DOI:
10.1039/C2RA20447A
(Paper)
RSC Adv., 2012,
2, 6274-6289
High Tg, processable fluorinated polyimides containing benzoisoindoledione unit and evaluation of their gas transport properties
Received
30th December 2011
, Accepted 3rd May 2012
First published on 4th May 2012
Abstract
This article describes the preparation of a series of processable fluorinated poly(ether imide)s from a new diamine monomer 4,9-bis-[4-(4′-amino-3-trifluoromethyl-biphenyl-4-yloxy)-phenyl]-2-phenyl-benzo[f]-isoindole-1,3-dione. The polymers showed extremely high Tg (up to 335 °C), outstanding thermal stability (up to 559 °C) and high tensile strength (up to 102 MPa). The transparent (λcut-off ≥ 363 nm) membranes from these polymers were investigated for their gas transport properties for CO2, O2, N2 and CH4 at three different temperatures. The effect of the benzo[f]isoindole-1,3-dione moiety and the aromatic dianhydrides (BPADA, 6FDA, BTDA, ODPA and PMDA) towards the glass transition temperatures of the polymers and their consequences on gas transport properties have been investigated. The polymer prepared from 6FDA showed the highest permeability coefficient for all the gases (PCO2 = 71.3, PO2 = 25.4) whereas BPADA based polymer exhibited the highest permselectivity (CO2/CH4 ∼ 37.2 and O2/N2 ∼ 8.4), staying closer to the latest upper boundary limit drawn by L. M. Robeson.
Introduction
Polyimide exhibits a number of outstanding properties such as, excellent thermal, mechanical, and electrical properties which led to its application in several robust fields starting from engineering thermoplastic to aerospace and electronic industries as well as for fibers, adhesives and in matrixes for advanced composite materials.1 Hence, polyimides have been considered as a class of high performance polymers and used for various advanced technologies like semiconductor, electronic packaging, fuel cell, liquid crystalline display (LCD) etc.2–4 Polyimides are also endowed with high thermo-oxidative stability, chemical and solvent resistive properties.5 In addition to all these excellent physical properties polyimides exhibit high gas selectivity for different gas pairs (e.g., CO2/CH4, H2/CH4, H2/CO, O2/N2etc.) which render these polymers increasingly interesting as the most appropriate basic materials for membrane based gas separation.6–8 Accordingly, development of polyimide based gas transport film has also been considered of significant commercial interest along with another class of films i.e., super gas barrier films for food packaging applications.9–16 Polyimides have mostly been used by Du Pont Co. (USA) and Ube Industries (Japan) are in their commercial application in separation processes.17 However, high softening temperature (Tg) and poor solubility of the polyimides in different organic solvents lead to the difficulty in processing in both melt and solution conditions. On the other hand, it is generally accepted that high Tg polymers leads to higher gas permselectivity for a pair of gases.18 Hence, high Tg of the polyimides is one of the important criteria to achieve a better separation performance besides their high thermal stability and mechanical strength. Calle et al. prepared a series of high Tg polyimides and observed an increment in permselectivity compared to very similarly structured polyimides of lower Tg.19 However, polyimides with high Tg mostly suffers from poor processability, particularly, by melt processing techniques. Solution processing could be another approach in this regard, but needs proper structural modification without compromising Tg to greater extent. In recent years, much attention has been given to the preparation of solution processable polyimides by designing new diamine or dianhydride monomers.20–22 In this connection, a plethora of literature report that inclusion of cardo groups, pendant trifluoromethyl groups and flexible ether linkages into the main chain enormously enhance the organosolubility of polyimides.23 Besides this, the presence of pendent trifluoromethyl groups in the main chain leads to more gas permeability as well as permselectivity for different gas pairs.24–27 Introduction of cardo groups into the main chain has been another approach to improve the gas separation performances of the polymers.28–30
Earlier we reported the gas transport behavior through cardo phenolphthalein containing fluorinated poly(ether imide)s (PEIs) where we observed an enhancement in the gas permeability as well as ideal permselectivity (especially, for CO2/CH4 gas pair) in comparison to other phthalimidine based polymers or closely structured fluorinated poly(ether imide)s.31 It is noteworthy to mention that rigid aromatic dianhydrides 6FDA [2,2-bis(3,4-dicarboxyphenyl) hexafluoropropane] and PMDA [benzene-1,2,4,5-tetracarboxylic dianhydride] based polyimides exhibited excellent ideal separation performances closer to the latest upper boundary limit drawn by Robeson.32 Those observations prompted us to search for a series of polyimides with more rigidity to surpass the upper boundary limit drawn by Robeson. The benzoisoindoledione unit is comprised of completely aromatic content and can be considered as a highly rigid framework owing to the extended planar aromatic moiety. Moreover, this cardo group is conformationally highly rigid due to the planar aromatic moiety that prevents mutual rotation of the aryl rings owing to its bulkiness, and formation of intermolecular charge transfer complex formation between the planar aromatic moiety and the diimide moiety present in the PEI backbone. Recently, Kricheldorf and coworkers prepared a series of polyimides with bulky substituents, hindered rotation of aromatic rings and a kinked nano structure, and they found that the gas separation properties were relatively close to the upper bounds defined by Robeson.33 Kim et al. also observed a dramatic increase in oxygen permeability when they included rigid 3,3′,4,4′-biphenyltetracarboxylic dianhydride in combination with a bulky p-tert-butylphenyl group or p-trimethylsilylphenyl group.34 Based on all these observations, we envisaged that the high conformational rigidity and bulkiness of benzoisoindoledione building blocks in combination with the fluorinated moieties within a poly(ether imide) matrix may lead to high processability as well as high gas permeability due to its bulky building block and high permselectivity from its higher packing efficiency originating from the extended planar and rigid structure. The ideal separation performance for both the CO2/CH4 and O2/N2 gas pair may also be further improved. It was already established in the literature that the conversion of the lactone moiety present in phenolphthalein to its corresponding anilide by the N-phenyl group leads to better separation performance towards different gas pairs.35,36 Therefore, we decided to replace the N-phenyl substituted phthalimidine unit with a more rigid as well as polar N-phenyl substituted benzoisoindoledione unit.
In view of all these, in continuation of our investigation on new polymeric membranes for superior gas transport properties,29,31,37 this present investigation consists of a study on the synthesis and gas transport properties of a new series of fluorinated PEIs containing the benzoisoindoledione moiety in the main chain. An attempt has also been taken towards the establishment of a correlation between the structural variations in the repeat unit of the PEIs and their impact on their gas transport properties.
Experimental section
Materials
All the reagents were purchased from Fluka, Aldrich and Acros Chemical Company and used as received unless otherwise noted. Phenolphthalein (Loba Chemie Pvt, India), zinc granules, maleic anhydride, aniline, K2CO3, and dichloromethane (DCM) (E. Merck, India) were used as received. All the dianhydrides, 4,4′-(4,4′-isopropylidenediphenoxy)bis(phthalic anhydride) (BPADA), 2,2-bis(3,4-dicarboxyphenyl) hexafluoropropane (6FDA), benzophenone-3,3′,4,4′-tetracarboxylic dianhydride (BTDA), 4,4′-oxydiphthalic anhydride (ODPA) and benzene-1,2,4,5-tetracarboxylic dianhydride (PMDA) were recrystallized from acetic anhydride and heated at 120 °C overnight prior to use. DMF (E. Merck, India) was purified by stirring with NaOH and distilled twice from P2O5 under reduced pressure. Toluene (E. Merck, India) was dried by refluxing over sodium metal.
Measurements
The carbon, hydrogen and nitrogen content of the compounds were analyzed by a pyrolysis method using a Vario EL (Elementar, Germany) elemental analyzer. FTIR spectra were recorded from a NEXUS 870 FTIR (Thermo Nicolet) spectrophotometer at room temperature and humid free atmosphere; KBr pellets were used for the monomers and ATR technique was used for polymeric membranes. 1H-NMR and proton decoupled 13C-NMR of monomers and polymers were recorded on a Bruker 200 and 500 MHz instrument (Switzerland) using CDCl3 and DMSO-d6 as solvents and TMS as the reference. Differential scanning calorimetry (DSC) was carried out under nitrogen using NETZSCH DSC 200PC differential scanning calorimeter at a heating rate of 20 °C min−1. Glass transition temperatures (Tg) were taken at the center of the step transition in the second heating run. Thermal decomposition behavior of these polymers was measured on a TGA Q 5000 of TA Instruments thermogravimetric analyzer at a heating rate of 10 °C min−1 under synthetic air (N2:O2 is 4
:
1). Tensile strength and elongation at break of thin polymer membranes were tested using a Hounsfield H10KS-0547 at room temperature at 5% strain rate of the sample specimen length; five specimens were used for the measurements. Wide angle X-ray diffractograms were recorded by Rigaku, Ultema III X-ray diffractometer using a Cu-Kα (λ = 0.154 nm) source, operated at 40 kV and 40 mA. The range of 2 theta for XRD measurements was 5–50 degrees. Inherent viscosities (ηinh) of all these polymer solutions in N,N-dimethylacetamide (DMAc) were determined at about 0.5 g/dL concentration using an Ubbelohde viscometer at 30 ± 0.5 °C. Gel permeation chromatography was performed with a Waters GPC instrument (Waters 2414). Tetrahydrofuran (THF) was used as the eluent (flow rate 0.5 mL min−1, polystyrene was used as a standard and a RI detector was used to record the signal. The densities of the membranes were determined using a Wallace High Precision Densimeter-X22B (U.K) by the water displacement method.
Monomer synthesis
4,9-Bis-(4-hydroxy-phenyl)-2-phenyl-benzo[f]isoindole-1,3-dione (6).
The bis(ether amine) monomer (8) was synthesized starting from phenolphthalein, as presented in Scheme 1. In a round bottom flask equipped with a condenser and a magnetic stirrer, phenolphthalein (1) (10.0 g, 0.03 mole), zinc granules (27.8 g, 0.43 mole) and sodium hydroxide (34 g, 0.85 mole) were taken into a solvent mixture of ethanol and water (1
:
1, v/v, 150 mL). The mixture was left to stir under reflux conditions. After seven hours of reflux the initial dark color turned into light pink. The reaction was monitored by thin layer chromatography (TLC) and it took ten hours to complete the reaction while the reaction mixture became yellowish in color. After completion of the reaction unreacted zinc was removed from the mixture by filtration. The solution was cooled and acidified to pH 6 by drop wise addition of concentrated hydrochloric acid. The off white precipitate was filtered, washed with water and dried at 80 °C. Yield: 9.4 g (93%). Mp: 242 °C. 1H-NMR (DMSO-d6, 200 MHz, δ (ppm)): 9.19 (s, 2H, H8), 7.69 (d, J = 7.6 Hz, 1H, H1), 7.38 (d, J = 7.6 Hz, 1H, H3), 7.27 (d, J = 7.4 Hz, 1H, H2), 6.95 (d, J = 7.8 Hz, 1H, H4), 6.75 (d, J = 8.4 Hz, 4H, H5), 6.63 (d, J = 8.0 Hz, 4H, H6), 6.36 (s, 1H, H7).
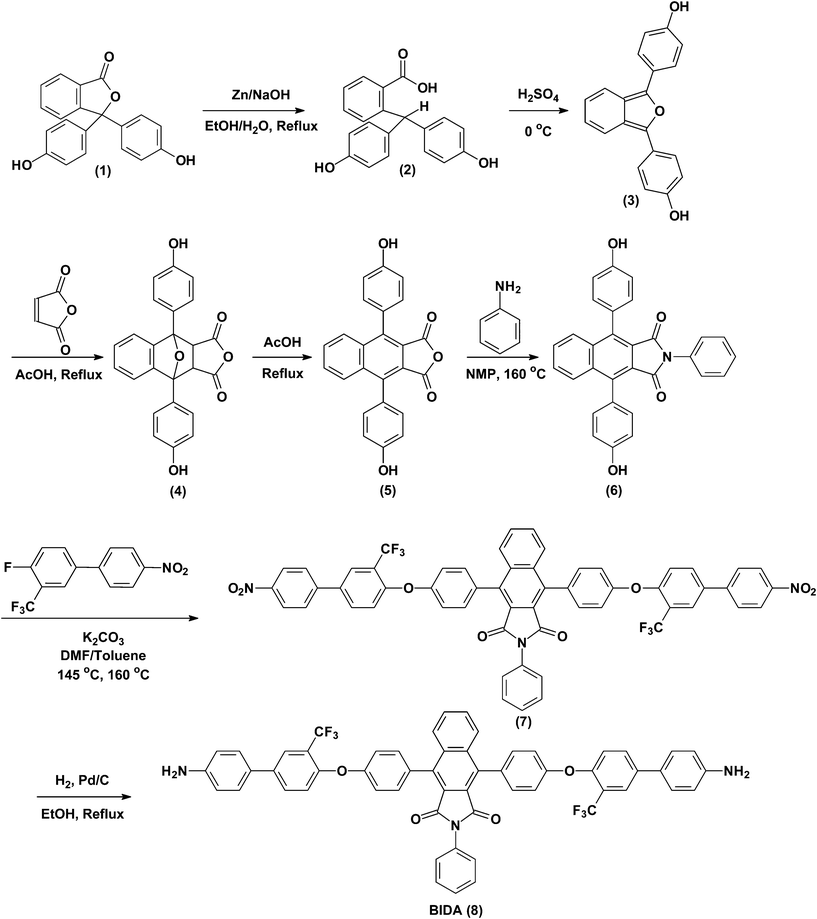 |
| Scheme 1 Synthesis of the bis(ether amine) monomer, ‘8’. | |
The remaining reaction steps for the preparation of the bis-hydroxy compound, 4,9-bis-(4-hydroxy-phenyl)-2-phenyl-benzo[f]isoindole-1,3-dione (6) from phenolphthalin (2) were done by following the reaction protocol as described by Hay and coworkers.38 The bis-hydroxy compound (6) was characterized by FT-IR, 1H-NMR, 13C-NMR spectroscopy which strongly supports the chemical structure of the bis-hydroxy compound (6).
4,9-Bis-[4-(4′-nitro-3-trifluoromethyl-biphenyl-4-yloxy)-phenyl]-2-phenyl-benzo[f]isoindole-1,3-dione (7).
In a round bottom flask equipped with a Dean–Stark apparatus, a nitrogen inlet and a magnetic stirrer 4,9-bis-(4-hydroxy-phenyl)-2-phenyl-benzo[f]isoindole-1,3-dione (6) (11.0 g, 24.05 mmole), 4-fluoro-4′-nitro-3-trifluoromethyl-biphenyl (13.72 g, 48.09 mmole) and anhydrous potassium carbonate (K2CO3) (9.97 g, 17.5 mmole) were dissolved into a solvent mixture of dry NMP and toluene (1
:
1, v/v, 160 mL). The mixture was initially heated for four hours at 145 °C, followed by heating at 160 °C for two hours. After completion of the reaction, monitored by thin layer chromatography (TLC), the reaction mixture was precipitated in a large excess of water to remove NMP. The precipitate was filtered, washed with water and dried at 80 °C. Yield: 23.2 g (98%). Mp: 407 °C. Anal.Calcd. for C56H31F6N3O8 (987.85 g mol−1): C, 68.09%; H, 3.16%; N, 4.25%; Found: C, 67.93%; H, 3.02%; N, 4.42%. FTIR (KBr, cm−1): 3072 (aromatic C–H stretching), 1766 (asymmetric imide C
O stretching), 1485, 1341 (asymmetric C–O–C stretch), 1249, 1133 (band due to C–F absorption), 1054 (symmetric C–O–C stretch), 851 (C–N stretch for aromatic—NO2), 824 (aromatic C–H bend out of plane). 1H–NMR (CDCl3, 400 MHz, δ (ppm)): 8.33 (d, J = 8.8 Hz, 4H, H2), 7.96–7.92 (m, 4H, H18, H23), 7.76 (d, J = 2.4 Hz, 2H, H11), 7.74 (d, J = 8.8 Hz, 4H, H3), 7.70–7.67 (m, 2H, H19), 7.54 (d, J = 8.4 Hz, 4H, H14), 7.47–7.34 (m, 5H, H6, H24, H25), 7.31 (d, J = 8.4 Hz, 4H, H13), 7.27 (d, J = 8.4 Hz, 2H, H7). 13C–NMR (CDCl3, 100 MHz, δ (ppm)): 166.43, 156.29, 156.15, 147.52, 145.66, 139.63, 135.99, 133.73, 132.32, 131.97, 131.88, 131.11, 129.43, 129.15, 128.73, 128.45, 127.80, 127.11, 126.47 (q, J = 5 Hz, C11), 124.51, 123.70, 123.62 (q, J = 271.25 Hz, C10), 122.3 (q, J = 31.25 Hz, C9), 119.97, 119.69.
4,9-Bis-[4-(4′-amino-3-trifluoromethyl-biphenyl-4-yloxy)-phenyl]-2-phenyl-benzo[f]-isoindole-1,3-dione (8).
In a round bottom flask equipped with a hydrogen balloon, the dinitro compound (7) (10 g, 10.12 mmole) and 1% palladium in charcoal (0.36 g) were taken into THF (20 mL). The mixture was stirred for 36 h maintaining sufficient hydrogen pressure in a balloon. After completion of the reaction as monitored by TLC, the reaction mixture was filtered to remove the catalyst and dried under vacuum. The crude product was finally purified by using silica gel column chromatography using dichloromethane as the eluent. Yield: 6.1 g (65%). Mp: 311.0 °C. Anal.Calcd. for C56H35F6N3O4 (927.89 g mol−1): C, 72.49%; H, 3.80%; N, 4.53%; Found: C, 72.74%; H, 3.13%; N, 4.29%. FTIR (KBr, cm−1): 3468, 3381 (N–H stretching), 3071, 3037 (aromatic C–H stretching), 1766 (asymmetric imide C
O stretching), 1718 (symmetric imide C
O stretching), 1619 (aromatic C
C ring stretching band), 1491, 1330 (asymmetric C–O–C stretch), 1248, 1125 (band due to C–F absorption), 1053 (symmetric C–O–C stretch), 822 (C–N stretch for aromatic—NH2). 1H–NMR (CDCl3, 400 MHz, δ (ppm)): 7.93 (dd, J = 6.4 Hz, J' = 3.2 Hz, 2H, H23), 7.84 (d, J = 2.0 Hz, 2H, H11), 7.67–7.62 (m, 5H, H6, H18, H25), 7.48 (d, J = 8.4 Hz, 4H, H14), 7.43–7.37 (m, 8H, H3, H24, H19), 7.24 (d, J = 8.4 Hz, 4H, H13), 7.18 (d, J = 8.4 Hz, 2H, H7), 6.76 (d, J = 8.4 Hz, 4H, H2), 3.77 (bs, 4H, −NH2 protons). 13C–NMR (CDCl3, 100 MHz, δ (ppm)): 166.45, 157.31, 153.45, 146.46, 139.79, 136.82, 136.07, 131.89, 131.74, 131.10, 130.17, 129.61, 129.29, 129.06, 128.77, 128.29, 128.01, 127.13, 125.02 (q, J = 5 Hz, C11), 123.63, 123.51 (q, J = 271.1 Hz, C10), 122.01 (q, J = 31.1 Hz, C9), 120.57, 118.89, 115.61.
Polymerization and preparation of the membrane
The polymerization of the synthesized bis(ether amine) with five different aromatic dianhydrides were achieved in two conventional stages as presented in Scheme 2. A representative polymerization reaction of the bis(ether amine) (8) with bisphenol-A dianhydride (BPADA) is as follows: in the first stage, in a 25 mL round bottomed flask equipped with a guard tube, BPADA (0.25 g, 0.48 mmole) was added in portions into the solution of bis(ether amine), BIDA (8) (0.45 g, 0.48 mmole) in dry DMF (7.0 mL). The mixture was left to stir at room temperature while it transformed to a highly viscous solution of polyamic acid (PAA) within 40–60 min. In the second stage, viscous PAA solution was spread onto a clean Petri dish and kept in oven initially at 80 °C for the slow removal of the solvent. Finally, the thermal cyclization of the PAA to PEI was achieved by sequential heating at 120, 150, 180, 200 and 220 °C, each for half an hour and at 250 °C for 15 min in an oven under nitrogen. All the membranes were removed from the Petri dishes after immersing in hot water. All the polymers in this series lead to clear, flexible and tough membranes after the thermal imidization except BIDA-PMDA which was brittle in nature. The average thickness of the membranes for BIDA-BPADA, BIDA-6FDA, BIDA-BTDA and BIDA-ODPA was 62, 71, 76, and 83 μm, respectively. Typical physical properties and calculated fractional free volume of the PEI membranes are summarized in Table 1. Since the required van der Waals volume components related to the benzoisoindoledione moiety was not reported in literature, the fractional free volumes (FFV) of the PEI membranes were not possible to calculate using group contribution method.39
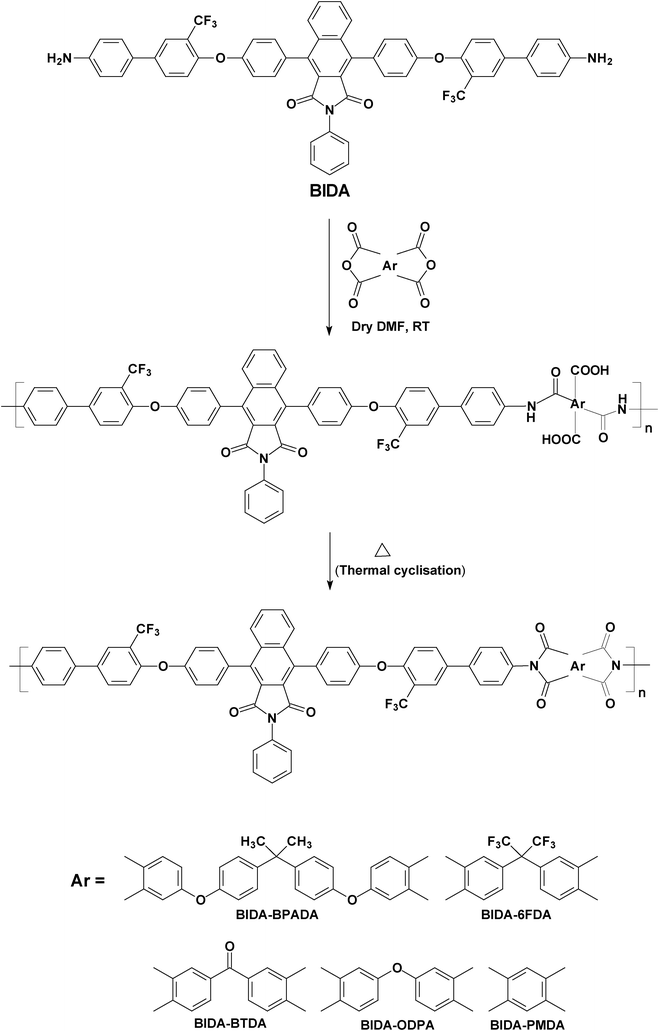 |
| Scheme 2 Synthesis of the poly(ether imide)s. | |
Table 1 Physical properties of the polyimides
Polymer |
Tda/Tdb (°C) |
Tgc (°C) |
Tensile strength (MPa) |
Young’s modulus (GPa) |
Elongation at break (%) |
η
inh
(dL/g) |
Mwe (g mol−1) |
PDIf |
5% weight loss temperature in air.
10% weight loss temperature in air.
Glass transition temperature (Tg) measured from DSC at a heating rate of 20 °C min−1.
Inherent viscosity measured at 30 ± 0.5 °C.
Number average molecular weight; polystyrene calibration.
Polydispersity index.
|
BIDA-BPADA |
536/565 |
274 |
102.1 ± 4.9 |
0.41 ± 0.03 |
17.4 ± 2.3 |
0.61 |
98,000 |
1.99 |
BIDA-6FDA |
527/555 |
315 |
94.8 ± 3.1 |
0.46 ±0.01 |
25.8 ± 2.9 |
0.52 |
84,500 |
1.92 |
BIDA-BTDA |
557/588 |
310 |
47.5 ± 1.6 |
0.70 ± 0.12 |
9.1 ± 1.8 |
— |
— |
— |
BIDA-ODPA |
559/592 |
301 |
97.3 ± 3.2 |
1.50 ± 0.35 |
6.0 ± 1.1 |
0.56 |
71,000 |
1.83 |
BIDA-PMDA |
532/575 |
335 |
— |
— |
— |
— |
— |
— |
BIDA-BPADA.
Feed composition: BIDA: 0.4484 g, 0.4833 mmol; BPADA: 0.2516 g, 0.4833 mmol; dry DMF: 7 mL.
Anal.Calcd. for C87H51F6N3O10 (1412.34 g mol−1): C, 73.99%; H, 3.64%; N, 2.98%; Found: C, 74.35%; H, 3.39%; N, 2.81%. FTIR (KBr, cm−1): 3074, 2924 (aromatic C–H stretching), 2850 (aliphatic C–H stretching), 1772 (asymmetric imide C
O stretching), 1716 (symmetric imide C
O stretching), 1598 (aromatic C
C stretching), 1483, 1364 (asymmetric C–O–C stretching), 1237, 1121 (C–F stretching), and 1048 (symmetric C–O–C stretching). 1H–NMR (CDCl3, 500 MHz, δ (ppm)): 7.89–7.84 (m, 6H, H11, H18, H31), 7.67 (d, J = 8.5 Hz, 2H, H23), 7.63 (d, J = 8.5 Hz, 4H, H2), 7.61–7.60 (m, 2H, H19), 7.48 (d, J = 8.0 Hz, 4H, H3), 7.45 (d, J = 8.0 Hz, 4H, H14), 7.39 (d, J = 2.0 Hz, 2H, H28), 7.37 (d, J = 7.5 Hz, 2H, H6), 7.34–7.32 (m, 4H, H24, H30), 7.28 (d, J = 8.5 Hz, 4H, H36), 7.22 (d, J = 8.5 Hz, 4H, H13), 7.19–7.16 (m, 3H, H7, H25), 6.99 (d, J = 9.0 Hz, 4H, H35), 1.7 (s, 6H, H38). 13C–NMR (CDCl3, 125 MHz, δ (ppm)): 166.71, 166.65, 166.23, 163.90, 156.58, 154.77, 152.67, 147.59, 139.52, 138.92, 135.85, 135.35, 134.22, 131.87, 131.68, 131.35, 130.49, 129.17, 128.91, 128.74, 128.65, 128.57, 128.16, 127.63, 126.91, 126.88, 125.88, 125.92, 125.79, 125.00, 123.50, 123.30 (q, J = 271.25 Hz, C10), 123.11, 121.85 (q, J = 31.25 Hz, C9), 119.98, 119.19, 111.89, 42.55, 30.99.
BIDA-6FDA.
Feed composition: BIDA: 0.4734 g, 0.5102 mmol; 6-FDA: 0.2266 g, 0.5102 mmol; dry DMF: 7 mL.
Anal.Calcd. for C75H37F12N3O8 (1336.09 g mol−1): C, 67.42%; H, 2.79%; N, 3.14%; Found: C, 67.68%; H, 2.49%; N, 3.29%. FTIR (KBr, cm−1): 3065, 2923 (aromatic C–H stretching), 1770 (asymmetric imide C
O stretching), 1717 (symmetric imide C
O stretching), 1600 (aromatic C
C stretching), 1487, 1366 (asymmetric C–O–C stretching), 1242, 1121 (C–F stretching), and 1048 (symmetric C–O–C stretching). 1H–NMR (CDCl3, 125 MHz, δ (ppm)): 8.09 (d, J = 8.0 Hz, 2H, H31), 7.97 (s, 2H, H28), 7.96–7.90 (m, 6H, H2, H11), 7.76–7.72 (m, 7H, H6, H18, H23, H25), 7.69–7.67 (m, 2H, H30), 7.56 (d, J = 8.5 Hz, 4H, H3), 7.52 (d, J = 8.5 Hz, 4H, H14), 7.44 (d, J = 7.5 Hz, 2H, H19), 7.40 (d, J = 7.0 Hz, 2H, H24), 7.36 (d, J = 7.0 Hz, 2H, H7), 7.30 (d, J = 8.5 Hz, 4H, H13). 13C–NMR (CDCl3, 125 MHz, δ (ppm)): 166.28, 166.05, 165.88, 156.53, 154.93, 139.54, 139.48, 139.26, 136.00, 135.87, 135.17, 132.66, 132.37, 131.90, 131.71, 131.67, 130.82, 130.55, 129.21, 128.95, 128.60, 128.24, 128.21, 127.83, 126.95, 125.98, 125.90, 125.50, 125.46, 124.24, 123.50, 123.29 (q, J = 271.25 Hz, C10), 121.89 (q, J = 32.5 Hz, C9), 119.98, 119.26.
BIDA-BTDA.
Feed composition: BIDA: 0.4951 g, 0.6358 mmol; BTDA: 0.2049 g, 0.6358 mmol; dry DMF: 7 mL.
Anal.Calcd. for C73H37F6N3O9 (1214.08 g mol−1): C, 72.22%; H, 3.07%; N, 3.46%; Found: C, 72.16%; H, 2.98%; N, 3.78%. FTIR (KBr, cm−1): 3061, 2923 (aromatic C–H stretching), 1772 (asymmetric imide C
O stretching), 1713 (symmetric imide C
O stretching), 1598 (aromatic C
C stretching), 1485, 1365 (asymmetric C–O–C stretching), 1239, 1119 (C–F stretching), and 1046 (symmetric C–O–C stretching). 1H–NMR and 13C–NMR spectra could not be recorded due to insolubility of this polymer in CDCl3 and DMSO-d6 at room temperature.
BIDA-ODPA.
Feed composition: BIDA: 0.5006 g, 0.6428 mmol; ODPA: 0.1994 g, 0.6428 mmol; dry DMF: 7 mL.
Anal.Calcd. for C72H37F6N3O9 (1202.07 g mol−1): C, 71.94%; H, 3.10%; N, 3.50%; Found: C, 71.87%; H, 2.98%; N, 3.34%. FTIR (KBr, cm−1): 3065, 2922 (aromatic C–H stretching), 1772 (asymmetric imide C
O stretching), 1713 (symmetric imide C
O stretching), 1602 (aromatic C
C stretching), 1484, 1362 (asymmetric C–O–C stretching), 1234, 1118 (C–F stretching), and 1046 (symmetric C–O–C stretching). 1H–NMR (CDCl3, 500 MHz) δ (ppm): 7.96 (d, J = 7.0 Hz, 2H, H31), 7.91–7.89 (m, 4H, H11, H18), 7.66 (s, 2H, H28), 7.64 (d, J = 8.5 Hz, 4H, H2), 7.60 (d, J = 7.0 Hz, 2H, H6), 7.51–7.48 (m, 6H, H3, H23), 7.44 (d, J = 8.0 Hz, 4H, H14), 7.36 (d, J = 7.0 Hz, 2H, H19), 7.32 (d, J = 7.0 Hz, 2H, H24), 7.28 (d, J = 7.0 Hz, 2H, H30), 7.21 (d, J = 7.0 Hz, 4H, H13), 7.17–7.16 (m, 3H, H7, H25). 13C–NMR (CDCl3, 125 MHz, δ (ppm)): 166.26, 166.20, 166.08, 161.23, 156.55, 154.85, 139.53, 139.18, 135.86, 135.26, 134.61, 131.89, 131.70, 131.10, 130.52, 129.19, 128.93, 128.58, 128.18, 127.72, 127.30, 126.93, 126.89, 126.55, 126.33, 125.97, 124.93, 123.50, 123.30 (q, J = 271.25 Hz, C9), 121.87 (q, J = 31.25 Hz, C10), 119.99, 119.22, 114.04.
BIDA-PMDA.
Feed composition: BIDA: 0.5468 g, 0.7022 mmol; PMDA: 0.1532 g, 0.7022 mmol; dry DMF: 7 mL.
Anal.Calcd. for C66H33F6N3O8 (1109.97 g mol−1): C, 71.42%; H, 3.00%; N, 3.79%; Found: C, 71.55%; H, 3.36%; N, 3.81%. FTIR (KBr, cm−1): 3038, 2923 (aromatic C–H stretching), 1771 (asymmetric imide C
O stretching), 1716 (symmetric imide C
O stretching), 1599 (aromatic C
C stretching), 1485, 1362 (asymmetric C–O–C stretching), 1238, 1118 (C–F stretching), and 1047 (symmetric C–O–C stretching). 1H–NMR and 13C–NMR spectra could not be recorded due to insolubility of this polymer in CDCl3 and DMSO-d6 at room temperature.
Measurements of gas transport properties
The gas transport properties of these PEI membranes were studied at 3.5 atm of applied gas pressure and at 35, 45 and 55 °C using automated Diffusion Permeameter (DP-100-A) manufactured by Porous Materials, Inc., USA. The permeation cell was placed in a thermostatically controlled housing for isothermal measurement conditions. The order of testing the permeability of the gases through the polymer membranes was first, under CH4, followed by N2, O2 and finally under CO2. All the membranes were degassed for at least 24 h at the operating temperature within the permeation cell prior to the experiments. The effective permeation area (A) was 5.069 cm2. To the upstream side of the membrane the gas pressure (pi = 3.5 atm) was applied instantaneously and in the downstream side a reservoir of constant volume (119 cm3) was connected with a pressure transducer (MKS Barton) to monitor the total amount of gas which passed through the polymer membrane. All the measurements were done using XL grade gases purchased from BOC Gases, India. The time-lag method was employed for the gas transport measurements. This technique allows the determination of the mean permeability coefficient P from the steady state gas pressure increment (dp/dt)s in the calibrated volume V of the product side of the cell. The permeability coefficients are reported in Barrer and were calculated from eqn (1), where T0 and p0 are the standard temperature and pressure (T0 = 273.15 K, p0 = 1.013 atm), T is the temperature of measurement, d is the thickness of the membrane and (dp/dt)s was obtained from the slope of the increments of downstream pressure vs. time plot. The reproducibility of the measurements was better than ±5% for CO2 and O2 permeability measurements whereas the reproducibility for CH4 and N2 permeability measurements was in the range ±10%. The mean permeability was calculated from the following equation. | P = [VdT0/Apip0T] (dp/dt)s | (1) |
The effective diffusion coefficient D was calculated from the time-lag θ according to the following eqn (2):
The ideal permselectivity of the membranes towards a gas ‘A’ relative to another gas ‘B’ was calculated from the individual gas permeabilities using the following eqn (3):
The ideal diffusivity selectivity of a membrane towards a gas ‘A’ relative to another gas ‘B’ was calculated from the calculated diffusion coefficients using the following eqn (4):
The solubility coefficient S of each gas for all the polymers was calculated from the corresponding permeability coefficient (P) and diffusion coefficient (D) values by following the eqn (5):
The solubility selectivity of the membranes for a gas ‘A’ relative to another gas ‘B’ was calculated from their individual solubility coefficient using the following eqn (6):
Results and discussion
Syntheses of monomers
The bis(ether amine) monomer ‘8’ was prepared starting from commercially available and very cheap phenolphthalein following the reaction scheme as shown in Scheme 1. Earlier, Strukelj and Hay reported the preparation of poly(imidoaryl ether ketone)s and poly(imidoaryl ether sulfone)s with high Tg and thermal stability using a benzoisoindoledione moiety in the polymer backbone.40 From this work we were interested to include the same bishydroxy compound to prepare a new series of fluorinated polyimides and to investigate the effect of this moiety on the properties of polyimides which is not reported yet. The reaction protocol for the preparation of bishydroxy intermediate (6) from phenolphthalein was followed as developed by Hay and co-workers38 except for the first reaction step. The first step involved the reduction of phenolphthalein (1) to phenolphthalin (2) and this step has been optimized in our own laboratory. The other starting material, 4-fluoro-4'-nitro-3-trifluoromethyl-biphenyl (3) was reported in our previous publication.41 These two starting materials lead to a fluorinated bis(ether amine) containing highly rigid benzoisoindoledione moiety. In combination with this rigid benzoisoindoledione unit, a pendent trifluoromethyl group and two ether linkages have been included into the diamine monomer to form highly organosoluble i.e., easily processable as well as highly thermally stable poly(ether imide)s.
Syntheses of PEIs
A new series of PEIs was synthesized using pendent trifluoromethyl and cardo benzoisoindoledione moieties containing bis(ether amine, ‘8’), and various fluorinated and non-fluorinated aromatic dianhydrides by the conventional ring opening polyaddition of bis(ether amine) to cyclic anhydrides to form poly(amic acid)s followed by thermal cyclization to poly(ether imide)s. Among these newly synthesized five polymers; BIDA-BPADA, BIDA-6FDA, BIDA-BTDA and BIDA-ODPA were capable of forming free standing films whereas the remaining polymer, BIDA-PMDA, was brittle in nature. BIDA-BPADA, BIDA-6FDA and BIDA-ODPA were highly soluble (10% w/v) at room temperature in various high boiling polar organic solvents, such as N,N-dimethyl acetamide (DMAc), N-methylpyrrolidone (NMP), DMF, and pyridine as well as in many low boiling solvents like THF, dichloromethane (DCM) and chloroform (CHCl3) at room temperature. BIDA-BTDA and BIDA-PMDA were insoluble in common organic solvents. The insolubility for BIDA-PMDA is expected from its highly rigid backbone. Both the benzoisoindoledione and PMDA unit lead to high rigidity of this polyimide. However, the insolubility of BIDA-BTDA is not only from the rigid benzoisoindoledione unit; the carbonyl group present in BTDA also plays a critical role in making this PEI highly insoluble and intractable. Possibly, during the thermal treatment for the conversion of PAA to PEI, the carbonyl group present in BTDA reacts with the end amine functionalities, leading to crosslinking.
Because of the extra reactive carbonyl group in BTDA with respect to other anhydrides, BTDA can behave as a B3 type monomer, and this carbonyl group can react with the amine end group (A2) present in the polymer chain that may possibly lead to crosslinked polyimide by the A2+B3 approach. This phenomenon within this class of polyimides (BTDA based) may also be due to a different chain packing arrangement that may render this polyimide (BIDA-BTDA) as insoluble. All the PEIs in the series were amorphous in nature as observed by an XRD study (broad peaks).
The structures of all the PEIs were well characterized by FTIR and NMR spectroscopies and a representative example of the FTIR and 1H-NMR spectra of BIDA-6FDA is presented in Fig. 1. Complete imidization of the poly(ether imide)s was further confirmed by FTIR spectroscopy. The stretching frequencies present in poly(amic acid) near 3200 cm−1 (–O–H stretch) and 3350 cm−1 (> N–H stretch) had disappeared completely for all the membranes. All the polymers showed characteristic absorption due to asymmetric >C
O stretching and symmetric >C
O stretching of the imide bands at ∼1770 cm−1 and ∼1715 cm−1, respectively. All the other characteristic absorption bands at ∼1485 and ∼1365 cm−1 corresponding to asymmetric C–O–C stretching, ∼1600 cm−1 to aromatic C
C stretching and at ∼1240 and ∼1120 cm−1 to C–F stretching also appeared. The molecular structure of the polymer backbone was confirmed by the 1H-NMR and 13C-NMR spectra. All the polymers showed the exact number of desired 1H and 13C signals in both aliphatic and aromatic regions. In the 1H-NMR spectra, no signal was observed above 10 ppm which again confirmed the complete imidization. In proton decoupled 13C-NMR spectra the C–F coupling constant values for each quartet for one bond coupling (1JC–F) were around 271 Hz and around 31 Hz for the two bond coupling (2JC–F).
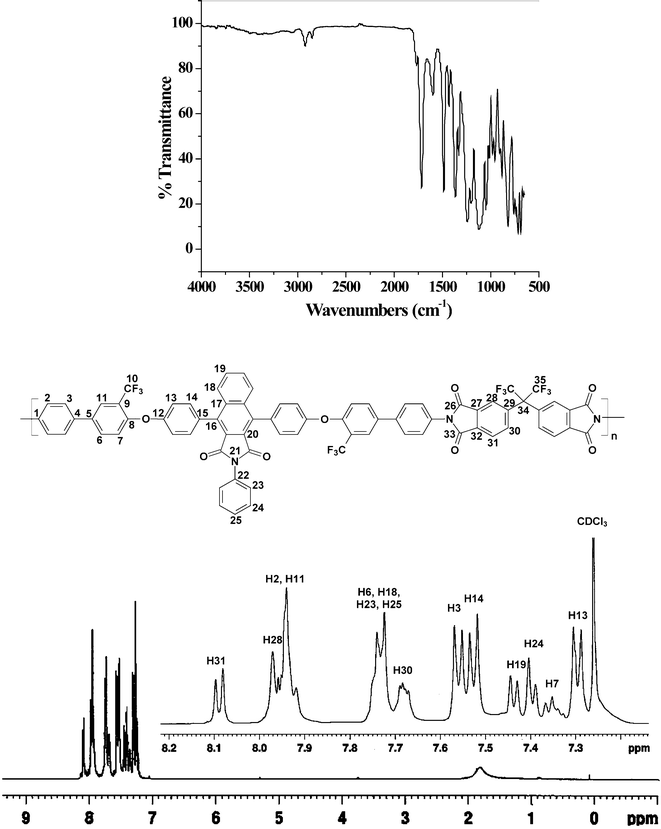 |
| Fig. 1 FTIR and 1H-NMR spectrum of BIDA-6FDA. | |
Properties of the PEIs
Thermal analysis revealed that all the PEIs in the series have outstanding thermal stability (Fig. 2). In spite of the amorphous structure, all these PEIs showed excellent thermo-oxidative stability, 5% wt loss temperature ranging from 527 to 559 °C and 10% wt. loss temperature from 555 to 592 °C. The glass transition temperature values observed were in the range 274–335 °C (Fig. 3). These polymers did not show any melting or crystallization at temperatures up to 350 °C. The polymer BIDA-BPADA showed the lowest Tg among the series. This may be attributed to the presence of extra flexible ether linkages in the BPADA unit whereas the highest Tg was observed for the PMDA unit containing BIDA-PMDA due to the rigidity in the polymer backbone. The order of Tg in the polymer series is in good agreement with the increasing order of rigidity and polarity of the polymer chains. BIDA-6FDA containing two extra –CF3 group from the dianhydride 6FDA was found to be a higher Tg material in comparison to the very similar structure of polymer BIDA-ODPA. These polymers showed both higher Tg and thermal decomposition temperatures in comparison to previously reported phthalimidine (BAPA) based polyimides,23 which is attributed to the more rigid backbone structure of this class of polymers.
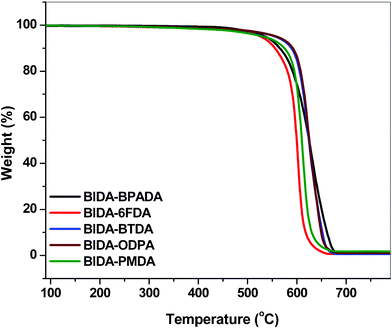 |
| Fig. 2 TGA plots of the poly(ether imide)s (heating rate, 10 °C min−1 in air). | |
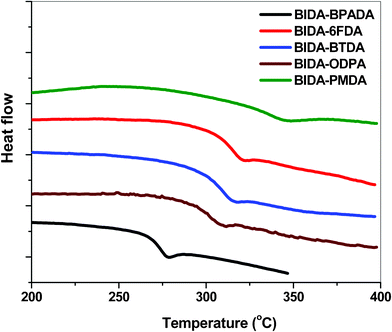 |
| Fig. 3 DSC plots of the poly(ether imide)s (heating rate, 20 °C min−1 in nitrogen). | |
Tensile strength is an important parameter for the membranes to be used for gas separation applications. Membranes have to withstand high pressure difference at elevated temperature for industrial applications, especially for landfill gas upgradation or hydrocarbon recovery or for pollution control. Good tensile strength as well as high thermo-oxidative stability and higher Tg of BIDA-BPADA, BIDA-6FDA, BIDA-BTDA and BIDA-ODPA make these PEIs suitable for gas separation applications. The polymers exhibited good tensile strength up to 102 MPa and Young’s modulus up to 1.5 GPa. The elongation at break for these polymers ranges between 6–26%. Low elongation at break values is observed due to the highly rigid benzoisoindoledione moiety and absence of any extendable geometry in the polymer structures. BIDA-PMDA was brittle in nature due to the high rigidity of the main chain. So, the gas transport property for this PEI was not possible.
Gas separation properties
Effect of chemical structures.
The mean permeability coefficients of four different gases through these four structurally different PEIs and ideal permselectivity values for different gas pairs through these membranes is presented in Table 2. The investigation of gas transport behavior for BIDA-PMDA was not possible due to the brittle nature of that polymer. The diffusion coefficients were calculated from the time-lag value of gas flow vs. time plot. The diffusivity coefficients and solubility coefficients of all the gases for these PEIs are tabulated in Table 3. Their diffusivity selectivity and solubility selectivity values are summarized in Table 4.
Table 2 Gas permeation properties of the poly(ether imide)sd
Polymer |
P (CO2) |
P (O2) |
P (N2) |
P (CH4) |
α (CO2/CH4) |
α (O2/N2) |
α (CO2/N2) |
α (CO2/O2) |
Values taken from ref. 31; gas permeability coefficient (P) measured at 35 °C and 3.5 bar.
Values taken from ref. 25. P (CO2) and P (CH4) measured at 10 atm of applied pressure. P (O2) and P (N2) measured at 2 bar of applied pressure.
Values taken from ref. 29. The upstream pressure was maintained at 3 bar at 30 °C.
P = gas permeability coefficient measured at 35 °C and 3.5 bar. α = permselectivity values. 1 Barrer = 10−10 cm3 (STP) cm cm−2 s cm Hg.
|
BIDA-BPADA |
25.65 |
10.32 |
1.23 |
0.69 |
37.17 |
8.39 |
20.85 |
2.49 |
BIDA-6FDA |
71.32 |
25.37 |
4.22 |
1.99 |
35.84 |
6.01 |
16.90 |
2.81 |
BIDA-BTDA |
16.06 |
6.99 |
0.98 |
0.55 |
29.20 |
7.13 |
16.39 |
2.30 |
BIDA-ODPA |
16.99 |
7.74 |
1.45 |
0.67 |
25.36 |
5.34 |
11.72 |
2.19 |
BAPA-BPADAa |
16.61 |
4.25 |
0.95 |
0.84 |
19.77 |
4.47 |
17.48 |
3.91 |
BAPA-6FDAa |
53.85 |
10.23 |
1.71 |
1.01 |
53.32 |
5.98 |
31.49 |
5.26 |
BAPA-BTDAa |
17.09 |
4.33 |
0.83 |
0.62 |
27.57 |
5.22 |
20.59 |
3.95 |
BAPA-ODPAa |
14.59 |
4.22 |
0.96 |
0.84 |
17.37 |
4.40 |
15.20 |
3.46 |
BATPHF-6FDAb |
22.8 |
6.50 |
1.30 |
0.70 |
32.57 |
5.00 |
17.54 |
3.51 |
BATPHF-BTDAb |
6.94 |
2.17 |
0.37 |
0.19 |
36.53 |
5.87 |
18.76 |
3.20 |
BATPHF-PMDAb |
24.60 |
7.06 |
1.50 |
0.94 |
26.17 |
4.71 |
16.40 |
3.48 |
PI-ADA-1c |
14.39 |
3.79 |
0.72 |
0.39 |
36.89 |
5.26 |
19.99 |
3.77 |
PI-ADA-2c |
16.06 |
4.79 |
0.83 |
0.45 |
35.69 |
5.77 |
19.35 |
3.35 |
PI-ADA-3c |
12.93 |
3.23 |
0.67 |
0.37 |
34.95 |
4.82 |
19.30 |
4.00 |
Table 3 Gas diffusion coefficients, D × 108 (cm2 s−1) and solubility coefficients in 10−2 cm3 (STP)/cm3 cm Hg of the poly(ether imide)s at 35 °C and 3.5 atma
Polymer |
CO2 |
O2 |
N2 |
CH4 |
D
|
S
|
D
|
S
|
D
|
S
|
D
|
S
|
S = gas solubility coefficients (from S = P/D, in cm3 (STP)/cm3 cm Hg).
|
BIDA-BPADA |
2.54 |
10.09 |
11.44 |
0.90 |
1.95 |
0.63 |
1.64 |
0.42 |
BIDA-6FDA |
3.28 |
21.74 |
14.00 |
1.81 |
3.32 |
1.27 |
1.48 |
1.35 |
BIDA-BTDA |
1.26 |
12.75 |
6.30 |
1.11 |
1.59 |
0.62 |
1.54 |
0.36 |
BIDA-ODPA |
1.85 |
9.18 |
5.87 |
1.32 |
1.93 |
0.75 |
1.63 |
0.41 |
Table 4 Diffusivity selectivity (αD) and solubility selectivity (αS) values for different gas pairs of the poly(ether imide)s at 35 °C and 3.5 atma
Polymer |
α
D
|
α
S
|
(CO2/CH4) |
(O2/N2) |
(CO2/N2) |
(CO2/O2) |
(CO2/CH4) |
(O2/N2) |
(CO2/N2) |
(CO2/O2) |
α
D
= diffusivity selectivity values of gas pairs. αS = solubility selectivity values of gas pairs.
|
BIDA-BPADA |
1.55 |
5.87 |
1.30 |
0.22 |
24.02 |
1.43 |
16.02 |
11.21 |
BIDA-6FDA |
2.22 |
4.22 |
0.99 |
0.23 |
16.10 |
1.42 |
17.12 |
12.01 |
BIDA-BTDA |
0.82 |
3.96 |
0.79 |
0.20 |
35.42 |
1.79 |
20.57 |
11.49 |
BIDA-ODPA |
1.14 |
3.04 |
0.96 |
0.32 |
22.39 |
1.76 |
12.24 |
6.96 |
The gas permeability coefficient through all these PEIs decreases following the sequence P (CO2) ≫ P (O2) > P (N2) > P (CH4), which is typically in the reversed order of their respective kinetic diameter, CO2 (3.3 Å) < O2 (3.46 Å) < N2 (3.64 Å) < CH4 (3.8 Å).42 In contrast to that, the diffusivity order was not in the same trend. The diffusivity of these gases was observed to be in the order of D (O2) ≫ D (CO2) > D (N2) > D (CH4) for all the PEIs. It is obvious from the kinetic diameter data that CO2 should diffuse faster compared to O2. So, from these observations it can be concluded that, there are little or no interaction of O2, N2 and CH4 with these polymer materials, whereas CO2 has high interaction with these polymers. Higher interaction of CO2 in PEIs is possibly arising from the presence of carbonyl or imide groups in PEI backbone and the high permeability of CO2 results from the enhanced solubility coefficient values for all the PEIs. It is also well evident in the literature that the solubility coefficients for CO2 in polyimides are exceptionally high compared to other gases.25,27
BIDA-6FDA containing two extra bulky and rigid pendent trifluoromethyl groups from the hexafluoroisopropylidene moiety exhibited the highest permeability for all the gases. BIDA-BTDA based on the BTDA moiety showed the lowest permeability coefficients for all gases among the series. This is possibly due to the presence of one additional carbonyl group from the BTDA unit in the main chain of BIDA-BTDA. The presence of this additional carbonyl group favors the intermolecular charge transfer complex formation, which, in turn, leads to closer packing of the polymer chains. This closer packing probably makes this polymer the least permeable for all the gases. Alternatively, enhanced gas barrier properties through the polymer–clay composites are due to an increased diffusion length of a permeating molecule, also known as a tortuous pathway.9–13 It can be seen from Table 3 that BIDA-6FDA has both the highest diffusion coefficient and solubility coefficient for all the gases. Presence of two trifluoromethyl groups in 6-FDA makes the polyimide chains bulkier, increase the rigidity and fractional free volume thereby increasing the permeability as well as the selectivity of different gas pairs.24–27 Moreover, BIDA-6FDA with bulky pendent –CF3 groups exhibited a larger solubility coefficient as well. All these facts were also observed by Okamoto and co-workers.25 A detailed analysis of the data from Table 2 and Table 4 reveals that the overall selectivity i.e., the permselectivities for CO2/O2, CO2/N2 and CO2/CH4 are mainly due to the solubility selectivities as the diffusion selectivities are relatively small.
BAPA
BATPHF
PI-ADA
It is generally believed that an increase in glass transition temperature (Tg) increases the chain stiffness which, in turn, increases the permselectivities.18 However, the observation from Table 2 followed a different trend. The trends of Tg of these polymers, BIDA-6FDA > BIDA-BTDA > BIDA-ODPA > BIDA-BPADA does not match with their permselectivity order for any gas pair. Higher –CF3 content in BIDA-6FDA compared to the other three PEIs in the series increases the permeability coefficient for all the gases in a large extent rendering this BIDA-6FDA with a little lower selective than BIDA-BPADA.
Comparison with other fluorinated poly(ether imide) membranes
As per our best knowledge benzoisoindoledione based poly(ether imide) has not been reported in the literature so far. Accordingly, the ideal gas separation performance of this series of polymers have been compared with closely molecularly structured dihydroisoindolone based BAPA-BPADA, BAPA-6FDA, BAPA-BTDA and BAPA-ODPA,31 and some of the previously reported fluorinated poly(ether imide)s e.g., BATPHF-6FDA, BATPHF-BTDA, BATPHF-PMDA, PI-ADA-1, PI-ADA-2 and PI-ADA-325,29 (Table 2). For a better understanding, the comparison of permselectivity for the CO2/CH4 gas pair versus permeability coefficient of CO2 of this polymer series with other PEIs is presented as a Robeson plot in Fig. 4. Fig. 5 represents a comparison of the permselectivity for an O2/N2 gas pair versus permeability coefficient of O2 of these PEIs with other previously reported PEIs. All the polyimides from the current series showed higher oxygen permeability and it is increased up to 148% in case of BIDA-6FDA in comparison to BAPA-6FDA. An increase in permeability without losing permselectivity has always been a thrust research area.43 A BIDA based polyimide also exhibited a good enhancement in CO2 permeability. The CO2 permeability was increased up to 32% in the case of BIDA-6FDA in comparison to BAPA-6FDA (PCO2 for BAPA-6FDA was 53.85 Barrer whereas the same for BIDA-6FDA was 71.32 Barrer). Thus, in comparison to the previously reported BAPA based polyimides, the polyimides from this current series exhibited better performance in respect to both the permeability of O2 and permselectivity toward O2/N2 gas pair which render this series of polymers closer to upper boundary limit. Especially, BIDA-BPADA surpass the upper boundary limit drawn by Robeson.32 Compared to BATPHF based PEIs these new polymers showed better separation performances for both O2/N2 and CO2/CH4 gas pairs. Among all the polymers in the series only the BTDA based BIDA-BTDA showed slightly lower permselectivity toward CO2 over CH4 (29.2) in comparison to BATPHF-BTDA (36.5). Recently, Maya et al.29 reported a few processable copolyimides, based mainly on 6-FDA as the dianhydride and using adamantane as the pendent group. The gas permeability through these polymeric membranes has also been presented in Table 2. It is important to mention that in comparison to these copolyimide membranes, BIDA-6FDA not only showed much higher permeability for all the gases but also showed slightly higher permselectivity for O2/N2 gas pair. The permeability value for CO2 and O2 for BIDA-6FDA was observed to be higher by more than 300% and 400%, respectively. However, the permselectivity for CO2/CH4 gas was found to be marginally lower (less than ∼3%) for BIDA-6FDA.
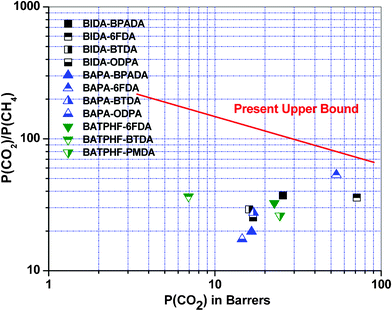 |
| Fig. 4 Robeson plot32 for a comparison of CO2/CH4 selectivity vs. CO2 permeability coefficients of the poly(ether imide)s with some other polymers reported earlier, values taken from ref. 31, 25. | |
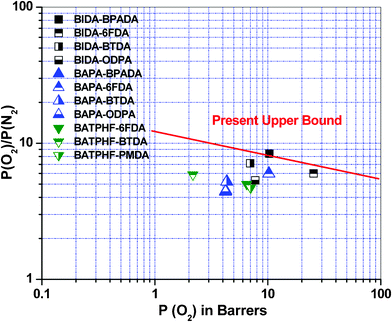 |
| Fig. 5 Robeson plot32 for a comparison of O2/N2 selectivity vs. O2 permeability coefficients of the poly(ether imide)s with some other polymers reported earlier, values taken from ref. 31, 25. | |
Effect of temperature on gas permeabilities, diffusivities and selectivities
The increment of the permeability and diffusion coefficients of the gases on the increase in temperature is tabulated in Table 5 and Table 6, respectively. The permeability coefficients increase with the temperature (in the range 35° to 55 °C) as can be observed from the permeability (P) vs. temperature (T) plots (Fig. 6). The permselectivity values decrease with increasing temperature, as observed from the permselectivity vs. temperature plots (Fig. 7). The frequency of the intersegmental jumps of the gas molecules as well as the polymer chain mobility is directly affected by the temperature. Therefore, as the temperature increases the diffusion rate as well as permeation rate also increases. At the same time, the chain segment motions may become wider, and low selectivity for a gas pair is the consequence.43 The diffusion coefficients also showed temperature dependency (Fig. 8). The diffusivity selectivity of CO2/CH4versus temperature plot is shown in Fig. 9.
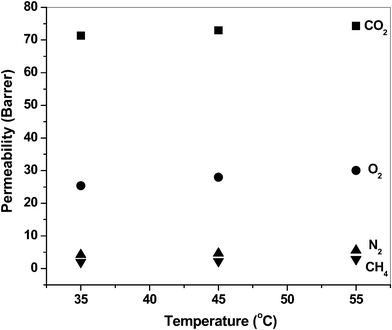 |
| Fig. 6 Representative plots of permeability vs. temperature for BIDA-6FDA. | |
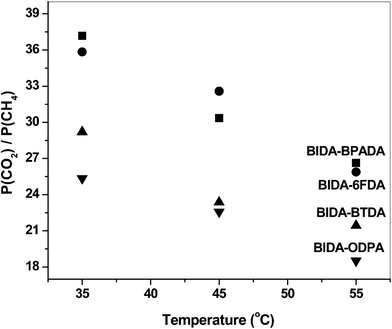 |
| Fig. 7 Effect of temperature on permselectivities. | |
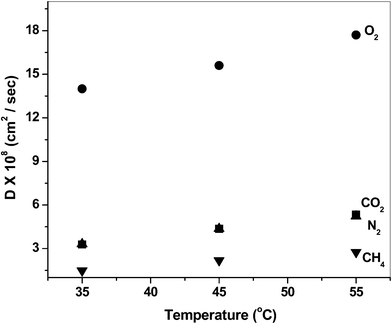 |
| Fig. 8 Representative plots of diffusivity vs. temperature for BIDA-6FDA. | |
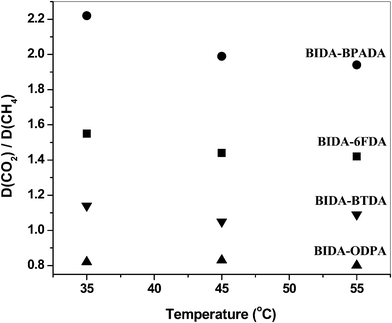 |
| Fig. 9 Effect of temperature on diffusivity selectivities. | |
Table 5 Gas permeability coefficients (P) in Barrer at different temperatures through the poly(ether imide) membranesa
Polymer |
Permeability (P in barrer) |
CO2 |
O2 |
N2 |
CH4 |
35 °C |
45 °C |
55 °C |
35 °C |
45 °C |
55 °C |
35 °C |
45 °C |
55 °C |
35 °C |
45 °C |
55 °C |
The possible errors for the permeability measurements for CO2 and O2 were within ±5% whereas for CH4 and N2 errors were in the range ±10%.
|
BIDA-BPADA |
25.65 |
27.32 |
28.49 |
10.32 |
11.66 |
12.79 |
1.23 |
1.55 |
1.76 |
0.69 |
0.90 |
1.07 |
BIDA-6FDA |
71.32 |
72.97 |
74.30 |
25.37 |
27.99 |
30.04 |
4.22 |
4.63 |
5.57 |
1.99 |
2.24 |
2.87 |
BIDA-BTDA |
16.06 |
17.29 |
18.43 |
6.99 |
7.98 |
8.87 |
0.98 |
1.26 |
1.42 |
0.55 |
0.74 |
0.86 |
BIDA-ODPA |
16.99 |
18.29 |
19.45 |
7.74 |
8.85 |
9.68 |
1.45 |
1.82 |
2.05 |
0.67 |
0.81 |
1.05 |
Table 6 Gas diffusivity coefficients (D × 108 in cm2 s−1) at different temperatures through the poly(ether imide) membranesa
Polymer |
Diffusion coefficients (D × 108 in cm2 s−1) |
CO2 |
O2 |
N2 |
CH4 |
35 °C |
45 °C |
55 °C |
35 °C |
45 °C |
55 °C |
35 °C |
45 °C |
55 °C |
35 °C |
45 °C |
55 °C |
The possible errors for the diffusivity measurements for CO2 and O2 were within ±5% whereas for CH4 and N2 errors were in the range ±10%.
|
BIDA-BPADA |
2.54 |
3.40 |
4.25 |
11.44 |
12.93 |
15.19 |
1.95 |
2.66 |
3.18 |
1.64 |
2.36 |
3.00 |
BIDA-6FDA |
3.28 |
4.36 |
5.34 |
14.00 |
15.60 |
17.70 |
3.32 |
4.36 |
5.23 |
1.48 |
2.19 |
2.76 |
BIDA-BTDA |
1.26 |
1.64 |
2.27 |
6.30 |
7.40 |
9.17 |
1.59 |
2.21 |
2.68 |
1.54 |
1.98 |
2.84 |
BIDA-ODPA |
1.85 |
2.53 |
3.21 |
5.87 |
7.20 |
8.65 |
1.93 |
2.65 |
3.16 |
1.63 |
2.41 |
2.96 |
Within a temperature range in which no significant thermal transitions of the polymer occurs, the temperature dependence of diffusivity and permeability can be expressed by the Arrhenius equations:
|  | (7) |
where, ‘
P0’ and ‘
D0’ are the pre-exponential coefficients; ‘
ED’ and ‘
EP’ are apparent activation energies for the diffusion and permeation processes, respectively;
‘R’ is the gas constant; and
‘T’ is the absolute temperature. The ‘
lnP’ and ‘
lnD’ values at different temperatures were plotted against 1/
T to calculate the activation energies for permeation and diffusion processes of these four gases through these four PEI membranes. The activation energies for both the diffusion and permeation processes have been calculated and tabulated in
Table 7. The trend of activation energies for the permeation process for all the PEIs,
EP (CO
2) <
EP (O
2) <
EP (N
2) <
EP (CH
4) was in good agreement with their permeability coefficient order:
P (CO
2) >
P (O
2) >
P (N
2) >
P (CH
4). Similarly, the trend of activation energies for the diffusion processes,
ED (O
2) <
ED (N
2) <
ED (CO
2) <
ED (CH
4) was also in good agreement with their diffusion coefficient values
D (O
2) >
D (N
2) >
D (CO
2) >
D (CH
4) for all the PEIs except BIDA-BPADA. For BIDA-BPADA, the order of activation energy for diffusion process,
ED (O
2) <
ED (N
2) <
ED (CO
2) <
ED (CH
4) is not matching with its trend of diffusion coefficients
D (O
2) >
D (CO
2) >
D (N
2) >
D (CH
4). The diffusion processes are more affected by the physical interaction present within the polymer matrix since the diffusion of the gas molecules through the polymer matrices are directly related to the spacing in the polymer matrix molecules which is governed by the chemical functionalities of the polymer backbones.
17,19 Higher diffusion of CO
2 than that of N
2 through BIDA-BPADA is probably due to the availability of relatively more free space within this polymer matrix in comparison to other polymers in the series. More free space in case of BIDA-BPADA is probably generated from less inter-polyimide chain attraction due to the presence of low polar and bulky aliphatic isopropylidene unit in the main chain which, in turn, favors higher diffusion of CO
2 than N
2 through this polymer. The activation energy values can be of interest per se as physicochemical characteristics of energy barriers in mass transport of small molecules through a polymeric matrix. Practical importance of these parameters arises from the fact that they are needed to calculate the gas permeation and diffusion coefficients at a temperature of interest. The representative plots for ln
P (CO
2)
vs. 1/
T of these four poly(ether imide)s are shown in
Fig. 10.
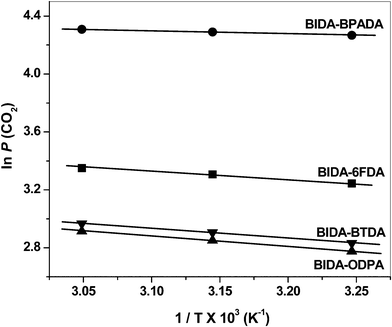 |
| Fig. 10 Representative plots of ln P (CO2) vs. 1/T of the PEIs. | |
Table 7 Activation energies (kJ mol−1) for the diffusion and permeation processes of the gases through poly(ether imide) membranes
Polymer |
CO2 |
O2 |
N2 |
CH4 |
E
D
|
E
P
|
E
D
|
E
P
|
E
D
|
E
P
|
E
D
|
E
P
|
BIDA-BPADA |
21.64 |
4.42 |
11.89 |
8.99 |
20.59 |
15.08 |
25.41 |
18.51 |
BIDA-6FDA |
20.50 |
1.72 |
9.84 |
7.11 |
19.11 |
11.68 |
26.23 |
15.42 |
BIDA-BTDA |
24.76 |
5.79 |
15.74 |
9.98 |
21.93 |
15.86 |
25.65 |
19.05 |
BIDA-ODPA |
23.17 |
5.68 |
16.29 |
9.39 |
20.76 |
14.79 |
25.13 |
18.78 |
Conclusions
A new diamine monomer, 4,9-bis-[4-(4'-amino-3-trifluoromethyl-biphenyl-4-yloxy)-phenyl]-2-phenyl-benzo[f]-isoindole-1,3-dione was synthesized that led to a series of organosoluble PEIs when reacted with various fluorinated and non-fluorinated aromatic dianhydrides. The rationale behind the incorporation of a bulky benzoisoindoledione moiety in the polyimide main chain is to increase the glass transition temperature and thermal stability of the polymers, and at the same time to increase the gas permeability due to its larger volume that prevents the close packing of the polymer chain. Correspondingly, fluorine in the form of a trifluoromethyl group was incorporated to enhance the solution processability of the polyimides. Introduction of the rigid and bulky benzoisoindoledione moiety into the polymer backbone dramatically enhances the processability as well as several physical properties of the polyimides. All the polymers exhibited excellent thermo-oxidative stability (up to 559 °C for 5% wt loss and 592 °C for 10% wt loss temperature) and very high glass transition temperatures (up to 335 °C). The membranes showed good tensile strengths (up to 101.2 MPa) except PMDA based highly rigid BIDA-PMDA (brittle in nature). High thermal stability, high glass transition temperature and high tensile strength of all these polymers (except BIDA-PMDA) render them as suitable candidates for gas transport measurements. The gas transport study of these polymeric membranes has been successfully investigated at three different temperatures (35, 45 and 55 °C) with an applied upstream pressure of 3.5 atm. The gas transport study revealed that the introduction of bulky and rigid aromatic content along with pendant trifluoromethyl groups into the main chain remarkably improved the gas permeability in comparison to the previously reported closely structured poy(ether imide)s based on phthalimidine. The BIDA-6FDA containing hexafluoroisopropylidene (6-FDA) unit showed the highest permeability coefficient for all the gases (PCO2 = 71.3 Barrer, PO2 = 25.4 Barrer) whereas BIDA-BPADA exhibited the highest permselectivity towards CO2 relative to CH4 (37.2) and towards O2 over N2 (8.4) among the series. All the membranes exhibited excellent separation performance for O2/N2 gas pair reaching closer to the latest upper boundary limit drawn by Robeson. BIDA-BPADA surpassed the latest boundary limit for O2/N2 gas pair. Temperature dependencies of the permeation and diffusion processes were utilized to calculate the activation energies of the permeation and diffusion processes for these four different gases through these PEI membranes.
Acknowledgements
The authors thank Department of Science and Technology (DST), India for financial support as project sponsor (Grant No. SR/S3/ME/020/2006-SERC-Eng) for this work. We thank AvH Foundation for donation of the GPC instrument used in this work.
References
-
M. K. Ghosh and K. L. Mittal, in Polyimides: Fundamentals and Application, Marcel Dekker, Inc., New York, 1996.
- J. Fang, X. Guo, S. Harada, T. Watari, K. Tanaka, H. Kita and K. I. Okamoto, Macromolecules, 2002, 35, 9022 CrossRef CAS.
- J. J. Ge, C. Y. Li, G. Xue, I. K. Mann, D. Zhang, S. Y. Wang, F. W. Harris, S. Z. D. Cheng, S. C. Hong, X. Zhuang and Y. R. Shen, J. Am. Chem. Soc., 2001, 123, 5768 CrossRef CAS.
- S. W. Lee, S. J. Lee, S. G. Hahm, T. J. Lee, B. Lee, B. Chae, S. B. Kim, J. C. Jung, W. C. Zin, B. H. Sohn and M. Ree, Macromolecules, 2005, 38, 4331 CrossRef CAS.
- M. G. Dhara and S. Banerjee, Prog. Polym. Sci., 2010, 35, 1022 CrossRef CAS.
- Y. Xiao, B. T. Low, S. S. Hosseini, T. S. Chung and D. R. Paul, Prog. Polym. Sci., 2009, 34, 561 CrossRef CAS.
-
N. N. Li, A. G. Fane, W. S. W. Ho and T. Matsuura, in Advanced Membrane Technology and Applications, John Wiley & Sons, Inc., New Jersey, 2008 Search PubMed.
- J. Fang, H. Kita and K. I. Okamoto, Macromolecules, 2000, 33, 4639 CrossRef CAS.
- M. A. Priolo, D. Gamboa, K. M. Holder and J. C. Grunlan, Nano Lett., 2010, 10, 4970 CrossRef CAS.
- M. A. Priolo, D. Gamboa and J. C. Grunlan, ACS Appl. Mater. Interfaces, 2010, 2, 312 CAS.
- A. J. Svagan, A. Åkesson, M. Cárdenas, S. Bulut, J. C. Knudsen, J. Risbo and D. Plackett, Biomacromolecules, 2012, 13, 397 CrossRef CAS.
- D. Lee, M. C. Choi and C. S. Ha, J. Polym. Sci., Part A: Polym. Chem., 2012, 50, 1611 CrossRef CAS.
- G. Laufer, C. Kirkland, A. A. Cain and J. C. Grunlan, ACS Appl. Mater. Interfaces, 2012, 4, 1643 CAS.
- R. Auras, B. Harte and S. Selke, J. Appl. Polym. Sci., 2004, 92, 1790 CrossRef CAS.
- A. P. Roberts, B. M. Henry, A. P. Sutton, C. R. M. Grovenor, G. A. D. Briggs, T. Miyamoto, M. Kano, Y. Tsukahara and M. Yanaka, J. Membr. Sci., 2002, 208, 75 CrossRef CAS.
- Y. H. Yang, M. Haile, Y. T. Park, F. A. Malek and J. C. Grunlan, Macromolecules, 2011, 44, 1450 CAS.
-
H. Ohya, V. V. Kudryavtsev and S. I. Semenova, in Polyimide membranes: applications, fabrications and properties, Gordon and Breach Publishers, Tokyo, 1996 Search PubMed.
- S. A. Stern, J. Membr. Sci., 1994, 94, 1 CrossRef CAS.
- M. Calle, A. E. Lozano, J. G. de La Campa and J. de Abajo, Macromolecules, 2010, 43, 2268 CrossRef CAS.
-
S. Banerjee, S. K. Sen, A. Ghosh and B. Dasgupta, In Polymer Synthesis, Chapter 4, Nova Science Publishers, Inc., New York, 2011 Search PubMed.
- J. Yan, Z. Wang, L. Gao and M. Ding, Macromolecules, 2006, 39, 7555 CrossRef CAS.
- S. Yoshida and A. S. Hay, Macromolecules, 1997, 30, 5979 CrossRef CAS.
-
S. Ando, T. Matsuura and S. Sasaki, In Fluoropolymers 2. Properties, Chapter 14. Plenum Publishers, New York, 1999 Search PubMed.
- M. R. Coleman and W. J. Koros, J. Membr. Sci., 1990, 50, 285 CrossRef CAS.
- K. Tanaka, H. Kita, M. Okano and K. I. Okamoto, Polymer, 1992, 33, 585 CrossRef CAS.
- J. H. Kim, S. B. Lee and S. Y. Kim, J. Appl. Polym. Sci., 2000, 77, 2756 CrossRef CAS.
- K. Tanaka, H. Kita and K. I. Okamoto, J. Polym. Sci., Part B: Polym. Phys., 1993, 31, 1127 CrossRef CAS.
- I. V. Farr, D. Kratzner, T. E. Glass, D. Dunson, Q. Ji and J. E. McGrath, J. Polym. Sci., Part A: Polym. Chem., 2000, 38, 2840 CrossRef CAS.
- E. M. Maya, I. García-Yoldi, A. E. Lozano, J. G. de la Campa and J. de Abajo, Macromolecules, 2011, 44, 2780 CrossRef CAS.
- Y. H. Kim, H. S. Kim and S. K. Kwon, Macromolecules, 2005, 38, 7950 CrossRef CAS.
- S. K. Sen and S. Banerjee, J. Membr. Sci., 2010, 350, 53 CrossRef CAS.
- L. M. Robeson, J. Membr. Sci., 2008, 320, 390 CrossRef CAS.
- M. Al-Masri, D. Fritsch and H. R. Kricheldorf, Macromolecules, 2000, 33, 7127 CrossRef CAS.
- H. S. Kim, Y. H. Kim, S. K. Ahn and S. K. Kwon, Macromolecules, 2003, 36, 2327 CrossRef CAS.
- Z. Wang, T. Chen and J. Xu, Macromolecules, 2007, 40, 3238 CrossRef CAS.
- G. Chen, X. Zhang, S. Zhang, T. Chen and Y. Wu, J. Appl. Polym. Sci., 2007, 106, 2808 CrossRef CAS.
- S. K. Sen, B. Dasgupta and S. Banerjee, J. Membr. Sci., 2009, 343, 97 CrossRef CAS.
- Y. Meng, I. A. Abu-Yousef, A. R. Hlil and A. S. Hay, Macromolecules, 2000, 33, 9185 CrossRef CAS.
- J. Y. Park and D. R. Paul, J. Membr. Sci., 1997, 125, 23 CrossRef CAS.
- M. Strukelj and A. S. Hay, Macromolecules, 1992, 25, 4721 CrossRef CAS.
- V. Kute and S. Banerjee, Macromol. Chem. Phys., 2003, 204, 2105 CrossRef CAS.
- W. J. Koros and G. K. Fleming, J. Membr. Sci., 1993, 83, 1 CrossRef CAS.
- G. Maier, Angew. Chem., Int. Ed., 1998, 37, 2960 CrossRef.
|
This journal is © The Royal Society of Chemistry 2012 |
Click here to see how this site uses Cookies. View our privacy policy here.