DOI:
10.1039/C2RA20093G
(Paper)
RSC Adv., 2012,
2, 6570-6575
A novel kind of coordination polymers employing 2,5-diamino-1,4-benzenedithiol as a bridging ligand: synthesis, structure, optical and magnetic properties†
Received
16th January 2012
, Accepted 10th May 2012
First published on 14th May 2012
Abstract
A serials of organo-soluble transition metal–DABDT coordination polymers were successfully synthesized by the coordination of transition metal ions and DABDT. These coordination polymers were characterized by FTIR, TGA, XRD, UV-Vis spectroscopy, fluorescence spectroscopy, TEM, and vibrational sample magnetometry. Results showed that DABDT coordinated transition metal ions by its amino and sulfydryl groups. The coordination polymers of Co–DABDT, Ni–DABDT, Cu–DABDT and Zn–DABDT exhibited crystal or semi-crystal characteristics, while Fe–DABDT was almost amorphous. The synthesized coordination polymers were soluble in the mixed solvent of DMF and DMSO (volume ratio, 1
:
1). The main UV-Vis absorptions of the as-synthesized transition metal–DABDT coordination polymers were below 400 nm in the ultraviolet region. These coordination polymers emitted cyan light in the DMF and DMSO mixed solution, especially for Zn–DABDT, which emitted obviously stronger cyan light than the other coordination polymers. Fe–DABDT exhibited superparamagnetic character in the approximate range of −5000 G to +5000 G and simple paramagnetic character in the other fields. Co–DABDT and Ni–DABDT followed simple paramagnetic behavior. TEM analysis showed that Ni–DABDT were porous nanospheres with an average diameter of about 69 nm. The rigid molecules of Ni–DABDT arrayed in parallel in the coordination polymer sphere. And Co–DABDT also showed a lamellar structure. The rigid coordination polymer molecules arrayed in parallel due to the formation of hydrogen bond between the –SH and –NH2 groups of adjacent molecular chains in the same plane. And the arrangement between different molecular layers was stacked layer upon layer on account of the π–π stacking of the coordination polymer chains. The solubility of the prepared coordination polymers enabled us to carry out a first-time study on their photophysical behavior. What's more, this is the first time to report the planar and lamellar structure of the transition metal-DABDT coordination polymers through TEM analysis.
1 Introduction
In recent years, there has been an ever-increasing interest in the design and synthesis of multifunctional coordination polymers (CPs) or metal–organic frameworks (MOFs) that display the coexistence or synergism of more than two physical properties in one framework. These materials are constructed by using organic ligands as linkers and metal ions as connectors, which not only possess fascinating structures and infinite topologies, but also have multifunctional properties.1–16 However, it is still a great challenge for the controllable synthesis of CPs, because the self-assembly of CPs from molecular building blocks is influenced greatly by many factors, such as the chemical structure of the ligand, the metal, the anion, the solvent, reaction temperature and pH value.17 The key to success is the design of the molecular building blocks that direct the formation of the desired architectural, chemical, and physical properties of the resulting solid-state materials.18 So the selection of the ligand and metal is a vital subject in the construction of CPs.19
Transition-metal ions are often utilized as versatile connectors in the construction of CPs. Depending on the metal and its oxidation state, their coordination numbers can range from 2 to 7, giving rise to various geometries, which can be realized by changing reaction conditions, such as solvents, counteranions, and ligands.18 Furthermore, the d10 metal ions can exhibit luminescent properties when bound to functional ligands. They have potential application in chemical sensors and photochemistry.19,20 On the other hand, 2,5-diamino-1,4-benzenedithiol dihydrochloride (DABDT) is a latent good building block for constructing CPs or MOFs with interesting topologies and properties because there are four possible bonding sites (two amino nitrogen and two sulfydryl sulfur donors) in its molecule. However, to the best of our knowledge, few report concerns about the synthesis of CPs using transition-metal ions as connectors and DABDT as linker. Kim et al. synthesized DABDT–transition metal ion (Fe(II), Co(II), Ni(II), Cu(II)) complexes for the first time.21,22 The products they got were paramagnetic with a high conductivity. They further assumed these compounds to have a square-planar ladder structure based on the elemental analysis and the structure known for the model complex.21,22 But unfortunately, the products they prepared were insoluble powders,21 and thus make it difficult to process and hinder detailed and more accurate studies on this type of coordination polymer.
In this article, thuswe investigated the synthesis of a series of organo-soluble transition metal–DABDT coordination polymers. In the viewpoint of molecular design, the combination of transition metal ions and DABDT is expected to display integrated properties of both fluorescence and magnetism properties. The synthetic reactions of transition metal–DABDT CPs, which are carried out in dilute solution and low temperature, are anticipated to have low reaction speed, and generate more seed crystals. This results in the slow growth of the coordination polymers. During this process, the interaction between coordination polymers and solvent molecules will be reinforced by the formation of hydrogen bonds between the coordination polymer and the solvent molecules (DMF). As a consequence, the solubility of the coordination polymers might be improved. The prepared CPs could be solubilised in some organic solvents and thereby photophysical measurements were possible at the molecular level in solution. What's more, an ordered topology structure will be generated by the self-assembly of these soluble CPs molecules by the π–π stacking of the benzene rings in the CP backbone and hydrogen bonds between –NH2 and –SH of adjacent molecular chains. More importantly, the planar and layered structure of the transition metal–DABDT coordination polymer was observed in our investigation, and the pseudo ladder-type shape of these polymers assumed by Kim et al.21 was also confirmed. The schematic plot for the synthesis of the transition metal–DABDT CPs is demonstrated in Scheme 1.
 |
| Scheme 1 Synthesis of transition metal–DABDT CPs. | |
Experimental
Materials
2,5-Diamino-1,4-benzenedithiol dihydrochloride (DABDT) was synthesized and purified by the literature procedure.23,24 Transition metal salts (FeCl3, CoCl2, Ni(NO3)2, CuSO4 and ZnCl2) and N,N-dimethylformamide (DMF) were purchased from the Shanghai Clinical Research Center (SCRC), and were used as received.
Measurements
Infrared spectra in KBr pellets were recorded with a Nicolet Fourier transform infrared (FTIR) spectrometer scanning from 4000 to 400 cm−1. Elemental analysis was conducted on an Elementary Varioel apparatus. The TEM measurements of the Ni–DABDT and Co–DABDT nanoparticles were performed on a JEOL-JEM 2010HR transmission electron microscope (TEM) for observation with an accelerating voltage of 200 kV. The alcohol dispersions of Ni–DABDT and Co–DABDT nanoparticles were sonicated for 10 min, and placed on carbon-coated copper grid. TGA experiments were carried out on a NETZSCH TG 209 instrument under nitrogen atmosphere with a heating rate of 15 °C min−1. Wide-angle X-ray diffraction (WAXD) patterns for powder samples at room temperature (ca. 25 °C) were obtained from a Shimadzu X-ray diffractometer (λ = 0.15406 nm). The scanning rate was 2° min−1 with 2θ ranging from 10 to 80°. UV-visible spectra were obtained on a Shimadzu 2550 UV-visible spectrophotometer. Fluorescence spectra were measured on an Edinburgh instruments F900. The magnetic properties of transition metal–DABDT CPs were determined by vibrational sample magnetometry (VSM).
Synthesis of transition metal–DABDT CPs
Into a 100 ml dry three-neck flask, 0.245 g (1 mmol) DABDT was dissolved in 50 mL DMF with constant stirring and dry N2 purging for 5 h. Next, 0.162 g (1 mmol) anhydrous FeCl3 was dissolved in 50 mL H2O. And the resulting FeCl3 solution was added dropwise into the DABDT solution at about 0 °C (in an ice water bath). After this, the pH value of the reaction solution was adjusted to 7.0 by adding aqueous ammonia, and then stirred for another 6 h. The resulting solution was evaporated under reduced pressure, and the solid product was washed three times by deoxygenated water and acetone, respectively, to eliminate the unreacted transition metal salt and DABDT. Finally, the product was dried at 100 °C under reduced pressure for 48 h. The other transition metal–DABDT CPs were prepared using the same procedure to Fe–DABDT coordination polymer. The colors of these products were yellowish-brown for Zn–DABDT and black for the other transition metal–DABDT CPs. These synthesized CPs were soluble in H2SO4 or the mixed solvent of DMF and DMSO (volume ratio, 1
:
1). The photographs of these transition metal–DABDT coordination polymers in the mixed solvent of DMF and DMSO (volume ratio, 1
:
1) are presented in the ESI,† S1.
Results and Discussion
FTIR spectra of transition metal–DABDT CPs
Fig. 1 represents the FTIR spectra of transition metal–DABDT CPs and DABDT. In curve a, strong and broad bands at 2810 cm−1 and a moderate absorption peak at 2580 cm−1 are assigned to the characteristic vibrations of –NH3Cl.23 The absorption at 2460 cm−1 is assigned to the characteristic vibrations of –SH.23 Absorptions at 1568, 1521, and 1485 cm−1 are the characteristic vibrations of the benzene ring.23 The FTIR spectra of DABDT are in accordance with the literature.23 Anal. Calcd for C6H10N2S2Cl2: C, 29.39; H, 4.11; N, 11.43. Found: C, 30.45; H, 4.16; N, 11.93.
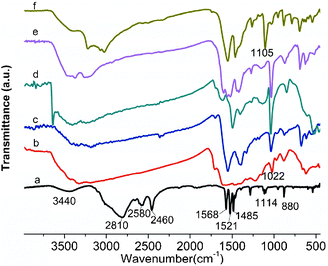 |
| Fig. 1 FTIR spectra of transition metal–DABDT CPs. a: DABDT; b: Fe–DABDT; c: Co–DABDT; d: Ni–DABDT; e: Cu–DABDT; f: Zn–DABDT. | |
The absorptions at 3440 cm−1, which are assigned to the stretching vibration of free –NH2 in DABDT, shift to low wavenumber in the transition metal–DABDT CPs. The absorption peaks at 2810 and 2580 cm−1, which are assigned to the characteristic vibrations of –NH3Cl, become unobvious in the transition metal–DABDT CPs, indicating the coordination of amino group and the metal ions. The absorptions at about 1114 cm−1 is assigned to the characteristic vibrations of Ar–S, which move to about 1022 cm−1 in Fe–DABDT (curve b), Co–DABDT (curve c), Ni–DABDT (curve d), Cu–DABDT (curve e), and 1105 cm−1 in Zn–DABDT (curve f). This indicates the coordination between the sulfur atom of DABDT and the metal ions. In addition, the absorptions at 2460 cm−1 which are assigned to the characteristic vibrations of –SH, are not obvious in all the transition metal–DABDT CPs, confirming the coordination of –SH and transition metal ions.
Co–DABDT: Anal. calcd for C24H32Cl6Co3N8S8: C, 26.73; H, 2.99; N, 10.39. Found: C, 26.43; H, 2.80; N, 8.62.
TEM analysis of Ni–DABDT and Co–DABDT CPs
Fig. 2 gives the TEM micrographs of the as-prepared Ni–DABDT and Co–DABDT CPs. As shown in Fig. 2 a, Ni-DABDT coordination polymer self-assemble into porous nanospheres with an average diameter of 69 nm. The HRTEM image (Fig. 2 b) shows that the rigid molecules of Ni–DABDT are arrayed in parallel in the coordination polymer sphere. The space between the adjacent molecular chains in the same layer is about 0.23 nm, which is in accordance with the diffraction peak at 38.4° in the XRD analysis of Ni–DABDT (Fig. 3). The planar and layered structure of Co–DABDT CPs is shown in Fig. 2 c and Fig. 2 d. The HRTEM image of a selected particle in Fig. 2 d is shown in Fig. 2 e. It is clear that the rigid coordination polymer molecules arrayed in parallel due to the formation of hydrogen bond between the –SH and –NH2 groups of adjacent molecular chains in the same plane. While the arrangement between different molecular layers is layer upon layer accumulation on account of the π–π stacking of the coordination polymer chains. The space between the adjacent molecular chains in the same layer is about 0.34 nm, which is in accordance with the diffraction peak at 25.7° in the XRD analysis of Co–DABDT (Fig. 3). The TEM analysis results of Ni–DABDT and Co–DABDT are consistent with the XRD analysis (Fig. 3), which shows the characteristics of a crystal or half-crystal. The schematic drawing of the parallel arrangement of multi transition metal–DABDT CPs and the orderly stacking of multi layers are shown in Fig. 2 h. To the best of our knowledge, this is the first time that the planar and lamellar structure of the transition metal–DABDT CPs has been reported. This result is in accordance with the pseudo ladder-type shape of these polymers assumed by Kim et al.21
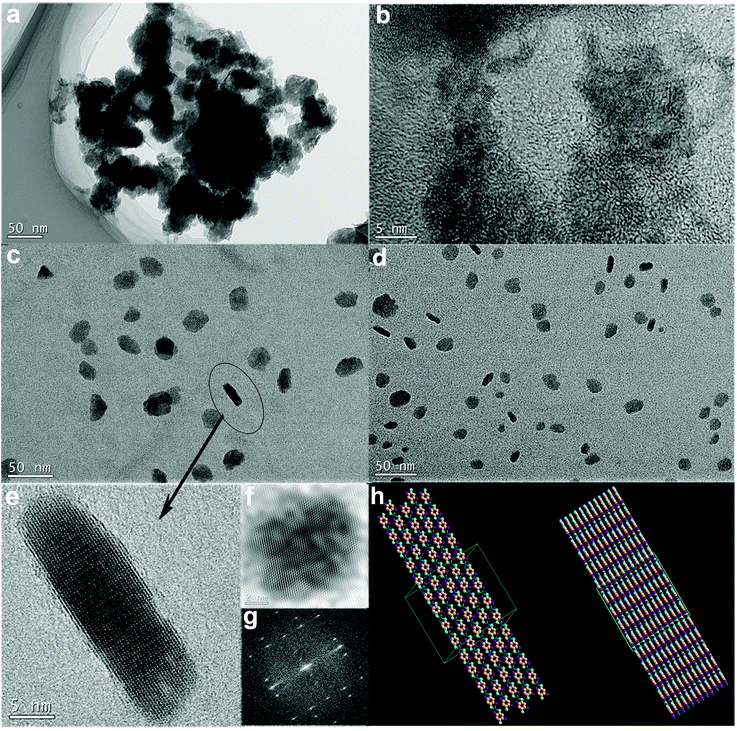 |
| Fig. 2 TEM images of Ni(II)–DABDT and Co(II)–DABDT CPs. a: Ni(II)–DABDT; b: HRTEM image of Ni(II)–DABDT; c: Co(II)–DABDT; d: Co(II)–DABDT; e: HRTEM image of Co(II)–DABDT; f: inverse FFT of Co(II)–DABDT; g: live FFT of Co(II)–DABDT; h: schematic drawing of the planar and layered structure of transition metal–DABDT CPs (left: monolayer; right: multilayer stacking). | |
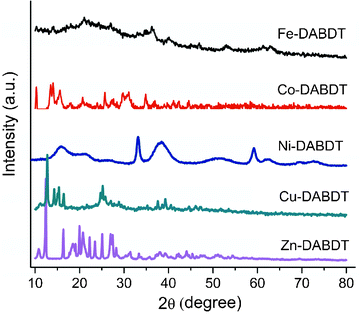 |
| Fig. 3 XRD of transition metal–DABDT CPs. | |
XRD analysis
The crystal structures of the as-synthesized products are examined by powder X-ray diffraction (XRD), and the results are shown in Fig. 3. The CPs of Co, Ni, Cu and Zn have sharp diffraction peaks showing crystal or semi-crystal characteristics, while Fe–DABDT is almost amorphous. Because the metal ions studied in this work have different coordination geometries, so in powder XRD patterns, these synthesized CPs have very different crystal structures.
The UV-Vis spectra of transition metal–DABDT CPs in DMF and DMSO (volume ratio, 1
:
1) mixed solution are illustrated in Fig. 4. Insert is the UV-Vis spectrum of DABDT in water solution, which shows the maximum absorption peak at about 226 nm and a shoulder peak at about 280 nm, belonging to the π–π* transition of benzene ring. The absorption at about 383 nm is attributed to the n-π* transition. The maximum absorption peaks of these transition metal–DABDT CPs are at about 250–270 nm except for Fe–DABDT, which has the maximum absorption at 286 nm and a shoulder peak at 360 nm. The main absorptions of the as-synthesized transition metal–DABDT CPs are below 400 nm in the ultraviolet region, which are tentatively attributed to the π–π* transition of DABDT. While the absorptions above 400 nm are ascribed to ligand-to-metal charge transfer (LMCT).
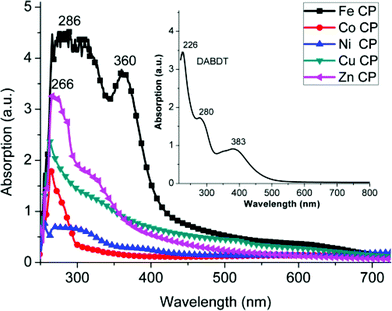 |
| Fig. 4 UV-Vis spectra of transition metal–DABDT CPs in DMF and DMSO (volume ratio, 1 : 1), solution concentration ≈ 0.06 wt%. | |
The fluorescence spectra of transition metal–DABDT CPs in DMF and DMSO solution (volume ratio, 1
:
1) are shown in Fig. 5. All these polymers exhibit broad emissions from about 390 nm to 650 nm. The maximal emission of the as-synthesized CPs are from 480 nm to 491 nm in the cyan region (491 nm for Fe–DABDT, 484 nm for Co–DABDT, and 480 nm for Ni–DABDT, Cu–DABDT and Zn–DABDT, repectively). Zn–DABDT emits obviously stronger cyan light (480 nm) than the other samples. This may be due to the special valence shell structure (3d104s0) of Zn2+. Zn–DABDT is believed to have special electronic properties compared to the other CPs.
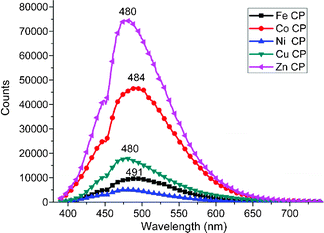 |
| Fig. 5 Fluorescence spectra of transition metal–DABDT CPs in DMF and DMSO (volume ratio, 1 : 1) (excitation 380 nm, solution concentration ≈ 0.06 wt%). | |
Thermal properties
The thermal gravimetric analysis of the transition metal–DABDT coordination polymer is shown in Fig. 6 and Table 1. All samples exhibit a gradual weight loss between 20 and 250 °C, corresponding to the release of water and DMF guest molecules (calc. 6–22.1%). The following decrease of mass above 250 °C can be associated with the destruction of the metal–organic framework. The residue weights at 150 °C are 94.0% (Fe–DABDT), 88.4% (Co–DABDT), 95.8% (Ni–DABDT), 93.3% (Cu–DABDT), and 98.7% (Zn–DABDT), indicating the release of absorbed water with weight losses of 6.0%, 11.6%, 4.2%, 6.7%, and 1.3%, respectively. The residue weights at 250 °C are 87.3% (Fe–DABDT), 83.1% (Co–DABDT), 94.0% (Ni–DABDT), 77.9% (Cu–DABDT), and 91.7% (Zn–DABDT), indicating the release of absorbed DMF with weight losses of 6.7%, 5.3%, 1.8%, 15.4%, and 7.0%, respectively. The residue weights at 800 °C are 19.0% (Fe–DABDT), 35.8% (Co–DABDT), 55.5% (Ni–DABDT), 46.7% (Cu–DABDT), and 54.0% (Zn–DABDT), respectively. The thermal stability of the prepared transition metal–DABDT CPs is good.
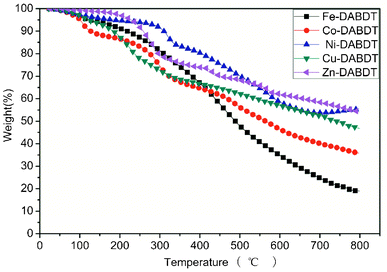 |
| Fig. 6 TGA of transition metal–DABDT CPs. | |
Table 1 Thermal properties of transition metal–DABDT CPs
Sample |
TGA |
Weight at 100 °C (%) |
Weight at 150 °C (%) |
Weight at 250 °C (%) |
Weight at 800 °C (%) |
Fe–DABDT |
96.5 |
94.0 |
87.3 |
19.0 |
Co–DABDT |
95.1 |
88.4 |
83.1 |
35.8 |
Ni–DABDT |
97.3 |
95.8 |
94.0 |
55.5 |
Cu–DABDT |
97.0 |
93.3 |
77.9 |
46.7 |
Zn–DABDT |
99.3 |
98.7 |
91.7 |
54.0 |
Magnetic properties
The hysteresis loops of transition metal–DABDT CPs are presented in Fig. 7. The hysteresis loops of Co–DABDT (Fig. 7 b) and Ni–DABDT (Fig. 7 c) show that they follow simple paramagnetic behavior. The hysteresis loop of Fe–DABDT (Fig. 7 a) exhibits superparamagnetic character in the approximate range of −5000 G to +5000 G and simple paramagnetic character (no magnetic saturation) in the fields below about −5000 G and above 5000 G. The hysteresis loops of Cu–DABDT and Zn–DABDT are shown in Fig. 7 d and e, respectively. We tentatively consider them to be diamagnetism.
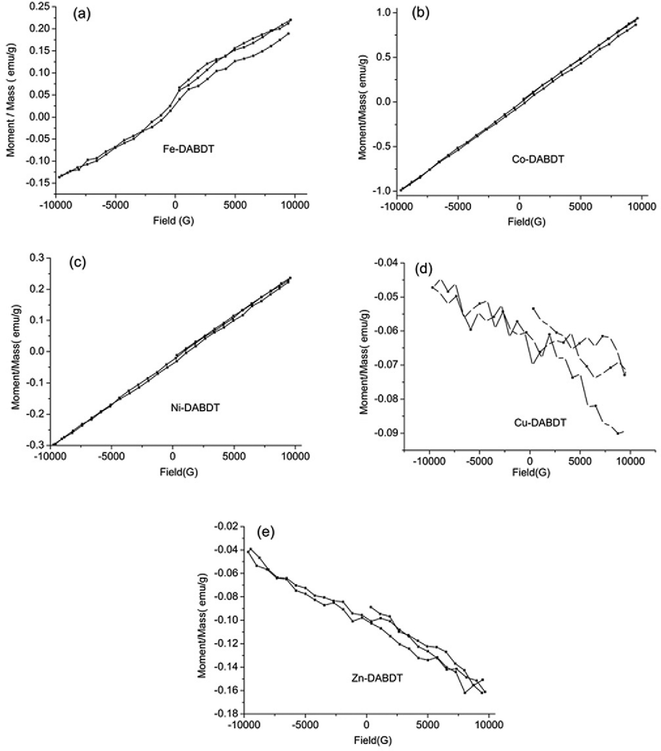 |
| Fig. 7 Hysteresis loops of transition metal–DABDT CPs. | |
Conclusion
In this work, five transition metal–DABDT CPs were successfully synthesized by the coordination of transition metal ions and the amino and sulfydryl groups of DABDT using DMF and water as the solvent. The coordination polymers of Co(II), Ni(II), Cu(II) and Zn(II) had sharp diffraction peaks showing crystal or semi-crystal characteristics, while Fe–DABDT was almost amorphous. The synthesized CPs were soluble in the mixed solvent of DMF and DMSO (volume ratio, 1
:
1). The main UV-Vis absorptions of the as-synthesized transition metal–DABDT CPs were below 400 nm in the ultraviolet region. All these polymers emitted cyan light (from 480 nm to 491 nm) in DMF and DMSO mixed solution, especially for Zn–DABDT, which emitted obviously stronger cyan light than the other CPs. Fe–DABDT exhibited superparamagnetic character in the approximate range of −5000 G to +5000 G and simple paramagnetic character in the other fields. Co–DABDT and Ni–DABDT followed simple paramagnetic behavior. TEM analysis showed that Ni–DABDT formed porous nanospheres with an average diameter of about 69 nm. The rigid molecules of Ni–DABDT arrayed in parallel in the coordination polymer sphere. And Co–DABDT also showed a lamellar structure. The rigid coordination polymer molecules arrayed in parallel due to the formation of hydrogen bond between the –NH2 and –SH groups of adjacent molecular chains in the same plane. The arrangement between different molecular layers was stacked layer upon layer on account of the π–π stacking of the CPs chains. The solubility of the prepared CPs enabled us to carry out a first-time study on their photophysical behaviors. What's more, this is the first time to report the planar and lamellar structure of the transition metal-DABDT coordination polymers through TEM analysis.
References
- H. Furukawa, N. Ko, Y. B. Go, N. Aratani, S. B. Choi, E. Choi, A. Ö. Yazaydin, R. Q. Snurr, M. O'Keeffe, J. Kim and O. M. Yaghi, Science, 2010, 329, 424 CrossRef CAS.
- H. X. Deng, C. J. Doonan, H. Furukawa, R. B. Ferreira, J. Towne, C. B. Knobler, B. Wang and O. M. Yaghi, Science, 2010, 327, 846 CrossRef CAS.
- K. Hirai, S. Furukawa, M. Kondo, H. Uehara, O. Sakata and S. Kitagawa, Angew. Chem., Int. Ed., 2011, 50, 8057 CrossRef CAS.
- A. Facchetti, Angew. Chem., Int. Ed., 2011, 50, 6001 CrossRef CAS.
- R. Kaminker, R. Popovitz-Biro and M. E. van der Boom, Angew. Chem., Int. Ed., 2011, 50, 3224 CrossRef CAS.
- J. Huo, L. Wang, E. Irran, H. J. Yu, J. M. Gao, D. S. Fan, B. Li, J. J. Wang, W. B. Ding, A. M. Amin, C. Li and L. Ma, Angew. Chem., Int. Ed., 2010, 49, 9237 CrossRef CAS.
- T. K. Maji, R. Matsuda and S. Kitagawa, Nat. Mater., 2007, 6, 142 CrossRef CAS.
- M. Oh and C. A. Mirkin, Nature, 2005, 438, 651 CrossRef CAS.
- B. Zhou, A. Kobayashi, H. B. Cui, L. S. Long, H. Fujimori and H. Kobayashi, J. Am. Chem. Soc., 2011, 133, 5736 CrossRef CAS.
- I. Welterlich and B. Tieke, Macromolecules, 2011, 44, 4194 CrossRef CAS.
- J. Puigmartí-Luis, M. Rubio-Martínez, U. Hartfelder, I. Imaz, D. Maspoch and P. S. Dittrich, J. Am. Chem. Soc., 2011, 133, 4216 CrossRef.
- A. Carné, C. Carbonell, I. Imaz and D. Maspoch, Chem. Soc. Rev., 2011, 40, 291 RSC.
- J. R. Long and O. M. Yaghi, Chem. Soc. Rev., 2009, 38, 1213 RSC.
- M. Yoshizawa, J. K. Klosterman and M. Fujita, Angew. Chem., Int. Ed., 2009, 48, 3418 CrossRef CAS.
- L. J. Murray, M. Dincă and J. R. Long, Chem. Soc. Rev., 2009, 38, 1294 RSC.
- Y. M. Xie, J. H. Liu, X. Y. Wu, Z. G. Zhao, Q. S. Zhang, F. Wang, S. C. Chen and C. Z. Lu, Cryst. Growth Des., 2008, 8, 3914 CAS.
- X. Meng, Y. Song, H. Hou, H. Han, B. Xiao, Y. Fan and Y. Zhu, Inorg. Chem., 2004, 43, 3528 CrossRef CAS.
- S. Kitagawa, R. Kitaura and S. Noro, Angew. Chem., Int. Ed., 2004, 43, 2334 CrossRef CAS.
- H. Y. Bai, J. F. Ma, J. Yang, B. Liu, L. P. Zhang, J. C. Ma and Y. Y. Liu, Eur. J. Inorg. Chem., 2010, 5709 CrossRef CAS.
- Q. Wu, M. Esteghamatian, N. X. Hu, Z. Popovic, G. Enright, Y. Tao, M. D. Iorio and S. Wang, Chem. Mater., 2000, 12, 79 CrossRef CAS.
- O. K. Kim, T. E. Tsai, T. H. Yoon and L. S. Choi, Synth.
Met., 1993, 59, 59 CrossRef CAS.
- O. K. Kim, T. H. Yoon and D. McDermott, J. Chem. Soc., Chem. Commun., 1989, 740 RSC.
- J. F. Wolfe and B. H. Loo, Macromolecules, 1981, 14, 915 CrossRef CAS.
- J. A. Osaheni and S. A. Jenekhe, Chem. Mater., 1992, 4, 1282 CrossRef CAS.
Footnote |
† Electronic supplementary information (ESI) available. See DOI: 10.1039/c2ra20093g |
|
This journal is © The Royal Society of Chemistry 2012 |