excellent performance.
Acknowledgements
The authors greatly appreciate the financial support from the National Natural Science Foundation of China (Grant No. 50973005).
References
- W. Liu, Q. Qiu, J. Wang, Z. Huo and H. Sun, Polymer, 2008, 49, 4399–4405 CrossRef CAS.
- Y. W. Chen-Yang, H. F. Lee and C. Y. Yuan, J. Polym. Sci., Part A: Polym. Chem., 2000, 38, 972–981 CrossRef CAS.
- X. Wang and Q. Zhang, Eur. Polym. J., 2004, 40, 385–395 CrossRef CAS.
- S. Lu and I. Hamerton, Prog. Polym. Sci., 2002, 27, 1661–1712 CrossRef CAS.
- M. A. Uddin, T. Bhaskar, T. Kusaba, K. Hamano, A. Muto and Y. Sakata, Green Chem., 2003, 5, 260–263 RSC.
- A. Cyriac, S. H. Lee, J. K. Varghese, J. H. Park, J. Y. Jeon, S. J. Kim and B. Y. Lee, Green Chem., 2011, 13, 3469–475 RSC.
- Y. L. Lin, G. H. Hsiue, Y. S. Chiu and R. H. Lee, J. Appl. Polym. Sci., 1997, 63, 895–901 CrossRef.
- M. Pecht and Y. Deng, Microelectron. Reliab., 2006, 46, 53–62 CrossRef CAS.
- S. Zhang, H. Lefebvre, M. Tessier and A. Fradet, Green Chem., 2011, 13, 2786–2793 RSC.
-
Fire Retardancy of Polymers, ed. M. Le Bras, C. A. Wilkie and S. Bourbigot, The Royal Society of Chemistry, 2005, pp. 126–138 Search PubMed.
- G. Wang, W. Cheng, Y. Tu, C. Wang and C. Chen, Polym. Degrad. Stab., 2006, 91, 3344–3353 CrossRef CAS.
- L. Becker, D. Lenoir, G. Matuschek and A. Kettrup, J. Anal. Appl. Pyrolysis, 2001, 60, 55–67 CrossRef CAS.
- H. Ren, J. Sun, B. Wu and Q. Zhou, Polym. Degrad. Stab., 2007, 92, 956–961 CrossRef CAS.
- A. Toldy, A. Szabo, C. Novak, J. Madarasz, A. Toth and G. Marosi, Polym. Degrad. Stab., 2008, 93, 2007–2013 CrossRef CAS.
- F. Gao, L. Tong and Z. Fang, Polym. Degrad. Stab., 2006, 91, 1295–1299 CrossRef CAS.
- S. Sun, Y. He, X. Wang and D. Wu, J. Appl. Polym. Sci., 2010, 118, 611–622 CAS.
- S. Lu and I. Hamerton, Prog. Polym. Sci., 2002, 27, 1661–1712 CrossRef CAS.
- J. Shieh and C. Wang, J. Polym. Sci., Part A: Polym. Chem., 2002, 40, 369–378 CrossRef CAS.
- L. Gao, D. Wang, Y. Wang, J. g Wang and B. Yang, Polym. Degrad. Stab., 2008, 93, 1308–1315 CrossRef CAS.
- B. Schartel, U. Braun, A. I. Balabanovich, J. Artner, M. Ciesielski, M. Doring, R. M. Perez, J. K. W. Sandler and V. Altstadt, Eur. Polym. J., 2008, 44, 704–715 CrossRef CAS.
- H. R. Allcock and P. E. Austin, Macromolecules, 1981, 14, 1616–1622 CrossRef CAS.
- D. Kumar, G. M. Fohlen and J. A. Parker, Macromolecules, 1983, 16, 1250–1257 CrossRef CAS.
- C. W. Allen, J. Fire Sci., 1993, 11, 320–328 CrossRef CAS.
- H. R. Allcock and M. s. Connolly, Macromolecules, 1985, 18, 1330–1340 CrossRef CAS.
- H. Lim and J. Y. Chang, J. Mater. Chem., 2010, 20, 749–754 RSC.
- S. Chen, Q. Zheng, G. Ye and G. Zheng, J. Appl. Polym. Sci., 2006, 102, 698–702 CrossRef CAS.
- J. Ding and W. Shi, Polym. Degrad. Stab., 2004, 84, 159–165 CrossRef CAS.
- M. E. Gouri, A. E. Bachiri, S. E. Hegazi, M. Rafik and A. E. Harfi, Polym. Degrad. Stab., 2009, 94, 2101–2106 CrossRef.
- D. A. Conner, D. T. Welna, Y. Chang and H. R. Allcock, Macromolecules, 2007, 40, 322–328 CrossRef CAS.
- S. Fei and H. R. Allcock, J. Power Sources, 2010, 195, 2082–2088 CrossRef CAS.
- R. Liu and X. Wang, Polym. Degrad. Stab., 2009, 94, 617–624 CrossRef CAS.
- L. Qian, L. Ye, G. Xu, J. Liu and J. Guo, Polym. Degrad. Stab., 2011, 96, 1118–1124 CrossRef CAS.
- A. Schafer, S. Seibold, O. Walter and M. Doring, Polym. Degrad. Stab., 2008, 93, 557–560 CrossRef.
- O. Mauerer, Polym. Degrad. Stab., 2005, 88, 70–73 CrossRef CAS.
- H. Wang, Y. Zhang, L. Zhu, Z. Du, B. Zhang and Y. Zhang, Thermochim. Acta, 2011, 521, 18–22 CrossRef CAS.
- S. Nie, Y. Hu, L. Song, Q. He, D. Yang and H. Chen, Polym. Adv. Technol., 2008, 19, 1077–1083 CrossRef CAS.
- F. Laoutid, L. Bonnaud, M. Alexandre, J. Lopez-Cuesta and P. Dubois, Mater. Sci. Eng., R, 2009, 63, 100–125 CrossRef.
- S. V. Levchik and E. D. Weil, J. Fire Sci., 2006, 24, 345–364 CrossRef CAS.
- H. Peng, Q. Zhou, D. Wang, L. Chen and Y. Wang, J. Ind. Eng. Chem., 2008, 14, 589–595 CrossRef CAS.
- C. Nguyen and J. Kim, Polym. Degrad. Stab., 2008, 93, 1037–1043 CrossRef CAS.
- G. F. Levchik, Y. V. Grigoriev, A. I. Balabanovich and S. V. Levchik, Polym. Int., 2000, 49, 10959–1100 Search PubMed.
Footnote |
† Electronic supplementary information (ESI) available: See DOI: 10.1039/c2ra20739g |
|
This journal is © The Royal Society of Chemistry 2012 |
Click here to see how this site uses Cookies. View our privacy policy here.