DOI:
10.1039/C2RA20265D
(Paper)
RSC Adv., 2012,
2, 6854-6870
Structure-property interplay of poly(amide-imide) and TiO2 nanoparticles impregnated poly(ether-sulfone) asymmetric nanofiltration membranes
Received
14th February 2012
, Accepted 18th May 2012
First published on 21st May 2012
Abstract
Poly(amide-imide) (PAI) and TiO2 nanoparticle-incorporated poly(ether-sulfone) (PES) asymmetric nanofiltration membranes with an integrally dense skin layer were prepared by diffusion induced phase separation. The prepared membranes were characterized by attenuated total reflectance-Fourier transform infrared spectroscopy (ATR-FTIR), X-ray diffraction (XRD), differential scanning calorimetry (DSC), scanning electron microscopy (SEM), atomic force microscopy (AFM), pure water flux, water content and contact angle technique to investigate the influence of PAI on the properties of the membranes. Intermolecular interactions between the components in blend membranes were established by ATR-FTIR and microcrystalline morphology was confirmed by XRD. The SEM analysis showed that blend membranes have an integrally dense skin layer adequate for nanofiltration and a spongy sub-layer along the entire membrane cross section. The contact angle measurements indicated that, hydrophilicity of the virgin PES membranes was improved by the addition of PAI and TiO2 nanoparticles due to the preferential orientation of these components towards the membrane surface during immersion precipitation. Surface free energy parameters of the membrane such as surface free energy, interfacial free energy, work of adhesion and spreading coefficient were calculated. The efficiency of these membranes in the separation of mixture solutions of divalent salt and surfactant were found to be improved significantly. Fouling stability of the membranes studied by bovine serum albumin (BSA) as the model foulant revealed improved fouling resistance. Chlorine stability of the modified membranes was also investigated. From the results, it was revealed that low interfacial free energy membranes prepared by the incorporation of PAI and TiO2 nanoparticles may be valuable in fouling stability industrial separations.
1. Introduction
Nanofiltration (NF) is a pressure-driven membrane separation process widely used for the drinking water purification, environmental protection, and in the textile, pharmaceutical, food and paper industries. This technique has been considered as a reliable technique over conventional separation processes and reverse osmosis (RO) because of its advantages such as low operational pressure, high flux and affordable maintenance cost.1,2 However the effective implementation of nanofiltration for the separation and purification of liquid mixtures is limited because of fouling.3 Membrane fouling, caused by the deposition of particles on the membrane surface, often causes a significant increase in hydraulic resistance and applied pressure drop, which increases operating cost and decreases the life of the membrane. Current research and development efforts are directed towards drastic improvements in selectivity while maintaining the inherent high-throughput characteristics of the membranes.4,5
Many researchers have demonstrated that, an increase in hydrophilicity of membranes significantly reduces membrane fouling as result of reduced hydrophobic interaction with the foulant.6,7 Various methods, such as polymer blending, nanoparticle incorporation, grafting, interfacial polymerization and atom transfer radical polymerization, have been used to improve the membrane performance and hydrophilicity.8 However, copolymer synthesis by grafting methods suffers from drawbacks such as highly expensive complicated equipment and strict operation, and these methods are often not suitable for using organic solvents as the reaction medium.9 The interfacial polymerization technique is quite complex and can only be carried out in two different bath solutions (aqueous and organic solution) and requires sophisticated preparation conditions.1,8 Therefore, it would be wise to give priority to the first two methods for membrane modification since it is an extremely attractive and inexpensive way of obtaining new structural materials.
Poly(ether-sulfone) (PES) is widely used as a commercial membrane material since it displays excellent mechanical, thermal and chemical resistance as well as outstanding film forming properties. However, the application of PES as a membrane material is severely limited by its hydrophobic nature, which necessitates modification before membrane preparation.10,11 On the other hand, aromatic poly(amide-imide) (PAI), an amorphous thermoplastic, appears to be of particular interest as a membrane material because of its remarkable physical, chemical properties and highly hydrophilic nature. Rahimpour et al. synthesized poly(amide-imide) containing oligoether spacers and blended them with PES for the development of ultrafiltration membranes for milk protein recovery.11 In our previous work, poly(amide-imide) was incorporated into a cellulose acetate matrix for fabricating fouling-resistant membranes for industrial separations and was found to be successful.12
Over the past few years, attractive membrane materials have been developed by mixing both polymers and ceramic materials, by dispersing an inorganic filler in a polymeric matrix.13 Composite membranes prepared by the incorporation of these nanoparticles showed exceptional performance characteristics such as high permeation rate, antifouling properties, thermal stability, and mechanical properties.13–15 Among the mostly used inorganic nanoparticles, nano-sized titanium dioxide (TiO2) is of special interest because of its high hydrophilicity, good chemical stability, thermal stability and antibacterial properties. Anatase TiO2 could also be used as a photo catalyst in waste water treatment.15 This is the first attempt in the literature that explores the usage of hydrophilic poly(amide-imide) and TiO2 in the modification of PES membranes for nanofiltration applications.
The objective of the present study was to develop low surface energy, high permeable and antifouling PES nanofiltration membranes by incorporating PAI and TiO2 nanoparticles into the casting solution by phase inversion technique. Attenuated total reflectance-Fourier transform infrared spectroscopy (ATR-FTIR) and X-ray diffraction (XRD) were used to characterize the intermolecular interactions between the components and crystalline structure of the membranes respectively. The morphology of the membranes was studied by scanning electron microscopy (SEM) and atomic force microscopy (AFM). Compatibility between the PES and PAI in the blend membranes was studied by differential scanning calorimetric analysis. The effect of polymer blend composition and TiO2 on the membrane morphology, compaction, pure water flux, water content and membrane hydrophilicity were investigated. Fouling resistance of the membranes was evaluated by BSA as the model foulant and chlorine stability of the membranes was also studied. An attempt has been made to correlate the changes in membrane morphology with compaction, water flux, water content and efficiency of the membranes in the separation of a solution containing salt and surfactant and the results are discussed.
2. Experimental section
2.1. Materials and methods
Commercial grade poly(ether-sulfone) Gafone-3300 (Tg-219 °C), obtained as a gift sample, from Gharda Chemicals Pvt Ltd., India, was used after drying under vacuum for 8 h. Commercial grade poly(amide-imide), TorlonRM 4000T-HV (Tg-280 °C) supplied as a gift sample by Solvay Advanced Polymers, L.L.C., USA, was used after drying under vacuum for 8 h.
Anhydrous titanium tetrachloride procured from Spectrochem Ltd., India, was used as the starting material for the synthesis of titanium dioxide nanoparticles. Analar grade N-methyl-2-pyrrolidone (NMP) and 1,4-dioxane from SRL Chemicals Ltd., India, was sieved through molecular sieves (type 5 Å) to remove moisture and stored in dry conditions prior to use. Sodium lauryl sulfate (SLS) of analar grade was obtained from Qualigens Fine Chemicals Ltd., India, and was used as a surfactant in the coagulation bath. Distilled water was employed for the nanofiltration experiments and for the preparation of the gelation bath. Anhydrous MgSO4, MgCl2, Na2SO4, and NaCl of analytical grade were purchased from SRL Chemicals Ltd., India, and used for the rejection studies. Bovine serum albumin (BSA, Mw = 69 kDa) was purchased from SRL Chemicals Ltd., India and sodium hypochlorite solution, 6–10% w/v from Sigma Aldrich was used for the membrane stability study.
Nanocrystalline TiO2 powders were prepared by a solution based processing method by controlling the hydrolysis of TiCl4 in aqueous solution. Titanium tetrachloride aqueous solution of 3 M concentration was prepared by mixing with distilled water in ice-water bath. The aqueous solution was then mixed with (NH4)2 SO4 solution at 70 °C at high speed, while the amount of TiCl4 solution necessary for the desired [Ti]:[H2O] molar ratio (1
:
25) was maintained. Maintaining the hydrolysis temperature for 1 h, the mixed solution was treated with 2.5 M dilute NH4OH until the pH value was 7. The reaction conditions were maintained for a period of 3 h, during which the reaction was assumed to have attained equilibrium. The preparation conditions have been described in detail elsewhere.16,17 Subsequently, the precipitated TiO2 was separated from the solution by filtration and repeatedly washed with distilled water and NH4OH solution to make the TiO2 free of chloride ions.16,17
2.3. Blend membrane preparation
The blend solutions based on PES and PAI were prepared by dissolving the two polymers of different composition in NMP as the solvent and 1,4-dioxane as co-solvent under constant mechanical stirring in a round-bottom flask for 8 h. A series of such polymer solutions were prepared by varying the composition of PES and PAI in the absence and presence of titanium dioxide nanoparticles, as shown in Table 1. The homogeneous solution thus obtained was allowed to stand at room temperature for at least 6 h in an airtight condition to get rid of air bubbles. In order to reduce the pore size of the membrane, the “phase inversion” method employed in our earlier works for the preparation of ultrafiltration membranes was modified accordingly.12,18 The casting environment, namely, relative humidity (12 ± 2%) and temperature (40 ± 2 °C), was changed from our earlier method and maintained for the preparation of membranes with better physical properties such as homogeneity, thickness, and morphology. In order to avoid the precipitation of the polymer solution by the atmospheric moisture, relative humidity values were always kept less than 15%. The thickness of the membranes was maintained at 0.20 ± 0.02 mm and measured with a micrometer having a precision of 0.001 mm.
The gelation conditions were changed from our earlier method and maintained constant throughout, since the thermodynamic conditions largely affect the morphology and performance of the resulting membranes. Prior to casting, a 2 L gelation bath, consisting of 4% (v/v) NMP, 1,4-dioxane and 0.2 wt % surfactant, SLS in distilled water, was prepared and kept at 5 ± 2 °C. Just prior to casting, the homogeneous polymer solution was mechanically stirred for 5 min and subsequently sonicated for 3 min to avoid agglomeration of nanoparticles. The membranes were cast over a glass plate using a doctor blade. After casting, the solvent present in the cast film was allowed to evaporate for 90 s, and the cast film along with the glass plate was gently immersed in the gelation bath. After 2 h of gelation, the membranes were removed from the gelation bath and washed thoroughly with distilled water to remove all solvents and surfactant from the membranes. The membrane sheets were subsequently stored in distilled water, containing 0.1% formalin solution to prevent microbial growth.
Table 1 Composition and casting conditions of PES–PAI blend membranesa
Blend composition |
TiO2 nanoparticles (wt %) |
Solvent (wt %) |
Poly(ether-sulfone) (wt %) |
Poly(amide-imide) (wt %) |
NMP |
1,4-Dioxane |
Casting temperature = 40 ± 2 °C; casting relative humidity = 12 ± 2%; solvent evaporation time = 90 s. Note: total polymer concentration = 20 wt%, total solvent concentration = 80 wt%.
|
100 |
0 |
0 |
70 |
30 |
90 |
10 |
0 |
70 |
30 |
80 |
20 |
0 |
70 |
30 |
70 |
30 |
0 |
70 |
30 |
99 |
0 |
1 |
70 |
30 |
89 |
10 |
1 |
70 |
30 |
79 |
20 |
1 |
70 |
30 |
69 |
30 |
1 |
70 |
30 |
The NF experiments were performed using a cross-flow filtration setup in which four membrane cells were connected in series. The cross-flow cell houses flat sheet circular membrane pieces with an effective area of 19.6 cm2. A centrifugal pump was used to pass the feed solution through the membrane surface at a flow rate of 1.4 L min−1 and the flow was assumed as turbulent. The valve located at the end of the cell was used to pressurize the feed solutions and to control the feed pressure. The flow diagram of the NF experimental setup is given in Fig. 1. All the experiments were carried out at 30 ± 2 °C and compaction at 2070 kPa transmembrane pressure, while pure water flux, rejection and fouling studies were carried at a transmembrane pressure of 1725 kPa.
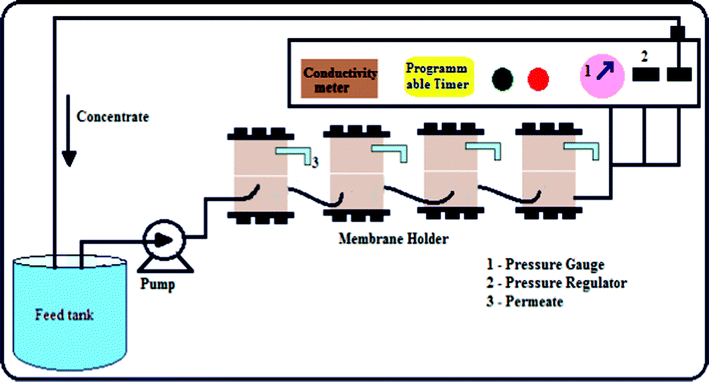 |
| Fig. 1 Schematic representation of the nanofiltration experimental set up. | |
2.5. Membrane characterization
2.5.2. FTIR analysis.
FTIR spectra of pure PES and PES–PAI blend membranes were recorded using an attenuated total reflectance (ATR) technique with a spectrometer (Thermo Nicolet, Avatar 370) in the range 4000–500 cm−1. The IR spectrum for the pure PAI powder was obtained by the KBr pellet method.
2.5.3. X-ray diffraction (XRD) analysis.
Wide angle X-ray diffraction (WAXD) patterns of the pure PES, PES–PAI blend membranes and PAI powder were recorded using a Bruker AXS D8 Advance X-ray diffractometer. The diffractograms were measured at diffraction angle 2θ in the range 5–50° using Cu-Kα radiation (λ = 1.5406 Å). A tube voltage of 40 kV and tube current of 30 mA were kept constant throughout the experiment.
2.5.4 Differential scanning calorimetric analysis.
Differential scanning calorimetric (DSC) analysis of the prepared membranes (approximately 6 mg each) were performed on a Universal V4.5A TA thermal analyzer in a nitrogen atmosphere. Glass transition temperature and compatibility of the components in blend membranes were studied by heating the membranes from room temperature to 300 °C at a heating rate of 10 °C min−1.
2.5.5. Scanning electron microscopy (SEM) analysis.
The cross sectional images of the PES and PES–PAI blend membranes were taken by scanning electron microscopy (SEM, Cam Scan MV2300). The membranes were cut into small pieces and mopped with blotting paper. The pieces were immersed in liquid nitrogen for 60–90 s and were frozen. These frozen fragments of the membranes were stored in a desiccator. The dried samples were gold sputtered for producing electric conductivity and photographs were taken in very high vacuum conditions operating at 10 kV.
2.5.6. Atomic force microscopy (AFM) analysis.
Atomic force microscopy was used to analyze the surface morphology and roughness of the prepared membranes (AFM device was Dual Scope™ scanning probe-optical microscope, DME model C-21, Denmark). Small squares of the prepared membranes (approximately 1 cm2) were cut and glued on a glass substrate. The membrane surfaces were imaged in a scan size of 2 μm × 2 μm and the surface roughness was measured by the tapping mode, since contact mode AFM cannot be applied to polymeric membranes because of the excessive tracking forces applied to the sample by the probe.
2.5.7. Membrane compaction.
The hydraulic compaction of fresh membranes was carried out by loading the membranes, of necessary size, in the nanofiltration test cell and filtering water at a pressure of 2070 kPa. The water flux was measured every 10 min till it leveled off after 30–40 min. The pure water flux (Jw) and compaction factor was determined as follows:19 |  | (1) |
where Jw is the water flux (in L m−2 h−1), Q is the quantity of permeate collected (in L), Δt is the sampling time (in h), and A is the membrane area (in m2). |  | (2) |
2.5.8. Pure water flux.
Membranes after compaction were subjected to a trans-membrane pressure of 1725 kPa to measure the pure water flux. The pure water flux (PWF) was measured after a stabilization of period of 15 min and PWF was calculated under steady state conditions using eqn (1). The pure water flux was measured three times and the average was reported for accuracy.
2.5.9. Equilibrium water content.
Equilibrium water content of the membranes was obtained by soaking the membranes in water for 24 h and weighing them after mopping with blotting paper. These wet membranes were placed in a vacuum oven at 50 °C for 48 h and the dry weights were determined. From these two values, the water content is calculated and an average of five measurements is reported: | 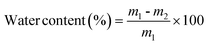 | (3) |
where m1 is the weight of the wet membrane and m2 is the weight of the dry membrane.
2.5.10. Measurement of contact angle and surface free energy.
Contact angle measurements of water on the wet membrane surfaces were carried out by the sessile drop method at ambient temperature using a goniometer (GBX Instruments, Germany). Membranes, of sample size 3 × 3 cm2, were washed thoroughly with water and mopped with blotting paper to remove the moisture content prior to the experiment. The sessile drop was formed on the membrane surface by depositing 5 μL of milli-Q water steadily on to the membrane surface with a microsyringe and contact angle was measured within 30 s of the addition of the water drop. For each sample, measurements were performed in six different locations and an average was considered for accuracy.
Theory and calculation.
The importance of contact angle is that they contain information about the surface tension of the solid surfaces through Young's equation:20where γlv is the liquid–vapour, γsv the solid–vapour and γsl the solid–liquid interfacial free energies respectively and θ is the measured angle with respect to the surface, as illustrated schematically in Fig. 2.
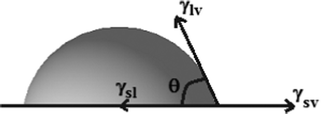 |
| Fig. 2 Schematic representation of contact angle measurement and various interactions during measurement. | |
The calculation of interfacial free energy, γsl from the contact angle of a liquid of surface tension γlv starts with the Young's equation, eqn (4). Of the four quantities in the Young's equation, only γlv and θ are readily measurable. Thus, in order to determine γsl and γsv, further information is necessary. Conceptually, an obvious approach is to seek one more relation among the parameters of eqn (4), such as an equation-of-state relation, of the form
The simultaneous solution of
eqn (4) and
(4a) would solve the problem.
In order to calculate the surface free energy of the solid, Kwok and Neumann20 modified the Berthelot rule and put forward an equation-of-state relation of the form of eqn (4a),
| 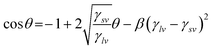 | (4b) |
This enables the calculation of the solid–vapour surface tension, γsv, from a single contact angle measurement with a liquid of known surface tension. According to Neumann such an equation-of-state in conjunction with Young's equation is acceptable only when the results go with that obtained using another equation of state. An alternative formulation of Berthelot's rule, put forward by Neumann was,
| 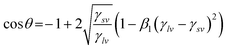 | (4c) |
The eqn (4c) has been tested along with Young's equation for the calculation of γsv and was found to be in good agreement with the results of eqn (4b). This establishes the use of either of the proposed equations-of-state in the calculation of solid–vapour surface tension, γsv. Thus, the solid–vapour surface tension can be determined when experimental (Young's) contact angle and liquid–vapour surface tension are known. The constants β and β1 used in the above eqn (4b) and (4c) are based on a constant value of 0.0001024 (m2 mJ−1)2 and 0.0001054 (m2 mJ−1)2 respectively. Thus the interfacial free energy, γsl, of the membranes can be calculated from these γsv values by the Young's equation given in eqn (4).
Thermodynamically, work of adhesion, Wa, is the work required per unit area, to separate the liquid from the membrane leaving an adsorbed film in equilibrium with the surface of membranes and is calculated by,
The spreading coefficient, Sc is the work done in spreading the liquid over a unit area of surface.
2.5.11. Rejection studies.
The salt rejection property of the membranes was studied by filtering MgSO4, MgCl2, Na2SO4, and NaCl electrolyte solutions at an initial concentration of 1000 ppm. Surfactant sodium lauryl sulfate, 0.1 mM concentration, was added to each electrolyte solution in order to reflect the composition of waste water in the textile and dye industries. The feed solution pH was adjusted to 4 and the feed solution was filtered individually at an operating pressure of 1725 kPa. The salt concentration in permeate solutions collected at different time intervals was measured by a conductivity meter connected to the nanofiltration unit. The percentage rejection of each salt was measured four times and calculated by the equation | 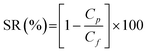 | (5) |
2.5.12. Fouling analysis.
0.1 wt% BSA solution was prepared in 0.5 M phosphate buffer solution (pH 4.0) and used as the feed solution for the fouling studies. Each membrane was initially compacted for 15 min and the pure water flux, Jw1 was measured at a transmembrane pressure of 1725 kPa, according to eqn (1). The cell was then emptied and refilled with BSA solution. The initial protein flux, Jp1, and steady state protein solution flux, Jp, after 4 h of filtration was recorded. To evaluate the antifouling property of the membranes, the flux decline rate was calculated by the following equation21 | 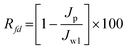 | (6) |
The degree of irreversible flux loss caused by irreversible fouling (Rir) and reversible flux loss caused by reversible fouling (Rr) is calculated using the following eqn (7) and (8), respectively
| 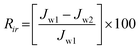 | (7) |
| 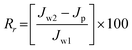 | (8) |
After filtration of the BSA solution for 4 h, the fouled membrane was washed with deionized water. The pure water flux, Jw2, of the cleaned membrane was measured again under the same conditions. The flux recovery ratio (FRR) was calculated using the following equation:21
| 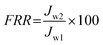 | (9) |
2.5.13. Membrane chlorine stability.
Chlorine stability of the membranes was analyzed by soaking the membranes in commercial sodium hypochlorite solution for 3 h at constant pH 4.0. The chlorinate solution with concentrations of 1000 and 2000 ppm were prepared by diluting sodium hypochlorite solution with pure water. The soaking tests were performed in petri dishes with closed lids covered tightly with aluminium foil. After thoroughly rinsing with de-ionized water, membrane stability was evaluated at an operating pressure of 1725 kPa in terms of normalized rejection performance for MgSO4 as described in the earlier section. Normalized rejection was defined as the rejection of chlorinated membranes divided by the rejection of their virgin membranes under same experimental conditions.22,23 Experiments were carried out four times and average was reported.
3. Results and discussion
3.1. Transmission electron microscopic analysis of TiO2
Transmission electron microscopy was used to investigate the particle size and the image is shown in Fig. 3. In this image, TiO2 particles can be seen in the form of black spots and particle size is found to be 20 ± 5 nm. The improvement in properties of the composites filled with nanoparticles depends on the available polymer–filler interfacial surface area, which is affected by both the degree of loading and the size of the filler.15,24 We expect that, 1,4-dioxane present in the casting solution acts as a dispersing agent for TiO2, because of its low solvating power compared to NMP for PES and PAI, which results in a decrease in the size of the aggregates. If the TiO2 loading is increased with the amount of 1,4-dioxane remaining unchanged, the dispersing effect of 1,4-dioxane should weaken, thereby favoring TiO2 aggregation. Thus in order to maintain the casting solution’s de-mixing behavior uniform and to avoid undesirable effects from the agglomeration of TiO2 nanoparticles, we have restricted the total loading to 1 wt%.
FTIR spectra of pure PES, PES–PAI blend membranes and PAI powder were recorded to investigate the intermolecular interactions between PES and PAI in the blend membranes. FTIR spectra obtained for all membranes and PAI powder are presented in Fig. 4. The spectral bands at 1486 cm−1 and at 1069 cm−1 of the pure PES membrane corresponds to the stretching frequencies of –SO2 group and –C–O– group respectively. On the other hand, spectral bands of pure PAI provide a broad band at 3506 cm−1 which corresponds to the stretching frequency of the –NH group in the amide linkage. Another two strong peaks with maxima at 1717 cm−1 and 1652 cm−1 are assigned to the stretching frequencies of the –C
O group of the amide linkage and –C
O group in the five membered ring of PAI respectively.
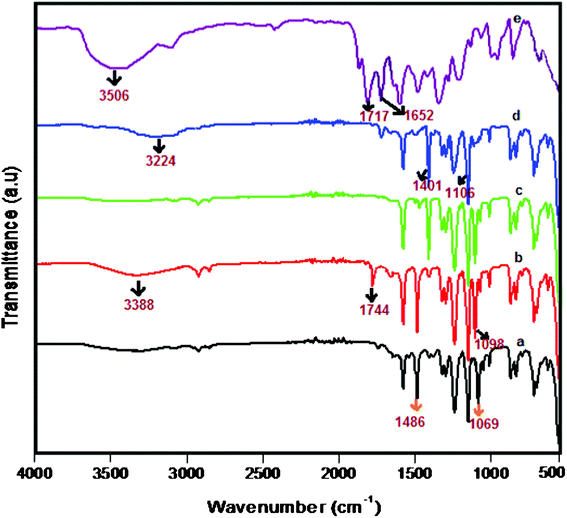 |
| Fig. 4 ATR-FTIR spectra of PES–PAI blend membranes (w/w) and pure PAI powder: (a) pure PES (b) PES–PAI (80/20) (c) PES–TiO2 (99/1) (d) PES–PAI–TiO2 (79/20/1) (e) pure PAI. | |
The spectra of PES–PAI blend membranes indicated that sharp band at 1069 cm−1 of pure PES is shifted to 1098 cm−1. Further, the –NH group stretching frequency at 3506 cm−1 and –C
O stretching frequency at 1717 cm−1 of pure PAI is shifted to 3388 cm−1 and 1744 cm−1, respectively. Whereas the stretching frequencies of the –C
O group at 1652 cm−1 of PAI disappeared and –SO2 stretching frequency of PES at 1486 cm−1 is unaltered. This shift in the stretching frequencies of PES–PAI blend membranes confirms the establishment of the hydrogen bond between the amide group of PAI and –C–O group of PES. The occurrence of such interactions is initiated by the transfer of a lone pair of electrons present in the oxygen of –C–O– group of PES to the amide of PAI. The possible interaction and the established hydrogen bond between PES and PAI in blend membranes are schematically represented in Fig. 5. The presence of such –N
H⋯O–C– interactions implied a good miscibility of PES and PAI in the blend membranes.25 In PES–PAI–TiO2 composite membranes sharp bands at 1486 cm−1 and at 1069 cm−1 of pure PES are shifted to 1401 cm−1 and 1106 cm−1 respectively. However, broad band appeared at 3388 cm−1 of the PES–PAI blend membrane shifted to 3224 cm−1 and the –C
O stretching frequency at 1717 cm−1 disappeared indicating a complex interaction between PES and PAI through TiO2 nanoparticles. The disappearance of peaks corresponding to C
O stretching in PES–PAI–TiO2 nano composite membranes is due to the strong binding of TiO2 to the PES–PAI matrix. From this we can conclude that in PES–PAI–TiO2 composite membranes, hydrogen bonding is facilitated by TiO2 nanoparticles by the migration of electrons to oxygen as represented in Fig. 5.
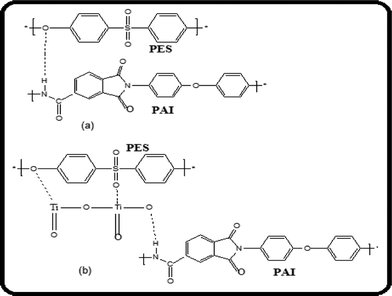 |
| Fig. 5 Schematic representation of the intermolecular interactions between the components in (a) PES–PAI and (b) PES–PAI–TiO2 blend membranes. | |
3.3. XRD-analysis of TiO2 nanoparticles and PES–PAI membranes
The XRD patterns of pure PES, PES–PAI blend membranes, PES–PAI composite membranes, pure PAI powder and TiO2 nanoparticles are shown in Fig. 6. From the XRD spectra it was observed that the pure PES membrane showed two broad semi crystalline peaks at a diffraction angle (2θ) of 19.2° and 29.5°. The PAI powder exhibited a semi crystalline diffraction peak at 11.2° indicating the amorphous nature of PAI. The TiO2 nanoparticles have dominant crystalline peaks at (2θ) of 24.12, 33.52 and 46.71 and a semi crystalline peak at 26.23°.26 No significant change in the XRD spectra could be detected in the PES–PAI blend membranes except an insignificant decrease in the intensity of the peaks of PES. However, PES–PAI nanocomposite membranes displayed XRD patterns that integrated all the diffraction peaks of PES–PAI blend membranes and TiO2. In contrast to the board diffraction peaks for the PES–PAI membranes, the intensity of peaks in the PES–PAI nanocomposites increased slightly and their locations were shifted to lower values compared to that of TiO2 nanoparticles. The XRD results showed that TiO2 nanoparticles remained in the matrix during the membrane formation.
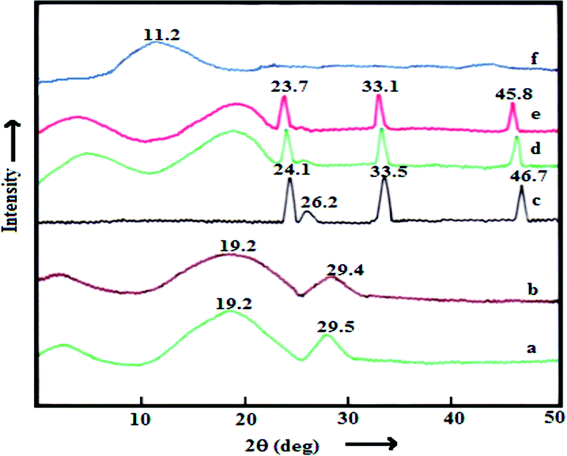 |
| Fig. 6 XRD patterns of the PES–PAI blend membranes (w/w) and pure PAI powder: (a) pure PES (b) PES–PAI (80/20) (c) TiO2 nanoparticles (d) PES–TiO2 (99/1) (e) PES–PAI–TiO2 (79/20/1) and (f) pure PAI powder. | |
3.4. Differential scanning calorimetric studies of PES–PAI blend membranes
The DSC thermograms of the pure PES and PES–PAI blend membranes presented in Fig. 7 were used to study the miscibility behaviour between PES and PAI in blend membranes. PES exhibits a glass transition temperature (Tg) of 218 °C in relatively good agreement with that reported in the literature.27 The Tg values of the blends lie between those of the components in the mixture, precisely at 223, 228.5 and 233.5 °C respectively for PES–PAI blend membranes at 90/10, 80/20 and 70/30 composition. The existence of a single well-defined Tg over the entire blend composition range, demonstrated the compatibility of the PES–PAI blend membranes probably due to the strong interaction between the components. From the DSC studies of the membranes, we can also conclude that the PES–PAI blend membranes have higher glass transition temperature than pure PES membranes. The thermoplastic PAI with Tg of 285 °C greatly contribute to the improvement of Tgs of the polymer blend and it is believed to be due to the formation of hydrogen bonds between the components as shown in ATR-FTIR analysis results.28
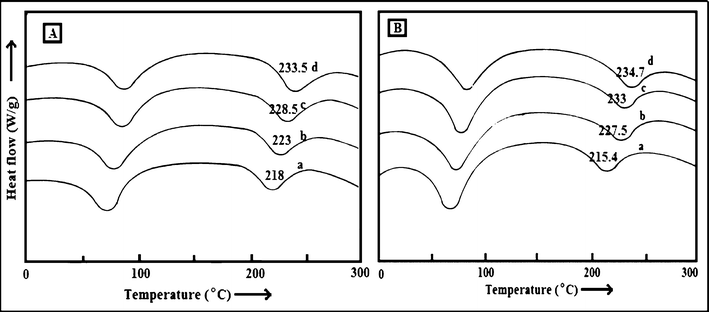 |
| Fig. 7 Differential scanning calorimetric curves of (A) PES–PAI membranes (w/w) (a) 100/0; (b) 90/10; (c) 80/20; (d) 70/30 and (B) PES–PAI–TiO2 (w/w) membranes (a) 99/1; (b) 89/10/1; (c) 79/20/1; (d) 69/30/1. | |
As shown in Fig. 7 (B) incorporation of TiO2 nanoparticles in the PES matrix led to small shift in Tg to lower temperature. However, addition of TiO2 nanoparticles to the PES–PAI binary polymer matrix increased the Tg appreciably due to the stiffening of the segmental motion of the polymer chains. It was expected that smaller TiO2 domains with a larger surface area increased interfacial interactions between TiO2 and the polymer matrix.26 Since the TiO2 nanoparticles are highly hydrophilic and PES is hydrophobic, there was a repulsive interaction resulted in a higher chain mobility and large region of free volume. However, PES–PAI blend membranes show a significant increase in Tg, which was perhaps due to the attractive interaction between inorganic nanoparticles and hydrophilic polymer matrix.
3.5. Scanning electron microscopic analysis of PES–PAI blend membranes
Fig. 8 and 9 show cross sectional SEM images of the membranes prepared from different compositions of PES and PAI in the absence and presence of the TiO2 nanoparticles respectively. From the images it can be seen that, membranes prepared from PES without PAI exhibit a finger like structure over the entire membrane cross-section in distinction to the sponge like structure of the PES–PAI blend membranes and the separating layer was found to be very thin in contrast to the dense skin in the blend membranes. In our method of preparation, evaporation time was 90 s, during which the top surface of the pure PES casting solution was converted from a clear one-phase into a turbid two-phase due to the imbibition of atmospheric water vapor and evaporation of less volatile solvent 1,4-dioxane. Under these conditions, blend polymer films was supersaturated with a layer of water vapor rather than being precipitated due to their high water tolerance compared to the poor water tolerance of pure PES membranes. Thus, due to their high hydrophobicity, the separating layer of pure PES membranes was formed at the very first instant of the coagulation bath-casting dope contact and results in thin surface layer. Upon immersion in the gelation bath, the bound layer of water present in the surface of blend membranes moves down few layers interior and forms an immobilized bound layer on the polymer–water interface. Such a change reflects an increase in the dope solution hydrophilicity with the increasing percentage of PAI content and results in a dense skin layer in blend membranes as evidenced by SEM images. As a result, the non-solvent inflow and solvent outflow from the polymer casting solution were restrained, which has a profound effect in the final structural development of the resultant membranes.29
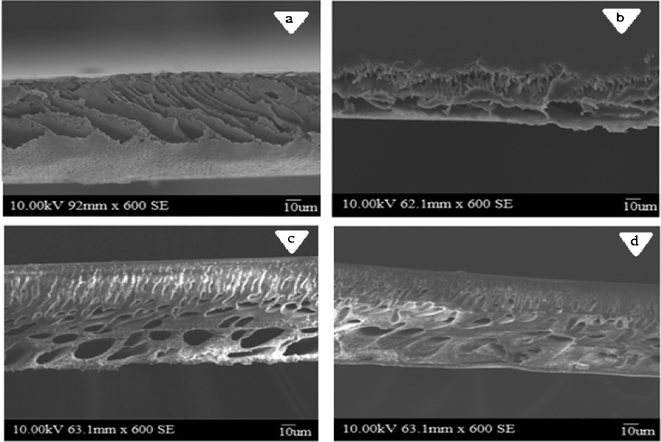 |
| Fig. 8 Cross sectional SEM images of PES–PAI blend membranes (w/w): (a) 100/0; (b) 90/10; (c) 80/20; (d) 70/30. | |
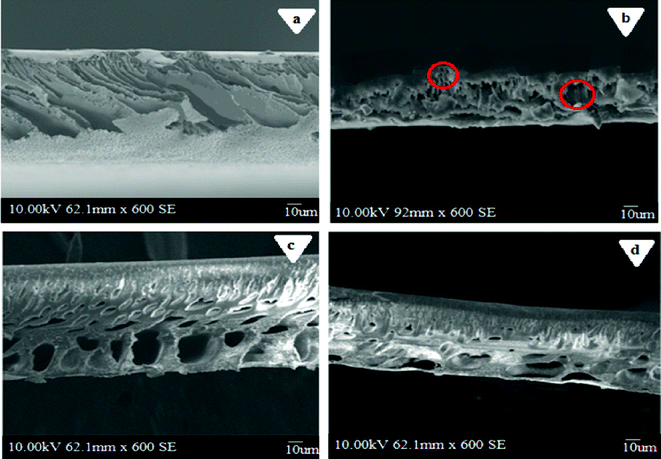 |
| Fig. 9 Cross sectional SEM images of PES–PAI blend membranes (w/w) in the presence of TiO2 nanoparticles: (a) 99/1; (b) 89/10/1; (c) 79/20/1; (d) 69/30/1. | |
In order to reduce the pore size of the membranes to the nanofiltration level, we have restricted the mutual diffusion rate of the solvent and non-solvent by controlling the phase inversion parameters by preparing a doped solution with 1,4-dioxane, which has a low miscibility with water, adding the solvent in the coagulation bath and employing a low bath temperature of 5 ± 2 °C. However, we admit that the rate of inward diffusion of non-solvent and outward diffusion of solvent change significantly with the polymer PAI and it is considered as a vital component in the development of the sponge like structure in the blend membranes. The much greater affinity of PAI to water leads to the longer exchange between the water in the coagulation bath and NMP in the doped solution before the doped solution becomes gelled and vitrified. Experimentally, 3–4 min of coagulation time was needed for the “setting” of the blend membranes, while pure PES membranes set within one minute. The faster solvent exchange through the thin skin of the pure PES membranes favored the rapid growth towards the depth of the polymer film. A spongy sub layer structure with an integrally dense skin layer, required for nanofiltration applications, was difficult to obtain with pure PES membranes, because the diffusion process associated with the redissolution step was speeded up by the relatively faster mutual diffusion through the thin skin formed on the water–polymer interface and highly hydrophobic nature of the polymer. Even though the controlled phase separation enables us to prepare PES membrane with small pore size, we couldn't avoid the formation of finger like pores in the cross section.
The blend membrane morphology differs significantly from that of the pure polymer membranes, indicating that the phase separation proceeded in a different way.30,31 A spongious structure with higher porosity was formed at the bottom part by this slow phase separation in the PAI incorporated polymer solution. Nevertheless, there was no significant difference in the overall membrane morphology between the membranes without and with TiO2 nanoparticle incorporation, indicating that the morphology of the PES–PAI polymer remained practically unchanged. Similar observations with defects in the separating layer and insignificant difference in the membrane morphology were reported in the literature too.14,26
Fig. 10 and 11 represent the three dimensional AFM images of pure PES and PES–PAI blend membranes in the absence and presence of TiO2 nanoparticles respectively. From the AFM images, it was revealed that, the surface of both unmodified and modified membranes possess a nodule-valley like structure. In these images, the brightest area represents the highest points or nodules of the membrane surface and dark regions indicate the valleys or membrane pores. From this, it was clear that the surface properties of the PES membranes were improved considerably by means of blending them with PAI. It seems that, in the case of a pure PES membrane, the surface contains large “nodules” and as the concentration of PAI increased the “nodules” decreased their size, indicating a reduction in the pore size of the blend membranes. These high peaks, or nodules, are decreased to a significant level at 80/20 blend composition due to the presence of amorphous PAI. But in the case of a PES–PAI blend at 70/30 composition, the “nodules” reappeared once again and it is larger in size. The results obtained from this AFM analysis are in good agreement with the SEM results. The pores beneath the skin layer of the pure PES membrane were finger like in shape, while blend membrane pores are located far below the dense skin and are equally distributed.
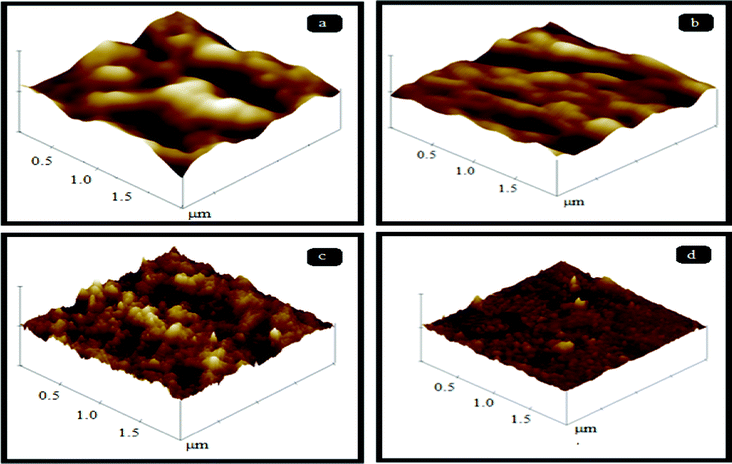 |
| Fig. 10 Three dimensional AFM images of PES–PAI blend membranes (w/w): (a) 100/0; (b) 90/10; (c) 80/20; (d) 70/30. | |
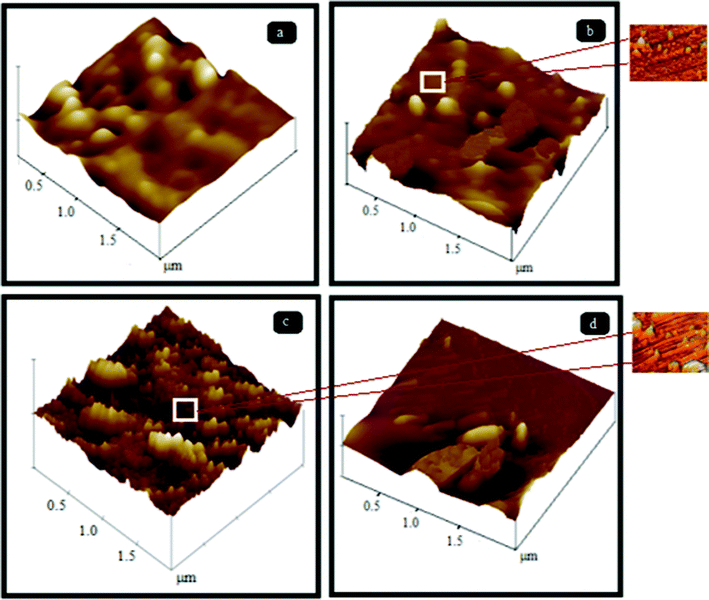 |
| Fig. 11 Three dimensional AFM images of PES–PAI blend membranes (w/w) in the presence of TiO2 nanoparticles: (a) 99/1; (b) 89/10/1; (c) 79/20/1; (d) 69/30/1 (images with a scan area of 0.5 × 0.5 μm are in the outset). | |
The roughness parameters were evaluated from AFM images on different parts of the same membrane and the mean values for each parameter are reported in Table 2. From the results it was clear that, the surface roughness of the membranes decreased with an increase in concentration of PAI in the casting solution. The decrease in surface roughness means that depressions and peaks become smaller; which in turn indicates a decrease in mean pore size. Many researchers reported that the change in the roughness parameters of polymeric membranes was in accordance with the change in the pore size.32–34 When the surface consists of deep depressions (pores) and high peaks (nodules), the tip moves up and down over a wide range and the result should be a high roughness parameter. Elimelech and co-workers32 correlated the surface roughness of the membranes with colloidal fouling and their experiments showed that as the roughness increases, colloidal fouling increases.
Table 2 AFM surface roughness values of the PES–PAI blend membranes
Polymer blend composition (w/w) |
TiO2 nanoparticles (wt %) |
AFM surface analysis data |
R
a (nm) |
R
q (nm) |
R
z (nm) |
100/0 |
0 |
3.17(± 0.49) |
4.28(± 0.27) |
18.43(± 0.83) |
90/10 |
0 |
2.31(± 0.11) |
3.62(± 0.15) |
13.94(± 0.64) |
80/20 |
0 |
1.73(± 0.23) |
2.38(± 0.21) |
8.11(± 0.57) |
70/30 |
0 |
0.89(± 0.10) |
1.53(± 0.12) |
5.08(± 0.46) |
99/0 |
1 |
3.89(± 0.37) |
4.97(± 0.29) |
21.70(± 0.32) |
89/10 |
1 |
3.01(± 0.16) |
4.13(± 0.40) |
16.28(± 0.73) |
79/20 |
1 |
2.47(± 0.43) |
3.21(± 0.28) |
11.42(± 0.68) |
69/30 |
1 |
1.07(± 0.51) |
2.13(± 0.19) |
7.81(± 0.42) |
However, surface roughness values of the TiO2 nanoparticle incorporated membranes slightly increased indicating the directional alignment of filler grains on the surface of the composite membranes rather than a change in pore size. Since the pore size of these nanocomposites from SEM images are comparable to PES–PAI blend membranes we could consider that, TiO2 nanoparticles were either located along the pore edges or were embedded within the polymer matrix. In our AFM images, preferential expulsion of larger aggregates of these TiO2 nanoparticles was not observed at a 2 × 2 μm scan area and this indicates a uniform dispersion in the matrix and this can be attributed to the early capture of these particles within the precipitating matrix.35 We demonstrated that, during the phase inversion process nanoparticles migrate to edges or surfaces of the internal pores which is the most available free surface when the composite’s average pore size is less than the size of the particle. This positioning of TiO2 nanoparticles in pore edges could be demonstrated by the preferential orientation of these particles in the AFM images of 0.5 × 0.5 μm scan size shown in the outset of Fig. 11. Thus we believe that the uniform dispersion achieved by this low loading of TiO2 nanoparticles has a profound effect in the final properties of the composite membranes that will be discussed in the subsequent sections.
3.7. Compaction of PES–PAI blend membranes
When a newly developed nanofiltration membranes is subjected to compressive stress (high applied pressure), the phenomenon of irreversible time-dependent flux decline with a decrease in thickness is generally observed.36 The results obtained for the compaction study is presented in Table 3. The compaction factor calculated by the eqn (2) is acceptable only when the results go with the values of difference between the initial water flux and steady water flux. However, in contrary to our previous publication both compaction factor calculated and difference in water fluxes between the initial state and steady state showed an opposing trend due to the strong dependency of the compaction factor with initial flux. Hence, the difference in water fluxes between the initial and steady stage is taken in to consideration for comparing the compaction behavior of the membranes under study. From Table 3 it is observed that the pure PES membrane in the absence of nanoparticles showed a steady state pure water flux of 294.14 L m−2 h−1 and the absolute flux decline was found to be 110.8 L m−2 h−1. Irrespective of the amount of PES, PAI and TiO2 content all the membranes were compacted and steady state water flux was attained within 30–40 min of operation indicating that main structural changes occurs in the beginning of the operation. In the case of blend membranes an insignificant decrease in the steady state pure water flux and flux decline were observed, indicative of the decrease in pore size of the blend membranes as in concurrence with the SEM analysis results.
Table 3 Initial pure water flux, final pure water flux and compaction factor of PES–PAI blend membranes
Polymer blend composition (w/w) |
TiO2 nanoparticles (wt %) |
Pure water flux (L m−2 h−1) at 2070 kPa |
Compaction factor |
(Initial − final) pure water flux (L m−2 h−1) |
Initial |
Final |
100/0 |
0 |
405.10 |
294.26 |
0.726 |
110.82 |
90/10 |
0 |
378.34 |
279.74 |
0.739 |
98.59 |
80/20 |
0 |
361.52 |
269.80 |
0.746 |
91.71 |
70/30 |
0 |
374.65 |
284.33 |
0.759 |
90.19 |
99/0 |
1 |
420.38 |
324.84 |
0.772 |
95.54 |
89/10 |
1 |
397.45 |
306.49 |
0.771 |
90.95 |
79/20 |
1 |
374.52 |
286.62 |
0.765 |
87.89 |
69/30 |
1 |
382.16 |
298.10 |
0.780 |
84.07 |
When membranes were operated under high external pressure, the thickness and pore size of membranes were decreased by the reorganization of polymeric chains, which lowers the porosity. This leads to an increase in the hydraulic resistance offered by the membrane as a result of the dense structure of the membrane and, consequently, lowers its flux. In compaction, due to an increased pressure drop with distance from the membrane surface, the load on the material is gradually increased with the distance from the surface.36 Thus the ultimate end of the material (the membrane downside) was exposed to the highest compaction pressure. Since most of the membrane space was found beneath the thin separating layer, the pure PES membrane with a finger-like structure was more exposed to structural disorder than the PES–PAI blend membrane with a sponge-type structure. Previous observations in the literature say that the separation properties of the membrane remain unchanged, which means that the separating layer was unchanged, but the total flux/permeability was reduced, which indicates that the overall porosity was reduced.36 In the case of TiO2 incorporated binary blend composite membranes, the absolute flux decrease is significantly less than that of the polymeric blend membranes. Since the compaction preferentially occurs in the porous sub-layer, membranes with homogeneously distributed TiO2 particles along the pore walls imply high resistance to deformation.37 This observation is substantiated by the high AFM surface roughness data by the incorporation of TiO2 in the casting solution.
3.8. Pure water flux of PES–PAI blend membranes
The pure water flux of pure PES, PES–PAI blend membranes and PES–PAI composite membranes is presented in Fig. 12. The pure water flux of blend membranes decreased from 251.62 L m−2 h−1 to 230.82 L m−2 h−1 with changes in the casting solution composition from 100/0 to 80/20 without TiO2 nanoparticles. From this it was clear that, the pure water flux of PES–PAI blend membranes is lower than that of pure PES membranes. These flux values are in clear accordance with the pore size of the membranes observed in the SEM analysis presented in Fig. 8. The addition of PAI in the casting solution results in an increase in skin layer thickness in comparison with the pure PES membrane, a decrease in the finger-like pore size and this thick layer offers high resistance to transport through the membranes. Even though, the pore size of the PES–PAI blend membranes was considerably smaller than the pure PES membranes, the water flux values are comparable. From the pure water flux studies it was clear that, not only does the porosity influence the water flux but also the hydrophilicity and properties of the constituent polymer in the membrane play a vital role.
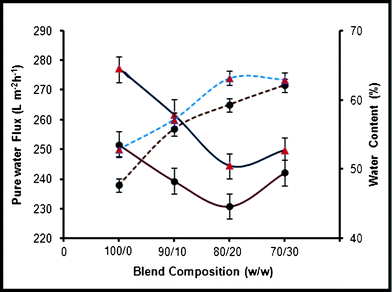 |
| Fig. 12 Effect of PAI concentration on the pure water flux and water content of PES–PAI blend membranes (— pure water flux, water content; ● without TiO2, ▲ with TiO2) | |
The addition of PAI in the PES membrane preparation solution has brought three advantages to the final membrane structure: (i) a change in the skin layer and sub-layer morphology of the membranes (ii) improvement in hydrophilicity and (iii) reduction in the pore size and increase in the porosity of the PES membrane. In the case of the PES–PAI system, polar groups (amide and imide) may effectively compete with the tendency of water molecules to associate with others of their kind, thereby causing a destructuring of the original water complexes and facilitating their transport through the membrane.38,39 However, in the case of pure PES membranes, its highly hydrophobic nature considerably reduces the competency and destructuring of the water clusters, which results in lower water flux. As expected TiO2 nanoparticle modified membranes showed the highest water flux, which may be due to the combined effect of an increase in pore size and hydrophilicity. However, the role of hydrophilicity seems more dominant than pore number and size, which is in good agreement with Luo et al.40 This finding is in contrast with other researchers who believe that the porosity plays the major role.26 TiO2 nanoparticles help to develop a nanogap called the “region of free volume” in the interstitial spaces between the polymer chains because of the repulsive interaction between the highly hydrophilic TiO2 and hydrophobic PES.41 In addition these particles are able to interact with water molecules through van der Waals forces and hydrogen bonds, as described above, and which in turn increases its permeability.
3.9. Water content of PES–PAI blend membranes
The pure PES membrane, in the absence of TiO2 nanoparticles, was found to have a water content of 47.69%, as shown in Fig. 12. In the PES–PAI polymer blend, as the PAI content was increased, the water content improved considerably and at 20% poly(amide-imide) the water content was found to be 59.26%. As the PAI concentration increased, the immiscible nature of blend increased due to low adhesion properties between PES and PAI chains. Further, this leads to the creation of void volume in the membrane because of the increase in the number of super molecular aggregates in the casting solution, which results in the formation of a larger number of pores. This in turn increased the water intake of the pores and enhanced water content at higher PAI composition in the blend. This large difference in water content between the pure PES and PES–PAI blend membranes indicates a change in the peculiar hydrophobic character of PES to hydrophilic by the incorporation of PAI as evidenced in water flux studies. The addition of TiO2 nanoparticles in the casting solution of pure PES further enhanced the water content of the membranes, and at 80/20 blend composition the membrane was found to have a water content of 63.11%. This increase in water content, irrespective of the polymer blend composition was attributed to the hydrophilic nature of TiO2 nanoparticles, which enhances the interaction with water molecules through van der Waals forces and hydrogen bonds.
From the water content values it was concluded that PES membranes adsorbed relatively small amounts of weakly hydrogen bonded clusters compared to the larger amounts of strongly hydrogen bonded clusters in the PES–PAI blend membranes.42 Since the blend membranes posses a sponge-like structure, the small nodules present in the entire membrane cross section act as a domain of water molecules compared to the finger-like structure of pure PES membranes. Thus the polar sites on the surface of the PES membranes placed by the incorporation of PAI support the hydrogen bonding interactions with water and lead to the formation of bigger water clusters in blend membranes. Since the interactions between water and the ions are of a similar order of magnitude as water–water interactions,43 the improved water content in the blend membranes is of significance in salt rejection and that will be discussed in the subsequent section.
3.10. Effect of PAI and TiO2 composition on the contact angle and surface free energy
The effect of TiO2 and PAI composition on the wettability of the blend membranes was studied by measuring the contact angle of the membranes of various compositions and the results are tabulated in Table 4. As shown in the table, the contact angle of the PAI incorporated membranes are smaller than the pure PES membranes, which indicate that the addition of PAI can be a useful way to improve membrane hydrophilicity. In general, contact angle measurement at thermodynamic equilibrium on a polymeric membrane measures its wettability and it is influenced by the chemical composition of the surface of the membrane, porosity and its surface roughness. In the case of PES–PAI blend membranes, the presence of polar amide and imide functional groups effectively compete with water by hydrogen bonding and van der Waals interactions and leads to lower contact angle as explained in the pure water flux studies. The effectiveness of individual atoms and substituent groups in increasing the wettability of polymeric membranes is in the order, N > O > I > Br > Cl > H > F.44 Since nitrogen is completely absent in the pure PES membranes, the trend shown in this case is satisfactory. In the case of PES–PAI membranes with TiO2, the contact angle values were decreased further. In this case, in addition to the hydrogen bonding effect of the functional groups of PAI and –O– atoms of TiO2, the surface porosity has an intense effect on the contact angle, since the water drop could penetrate into the pores gradually due to the capillary force, which decreases the contact angle compared to membranes without TiO2.
Table 4 Surface free energy, interfacial free energy, work of adhesion and spreading co-efficient values calculated from the contact angle valuesa
PES–PAI composition (w/w) |
Contact angle(θ) |
Surface free energy, γsv mJ m−2) |
Interfacial free energy, γsl (mJ m−2) |
Work of adhesion, Wa (mJ m−2) |
Spreading coefficient, Sc (mJ m−2) |
eqn (4b)
b
|
eqn (4c)
|
γ
sl, Wa and Sc values are calculated based on the surface free energy (γsv) values obtained from eqn (4b), Since both eqn (4b) and eqn (4c) have given almost same values for γsv.
γ
lv −72.8 mJ m−2. Numbers in the parenthesis are standard deviation.
|
100/0 |
67.6(± 1.0) |
42.27 |
42.55 |
14.53 |
28.74 |
−45.06 |
90/10 |
62.0(± 1.7) |
45.83 |
46.01 |
11.65 |
35.18 |
−38.62 |
80/20 |
56.2(± 0.9) |
49.46 |
49.57 |
8.96 |
41.50 |
−32.20 |
70/30 |
51.9(± 1.2) |
52.10 |
52.18 |
7.18 |
45.92 |
−27.88 |
99/0 |
59.5(± 2.1) |
47.40 |
47.55 |
10.46 |
37.95 |
−35.85 |
89/10 |
53.0(± 1.4) |
51.53 |
51.51 |
7.62 |
44.81 |
−28.99 |
79/20 |
47.4(± 1.5) |
54.80 |
54.85 |
5.53 |
50.28 |
−23.52 |
69/30 |
39.8(± 1.0) |
59.18 |
59.20 |
3.25 |
56.93 |
−16.87 |
From the measured contact angle values, various surface parameters, such as surface free energy, interfacial free energy, work of adhesion and spreading coefficient were calculated and the results are tabulated in Table 4. For pure PES membranes, the surface free energy, γsv value was 42.27 mJ m−2 and it increased to 52.10 mJ m−2 for blend membranes at 70/30 composition without TiO2. From the results it was clear that, surface free energy increases with the increase in composition of PAI (lower contact angle) in the PES–PAI blend membranes. But in the case of interfacial free energy, γsl shows an opposite trend to that of surface free energy, which decreased with an increase in PAI composition. Interfacial free energy, γsl, for pure PES membranes was 14.53 mJ m−2 and it decreased to 3.25 mJ m−2 for TiO2 incorporated blend membranes at 70/30 composition. In the polymeric membrane blend system, the surface composition may differ greatly from that in the bulk since the components of lower surface free energy always tend to enrich the surface in order to minimize the free energy of the system.44,45 A lower equilibrium interfacial energy surface, that results from the placement of lower surface energy component, is achieved at the cost of maintaining a gradient between the surface and bulk composition. In most membrane separation processes, a lower interfacial free energy surface corresponds to lower fouling. Thus the development of such a low energy, low adhesive surface is accepted as the most promising alternative for the control of fouling.45
The work of adhesion, Wa values calculated follows the same trend as that of surface free energy, that is, the Wa values increases with an increase in PAI composition. Thus for a pure PES membrane the Wa value is 28.74 mJ m−2 and for the blend membranes with TiO2, it has gone up to 56.93 mJ m−2. Adhesion on the low-energy surfaces is very strong whereas that on surfaces possessing a high polar contribution to their surface energy is very low.46 Thus it can be concluded that, the polar group introduced on the surface of the PES membrane by blending with PAI and TiO2 was good enough to modify its adhesive properties. The spreading coefficient values obtained reveal that as the PAI composition increases in the blend system, the spreading coefficient values become less negative which shows an increase in wettability.
3.11. Rejection studies of salt PES–PAI blend membranes
In nanofiltration, the charge of the membrane is significant since charge affects the electrostatic interaction between the charged molecules and membrane surface. However, the charge of the membrane depends on the operational pH of the system because membrane functional groups protonate and deprotonate over the pH under study. The rejection performance of the PES–PAI blend membranes for the salts solutions MgSO4, MgCl2, Na2SO4, and NaCl in the presence of a small quantity of surfactant, SLS, is given in Table 5. From the rejection values it was clear that as the concentration of PAI in the blend system increased, the rejection of a particular electrolyte increased due to the smaller size of the membrane pores, as shown in morphological analysis. The pure PES membranes in the absence of TiO2 nanoparticles exhibited 68.72% rejection for MgSO4, which was lower than that of the PES–PAI blend membranes. The other salts MgCl2, Na2SO4, and NaCl had rejections of 65.91, 58.12 and 26.83% respectively. Thus the percentage rejection of electrolyte solutions was in order MgSO4 > MgCl2 > Na2SO4 > NaCl, and thus the performance of the membrane can be explained on the basis of the Donnan effect and size exclusion mechanism.47
Table 5 Percentage rejection values of the salt in the presence of surfactant by PES–PAI blend membranes
Polymer blend composition (w/w) |
TiO2 nanoparticles (wt %) |
Salt rejection (%) |
MgSO4 |
MgCl2 |
Na2SO4 |
NaCl |
100/0 |
0 |
68.72(± 0.4) |
65.91(± 0.9) |
58.12(± 2.0) |
26.83(± 0.1) |
90/10 |
0 |
86.51(± 1.0) |
82.71(± 1.3) |
79.19(± 1.6) |
32.56(± 0.7) |
80/20 |
0 |
95.76(± 2.1) |
94.47(± 0.5) |
92.29(± 0.8) |
42.86(± 1.3) |
70/30 |
0 |
94.18(± 0.3) |
92.56(± 1.1) |
89.62(± 1.0) |
39.59(± 2.2) |
99/0 |
1 |
71.53(± 1.8) |
65.27(± 1.0) |
60.46(± 0.3) |
23.41(± 1.1) |
89/10 |
1 |
87.13(± 1.5) |
81.95(± 0.7) |
82.17(± 1.5) |
33.67(± 0.4) |
79/20 |
1 |
96.89(± 1.1) |
93.84(± 1.1) |
94.18(± 0.8) |
42.92(± 2.0) |
69/30 |
1 |
96.19(± 0.6) |
93.00(± 2.3) |
93.58(± 1.4) |
41.37(± 1.0) |
The rejection mechanism of this PES–PAI nanofiltration membrane could be explained by (1) steric hindrance, (2) Donnan exclusion, (3) degree of hydration and (4) micelle formation in the presence of surfactant SLS.48,49 At our operational pH 4.0, the amino group of PAI in PES–PAI blend membranes gets protonated (
NH2+) and this imparts a constant positive charge in the membrane surface and in the pore walls. This positive charge on the blend membranes rejects preferably cations of higher valence, which results in the higher rejection of magnesium than sodium salts. According to Elimelech et al., anions can easily approach the membrane surface because they are less hydrated than cations.49 The narrow passage in the separating layer of the blend membrane imposes stronger steric hindrance to the larger hydrated counter-ions, which results in the higher rejection of sulfate than chloride salts. The rejection of a given salt by the membranes was in the order PES–PAI (80/20 wt %) > PES–PAI (70/30 wt %) > PES–PAI (90/10 wt %) > PES (100 wt %). Though the morphology of membranes under this investigation corresponded to “loose” nanofiltration membranes, its percentage salt rejection values were significantly higher and it was attributed to the micelles formed by the surfactant ions. Due to electrostatic attraction between the negatively charged polar head of the surfactant ions and membrane surface, the majority of the surfactant molecules approach the membrane surface. This leads to the formation of micelles by surfactant ions associating with each other to remove their hydrocarbon chains from the bulk water and hence, to reduce the free energy of the system.49,50 These micelles have a capacity to accommodate salt molecules and lead to increased salt rejection. Schematic representation of the micelle and hydration layer formation, during the separation of salt solutions is illustrated in Fig. 13. In TiO2 nanoparticle incorporated membranes, separation efficiency was further increased due to the denser separating layer formed due to the migration of this particles in to the edges or surfaces of the internal pores.
3.12. Fouling analysis of the PES–PAI blend membranes
The antifouling performance of the pristine PES, PES–PAI blend and PES–PAI–TiO2 nano composite membranes was studied by BSA as the model foulant and the results are tabulated in Table 6. Flux decline rate, Rfd, was used to evaluate the fouling resistant ability and the lower value of Rfd corresponds to a higher fouling-resistant ability of the membranes and vice versa. As shown in Table 6, the membranes with 0%, 10%, 20%, and 30% PAI content have an Rfd value of 76.1%, 65.7%, 59.5%, and 52.0%, respectively. However, the flux decline for the PES–PAI blend membranes was slightly high compared to the rather stable flux for the TiO2 incorporated membranes. The results showed that the flux decline rate of the blend and nanocomposites membranes was dramatically decreased compared to that of the PES membrane. Since the introduction of PAI and TiO2`nanoparticles increased the hydrophilicity of the PES membrane surface, adsorbed BSA on these membranes can be more readily dislodged by shear force than those on neat PES membranes.51 It was also mainly the hydrophilic modification of the membrane surface by the introduction of PAI and TiO2 that gave rise to the inhibition of hydrophobic interactions between the BSA and the membrane surface.
Table 6 Summary of the data of flux decline rate, irreversible fouling, reversible fouling, flux recovery rate and normalised rejection of the PES–PAI membranes
Polymer blend composition (w/w) |
TiO2 nanoparticles (wt %) |
Fouling analysis of PES–PAI membranes (%) |
Normalised MgSO4 rejection |
Flux decline rate, Rfd |
Irreversible fouling, Rir |
Reversible fouling, Rr |
Flux recovery ratio, FRR |
At 1000 ppm |
At 2000 ppm |
100/0 |
0 |
76.1(± 1.3) |
32.8(± 0.5) |
20.7(± 0.6) |
68.2(± 1.2) |
1.01 |
1.00 |
90/10 |
0 |
65.7(± 1.8) |
25.3(± 2.1) |
34.2(± 2.0) |
75.8(± 1.5) |
0.99 |
0.99 |
80/20 |
0 |
59.5(± 2.0) |
17.5(± 1.6) |
48.9(± 1.8) |
83.1(± 0.6) |
1.00 |
1.01 |
70/30 |
0 |
52.0(± 1.1) |
12.8(± 0.9) |
64.1(± 1.1) |
88.2(± 2.3) |
0.98 |
0.98 |
99/0 |
1 |
67.7(± 1.0) |
26.4(± 2.6) |
16.3(± 1.0) |
74.2(± 1.9) |
1.00 |
0.98 |
89/10 |
1 |
56.3(± 2.4) |
17.3(± 1.2) |
30.3(± 1.2) |
83.1(± 2.8) |
1.01 |
0.99 |
79/20 |
1 |
47.7(± 1.1) |
9.2(± 2.2) |
47.1(± 1.0) |
91.2(± 1.1) |
0.99 |
1.00 |
69/30 |
1 |
42.9(± 1.0) |
6.1(± 0.4) |
61.0(± 1.0) |
94.1(± 2.3) |
1.00 |
1.01 |
To study the antifouling property in more detail, we calculated reversible flux decline and irreversible flux decline of these membranes, and the results are tabulated in Table 6. It can be seen that the Rir value of the pure PES membrane was larger than that of the PES–PAI blend and PES–PAI–TiO2 nanocomposite membrane and main flux decline was because of irreversible fouling. The existence of PAI and TiO2 on the PES membrane surface increased the hydrophilicity and further reduced irreversible protein adsorption and deposition. However, the membrane fouling of the PES–PAI blend and PES–PAI–TiO2 nanocomposite membrane was largely attributed to reversible membrane fouling, which can be easily removed by simple water washing as illustrated by the flux recovery data of these membranes presented in Table 6. In blend membranes, a hydration layer was formed on the membrane surface by the hydrogen bonds between the hydrophilic reactive sites and water. In blend membranes this water layer keeps the proteins in the bulk solution and minimum availability of such reactive sites for water in pure PES membranes leads to the instantaneous binding of proteins.52
3.13. Chlorine stability studies of the PES–PAI blend membranes
In order to study the chlorine stability, short-time exposure of membranes to high concentration of chlorine was adopted since it is equivalent to the long-time exposure of membranes to low concentration of free chlorine.53 The measured normalized MgSO4 rejection in the presence of surfactant SDS of the virgin and the modified PES blend membranes chlorinated under different concentration are presented in Table 6. It can be observed from the normalized rejection that both virgin and modified PES membranes are resistance to chlorine attack. This could be explained by the fact that the PES membranes are considered highly tolerant to oxidants (>250
000 mg h L−1 for chlorine) and to a wide pH range between 2 to 12. Though the amide group in PAI is susceptible to chlorine attack, the strong stable imide linkage protects it from deterioration during exposure to hypochlorite solution. Thus the incorporation of PAI in the PES matrix did not affect the stability of the PES membranes due to the presence of imide linkages and thus it offers long term value in water purification.23
Conclusion
In the present investigation, high performance PES asymmetric nanofiltration membranes were prepared by a phase inversion technique using hydrophilic PAI and TiO2 nanoparticles as the modification agents. The effect of blend ratio and presence of TiO2 nanoparticles on the morphology, permeation properties, porosity, hydrophilicity, separation efficiency and fouling resistance of the resultant membranes were evaluated. ATR-FTIR measurements revealed the presence of hydrogen-bonding interactions between the pendant oxygen in the backbone of PES and amide groups of PAI in blend membranes. Morphological analysis of the blend membranes revealed that as the weight percentage of PAI in the PES matrix increased, defect free dense skin layer and sponge sub layer were formed in the entire membrane cross section. Nevertheless, there was no significant difference in the overall membrane morphology between the membranes without and with TiO2 nanoparticles, indicating that the morphology of the PES–PAI polymer remained practically unchanged.
Though the pore sizes of the PES–PAI blend membranes were smaller than virgin PES membranes, pure water flux and water content of the blend membranes are comparable to the virgin membranes due to the preferential orientation of the polar groups towards water during the membrane formation process, which leads to the enrichment of the surface with organic functional groups. Surface energy parameters calculated from the contact angle measurements indicated that the interfacial free energy of the blend membranes decreased compared to PES membranes. Overall results suggest that membrane morphology, pure water flux, water content, porosity and hydrophilicity of the prepared PES–PAI blend membranes improved significantly by the incorporation of PAI and TiO2 nanoparticles. These prepared membranes were found to be effective in the separation of divalent salts, such as MgSO4, Na2SO4, MgCl2 and NaCl, in solution from aqueous solution containing surfactant. Fouling propensity of the PES membranes decreased by the incorporation of PAI due to the presence of reactive functional groups, which minimizes the interfacial energy and this improvement in fouling resistant property was more noticeable in the TiO2 incorporated PES–PAI membranes. TiO2 nanoparticles did not have any profound influence on the salt rejection properties of the membranes, however, the other properties of the membranes such as hydrophilicity, free energy and fouling resistance were improved considerably. By comparing the properties (morphology, pure water flux, hydrophilicity and interfacial free energy) and performance (% salt rejection and fouling stability) of the membranes of different compositions, it was found that the PES–PAI–TiO2 blend nanocomposite membrane at 79
:
20
:
1 composition should be considered as the best one for industrial applications. Therefore, PAI and TiO2 nanoparticles should be considered as effective modification agents for the development of low energy, antifouling PES nanofiltration membranes for various industrial separations.
Acknowledgements
The authors gratefully acknowledge Solvay Advanced Polymers, Alpharetta, USA for providing poly(amide-imide) (TorlonRM4000T-HV). The authors also thank University Grants Commission (UGC), New Delhi, India, for financial assistance.
References
- Y. Zhang, Y. Su, W. Chen, J. Peng, Y. Dong and Z. Jiang, Ind. Eng. Chem. Res., 2011, 50, 4678–4685 CrossRef CAS.
- R. Malaisamy and M. L. Bruening, Langmuir, 2005, 21, 10587–10592 CrossRef CAS.
- B. Bernstein, S. Belfer and V. Freger, Langmuir, 2010, 26(14), 12358–12365 CrossRef.
- S. Cheng, D. L. Oatley, P. M. Williams and C.J. Wright, Adv. Colloid Interface Sci., 2011, 164(1–2), 12–20 CrossRef CAS.
- J. Kim and B.V. Bruggen, Environ. Pollut., 2010, 158, 2335–2349 CrossRef CAS.
- Y. N. Wang and C. Y. Tang, J. Membr. Sci., 2011, 376, 275–282 CrossRef CAS.
- Z. Yi, L. P. Zhu, Y. Xu, Y. Zhao, X. Ma and B. Zhu, J. Membr. Sci., 2010, 365, 25–33 CrossRef CAS.
- N. Nady, M. C. R. Franssen, H. Zuilhof and M. S. Eldin, Desalination, 2011, 275(1–3), 1–9 CrossRef CAS.
- Q. Shi, Y. Su, X. Ning, W. Chen, J. Peng and Z. Jiang, J. Membr. Sci., 2010, 347, 62–68 CrossRef CAS.
- A. Rahimpour and S. S Madaeni, J. Membr. Sci., 2010, 360, 371–379 CrossRef CAS.
- A. Rahimpour, S. S. Madaeni and S. Mehdipour-Ataei, J. Membr. Sci., 2008, 311, 349–359 CrossRef CAS.
- S. Rajesh, K. H. Shobana, S. Anitharaj and D. Mohan, Ind. Eng. Chem. Res., 2011, 50, 5550–5564 CrossRef CAS.
- E. Celik, H. Park, H. Choi and H. Choi, Water Res., 2011, 45, 274–282 CrossRef CAS.
- J. N. Shen, H. Ruan, L. Wu and C. Gao, Chem. Eng. J., 2011, 168, 1272–1278 CrossRef CAS.
- A. Sotto, A. Boromand, S. Balta, J. Kim and B. V. Bruggen, J. Mater. Chem., 2011, 21, 10311–10320 RSC.
- Q. H. Zhang, L. Gao and J. K. Guo, Appl. Catal., B, 2000, 26, 207–215 CrossRef CAS.
- Q. H. Zhang, L. Gao and J. K. Guo, Nanostruct. Mater., 1999, 11, 1293–1300 CrossRef CAS.
- A. Nagendran, A. Vijayalakshmi, D. Lawrence Arockiasamy, K. H. Shobana and D. Mohan, J. Hazard. Mater., 2008, 155, 477–485 CrossRef CAS.
- S. Vidya, A. Vijayalakshmi, A. Nagendran and D. Mohan, Sep. Sci. Technol., 2008, 43, 1933–1954 CrossRef CAS.
- D. Y. Kwok and A. W. Neumann, Colloids Surf., A, 2000, 161, 31–48 CrossRef CAS.
- S. Rajesh, A. Jayalakshmi, S. Senthilkumar, H. S. Hari Sankar and D. Mohan, Ind. Eng. Chem. Res., 2011, 50, 14016–14029 CrossRef CAS.
- Y. N. Kwon and J. O. Leckie, J. Membr. Sci., 2006, 282, 456–464 CrossRef CAS.
- A. K. Singh, S. Prakash, V. Kulshrestha and V. K. Shahi, ACS Appl. Mater. Interfaces, 2012, 4(3), 1683–1692 CAS.
- Y. Yang, H. Zhang, P. Wang, Q. Zheng and J. Li, J. Membr. Sci., 2007, 288, 231–238 CrossRef CAS.
- S. Belfer, R. Fainchtain, Y. Purinson and O. Kedem, J. Membr. Sci., 2000, 172, 113–124 CrossRef CAS.
- G. Wu, S. G. Gan, L. Cui and Y. Xu, Appl. Surf. Sci., 2008, 254, 7080–7086 CrossRef CAS.
- A. Razmjou, J. Mansouri and V. Chen, J. Membr. Sci., 2011, 378, 73–84 CrossRef CAS.
- W.J. Lau and A.F. Ismail, Desalination, 2009, 249, 996–1005 CrossRef CAS.
- H. Strathmann, K. Kock, P. Amar and R. W. Baker, Desalination, 1975, 16, 179–203 CrossRef CAS.
- I. C. Kim, H. Yoon and K. K. Lee, J. Appl. Polym. Sci., 2002, 84, 1300–1307 CrossRef CAS.
- Q. T. Nguyen, O. T. Alaoui, H. Yang and C. Mbareck, J. Membr. Sci., 2010, 358, 13–25 CrossRef CAS.
- E. M. Vrijenhoek, S. Hong and M. Elimelech, J. Membr. Sci., 2001, 188, 115–128 CrossRef CAS.
- W. R. Bowen, N. Hilal, R. W. Lovitt and P. M. Williams, J. Colloid Interface Sci., 1996, 180, 350–359 CrossRef CAS.
- K. Boussu, B. V. Bruggen, A. Volodin, J. Snauwaert, C. V. Haesendonck and C. Vandecasteele, J. Colloid Interface Sci., 2005, 286, 632–638 CrossRef CAS.
- J. S. Taurozzi, C. A. Crock and V. V. Tarabara, Desalination, 2011, 269(1–3), 111–119 CrossRef CAS.
- H. Mehdizadeh and J. M. Dickson, Ind. Eng. Chem. Res., 1989, 28, 814–824 CrossRef CAS.
- K. Ebert, D. Fritsch, J. Koll and C. Tjahjawiguna, J. Membr. Sci., 2004, 233, 71–78 CrossRef CAS.
-
R. Kesting, Synthetic Polymeric Membranes, McGraw-Hill: New York, 1971 Search PubMed.
-
R. W. Baker, Membrane Technology and Applications, McGraw- Hill: New York, 2000 Search PubMed.
- M. I. Luo, W. Tang, J. Q. Zhao and C. S. Pu, J. Mater. Process. Technol., 2006, 172, 431–346 CrossRef CAS.
- L. Yong Ng, C. P. Leo and A. W. Mohammad, J. Appl. Polym. Sci., 2011, 121, 1804–1814 CrossRef.
- D. Murphy and M. N. Pinho, J. Membr. Sci., 1995, 106, 245–257 CrossRef CAS.
- W. A. P. Luck, Desalination, 1987, 62, 19–35 CrossRef CAS.
-
R. F. Brady, Polymer Characterization and Analysis, Jr. American Chemical Society, Oxford University Press, New York, 2003 Search PubMed.
- P. Anadao, L. F. Sato, H. Wiebeck and F. R. Valenzuela-Diaz, Appl. Clay Sci., 2010, 48, 127–132 CrossRef CAS.
-
Z. Xu, X. Huang, L. Wan, Surface Engineering of Polymer Membranes Springer: Hangzhou, 2009.
- Y. C. Chiang, Y. Z. Hsub, R. C. Ruaan, C. C. Chuang and K. L. Tung, J. Membr. Sci., 2009, 326, 19–26 CrossRef CAS.
- S. Paria and K. C. Khilar, Adv. Colloid Interface Sci., 2002, 110, 75–95 CrossRef.
- A. M. Childress and M. Elimelech, Environ. Sci. Technol., 2000, 34, 3710–3716 CrossRef CAS.
- H. Gecol, E. Ergican and A. Fuchs, J. Membr. Sci., 2004, 241, 105–119 CrossRef CAS.
- T. H. Bae, I. C. Kim and T. M. Tak, J. Membr. Sci., 2006, 275, 1–5 CrossRef CAS.
- J. A. Koehler, M. Ulbricht and G. Belfort, Langmuir, 1997, 13(15), 4162–4171 CrossRef CAS.
- M. Liu, D. Wu, S. Yu and C. Gao, J. Membr. Sci., 2009, 326, 205–214 CrossRef CAS.
|
This journal is © The Royal Society of Chemistry 2012 |
Click here to see how this site uses Cookies. View our privacy policy here.