DOI:
10.1039/C2RA00947A
(Paper)
RSC Adv., 2012,
2, 6036-6041
Rapid detemplation of nanozeolite β: microwave-assisted Fenton-like oxidation†
Received
21st October 2011
, Accepted 25th April 2012
First published on 31st May 2012
Abstract
An in situ microwave-assisted Fenton-like oxidation has been developed for detemplation of the nanozeolite β without any pretreatment. The influencing factors on the removal of structure-directing agents (SDAs) in the nanozeolite β, such as the composition of Fenton-like reagents (pH, H2O2/TEA+, Fe3+/H2O2) and microwave operation conditions, are investigated, to find that the SDAs in the micropores and solution of the as-prepared nanozeolites could be completely eliminated within 15 min. In comparison with those obtained by the traditional high-temperature calcination, the Fenton-treated nanozeolite particles not only preserve their monodispersity and large external surface area, but also avoid the formation of extra-framework aluminum, which renders an enhanced catalytic performance in fructose dehydration.
Introduction
Nanosized zeolites (nanozeolites) are believed to have excellent applications as a catalyst, adsorbent, carrier for inorganic and organic molecules and building blocks of hierarchical structure, owing to their small size, short channel, large external surface area, defined porosity and controllable surface properties.1,2 Many nanozeolites are usually synthesized in the presence of structure-directing agents (SDAs, e.g. quaternary ammonium salts).3 The SDAs will be embedded in the micropores of the resulting nanozeolite particles, which have to be removed before their application. So far, the most widely used method for the removal of SDAs is calcination at high temperature (400–600 °C). However, the calcination process often not only causes irreversible aggregation of nanozeolites, so that they lose their monodispersity and external surface, but also leads to structural and compositive inhomogeneities. These will greatly influence the physical and chemical properties of the nanozeolites. For example, the dealumination caused by calcination often produces extra-framework aluminum (EF-Al), which will affect the amount and distribution of acid sites as well as the catalytic properties of the nanozeolite.4 Moreover, such dealumination will become more visible for zeolite β due to its larger framework flexibility and higher number of T-atoms in the four-membered rings.4 Zeolite β is of special interest because of its 12-ring pore structure, which is large enough to accommodate commercially interesting hydrocarbons, allowing catalytic reforming, alkylation, dewaxing, cracking and so on.5 Recently, several low-temperature detemplation (T < 200 °C) techniques have been developed, especially advanced oxidation methods using nitric acid/hydrogen peroxide,6 ozone/oxygen,7 ultraviolet light,8 oxygen plasma9 and Fenton reagent4 as the oxidants. The mild treatment conditions not only avoid the huge consumption of heat but also preserve the morphology, size and pristine structure of the materials. However, these methods are usually time consuming and employ complex manipulation and instruments.
As a new kind of clean energy, microwaves are widely used in chemical synthesis owing to their unique energy conversion mode.10 The dielectric heating characteristics of microwaves can bring about the selective heating of different substrates or solvents in a reaction system,10,11 and therefore significantly reduce the synthesis time from several days to several hours, or even minutes.
Herein, we have developed an in situ microwave-assisted Fenton-like oxidation method (Fe3+/H2O2) to quickly remove SDAs of the as-prepared nanozeolites β. Compared with the traditional high-temperature calcination, this detemplation method is able to not only completely remove SDAs in the as-prepared nanozeolites within 15 min but also preserves the intrinsic framework structure and monodispersity of the nanozeolites, which ensures their promising catalytic and assembly performance.
Experiment
Reagents
Fused silica (Aerosil 400, Shanghai Chlorine Alkali Industry), tetraethylammonium hydroxide (TEAOH, 20 wt%, Sinopharm Chemical Reagent Co. Ltd), aluminum foil (≥99.5%, Sinopharm Chemical Reagent Co. Ltd), ferric nitrate (Fe(NO3)3·9H2O, Shanghai Reagent factory), hydrochloric acid (HCl, 36 wt%, Shanghai Reagent factory), H2O2 (30 wt%, Shanghai Reagent factory) and distilled water, were used.
Synthesis of nanozeolite β
Nanozeolite β was hydrothermally synthesized under microwave irradiation according to a gel composition of 1SiO2:0.016Al2O3:0.16(TEA)2O:10.78H2O.12 After the relatively low temperature process (100 °C) for 60 min under the microwave irradiation, the temperature is increased to 150 °C for about 360 min to complete the crystallization of nanozeolite β. The resulting colloidal solution of nanozeolites was collected without any treatment.
Microwave assisted Fenton-like oxidation process
The detemplation is carried out in a Teflon reactor under microwave irradiation for 15 min (Table 1). The microwave oven (Sineo MDS-6, Shanghai) used here is able to operate with controlled pressure and temperature. The non-pulsed microwave irradiation heats continuously and precisely (limit of accuracy: ±0.1 MPa) in the pressure range 0–5 MPa. In detail, the microwave-assisted detemplation procedure was divided into two stages according to the batched addition of H2O2 (30 wt%). In Stage I, a portion of H2O2 was added into the as-prepared β nanozeolite solution to avoid the possibility of high system pressure caused by the rapid decomposition of H2O2, and then the pH of the system was adjusted to 2–7 by 0.5 M HCl. After a portion of 0.2 M Fe(NO3)3 aqueous solution was added, the reactor was irradiated by microwaves for 5 min. Then, in Stage II, another portion of H2O2 was added into the system and reacted for another 10 min under microwave irradiation. Finally, the obtained samples were washed with 0.05 M HCl and deionized water. The added amount of H2O2 depends on the content of TEA+ in a nanozeolite solution, while the latter was determined by the volume of the as-prepared β nanozeolite solution and the mass of TEAOH used in the synthesis gel (ESI †). As shown in Table 1, a series of H2O2/TEA+, Fe3+/H2O2 molar ratios and microwave conditions were adopted to explore the optimized conditions for detemplation.
Table 1 Summary of the composition of Fenton-like reagents, microwave operating conditions and textural properties of nanozeolite β, detemplated by Fenton-like oxidation and calcination
Sample |
Fenton-like reagenta |
MW conditions |
Textural property of sample |
pH |
H2O2/TEA+ (mol/mol) |
Fe3+/H2O2 (10−6 mol/mol) |
Pressure (MPa) |
Power (W) |
BET surface area (m2 g−1) |
External surface area (m2 g−1) |
Micropore area (m2 g−1) |
Micropore volume (cm3 g−1) |
Stage I |
Stage II |
The reaction temperature of Fenton-like treated systems is about 170–190 °C.
The sample was calcined at 550 °C for 350 min.
|
1 |
2 |
35.7 |
71.3 |
41.2 |
2.0 |
800 |
643 |
261 |
382 |
0.180 |
2 |
3 |
35.7 |
71.3 |
41.2 |
2.0 |
800 |
652 |
267 |
385 |
0.181 |
3 |
7 |
35.7 |
71.3 |
41.2 |
2.0 |
800 |
309 |
92 |
217 |
0.101 |
4 |
3 |
35.7 |
71.3 |
30.9 |
2.0 |
800 |
609 |
267 |
342 |
0.158 |
5 |
3 |
35.7 |
59.5 |
30.9 |
2.0 |
800 |
655 |
258 |
397 |
0.184 |
6 |
3 |
35.7 |
35.7 |
30.9 |
2.0 |
800 |
528 |
242 |
286 |
0.133 |
7 |
3 |
35.7 |
23.8 |
30.9 |
2.0 |
800 |
417 |
216 |
201 |
0.091 |
8 |
3 |
35.7 |
59.5 |
51.5 |
2.0 |
800 |
584 |
255 |
329 |
0.152 |
9 |
3 |
35.7 |
59.5 |
15.5 |
2.0 |
800 |
552 |
208 |
344 |
0.159 |
10 |
3 |
35.7 |
59.5 |
7.7 |
2.0 |
800 |
396 |
157 |
239 |
0.110 |
11 |
3 |
35.7 |
59.5 |
0 |
2.0 |
800 |
303 |
94 |
209 |
0.106 |
12 |
3 |
35.7 |
59.5 |
30.9 |
0.5 |
800 |
494 |
205 |
289 |
0.134 |
13 |
3 |
35.7 |
59.5 |
30.9 |
1.0 |
800 |
561 |
190 |
371 |
0.171 |
14 |
3 |
35.7 |
59.5 |
30.9 |
1.5 |
800 |
560 |
188 |
372 |
0.173 |
15 |
3 |
35.7 |
59.5 |
30.9 |
2.0 |
400 |
595 |
216 |
379 |
0.174 |
16 |
3 |
35.7 |
59.5 |
30.9 |
2.0 |
600 |
586 |
187 |
399 |
0.184 |
17 |
Calcined βb |
527 |
139 |
388 |
0.183 |
Catalytic dehydration of fructose
Dehydration of fructose was catalyzed by both Fenton-treated β and calcined β nanozeolites at 550 °C. 0.2 g fructose was dissolved in 3.8 g water, and then 50 mg of nanozeolite was added into the system. The reaction was carried out under microwave irradiation (Discover SP, CEM) at 150 °C for 5–20 min. The reaction process was tracked, and the products collected at different reaction times were analyzed by high performance liquid chromatography (HPLC, Shimazu) equipped with a refractive index detector (RID) and a shodex SH1011 sugar column. The column oven temperature was 40 °C, and the mobile phase was a 0.5 mM sulfuric acid aqueous solution at a flow rate of 1 mL min−1. The conversion of fructose and the yield of 5-HMF were calculated by the following equations: |  | (1) |
| 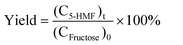 | (2) |
where (CFructose)0, (CFructose)t, and (C5-HMF)t represent the original molar concentration of fructose, and the molar concentrations of fructose and 5-HMF in the products collected at different times.
Characterization
The crystallinity of the products was checked by X-ray diffraction (XRD, Rigaku D/max-IIA). The size, morphology and dispersibility of the crystal particles were observed by scanning electron microscopy (SEM, Philips XL 30) and transmission electron microscopy (TEM, JEOL JEM-2010). Size distribution measurements of the nanozeolite were collected by zetasizer (Nano-ZS90, Malvern). The SiO2/Al2O3 and Fe/Si ratios of nanozeolites were detected by energy-dispersive X-ray spectroscopy (EDX, Philips XL 30). The degree of detemplation is determined by thermogravimetric analysis (TGA) and mass spectroscopy (MS, Mettler Toledo, TGA/SDTA 851 e) to measure the mass decrease and burning production upon heating in air up to 1173 K, with a heating rate of 10 °C min−1. By Fourier transform infrared spectroscopy (FTIR, Thermo Nicolet, Nexus) using KBr as the background, the organic groups of SDAs are characterised before and after detemplation. The framework structures of the nanozeolites were detected by 27Al nuclear magnetic resonance (NMR, DSX-300, Bruker). Nitrogen adsorption (Micromeritics, ASAP, 2010) tests were conducted to provide information on the textural properties of the nanozeolites and to confirm the removal of the SDAs from the framework. The total acidity of the nanozeolites was studied by auto-titration device (ZDJ-5, Shanghai Jingke), using an n-butylamine titration method.
Results and discussion
Structure characterization of nanozeolite β detemplated by Fenton-like oxidation
The SDAs in nanozeolite β are removed successfully in 10–15 min via this Fenton-like method. Fig. 1 shows SEM and TEM images, the XRD pattern and the size distribution of nanozeolites β detemplated by Fenton-like oxidation (Fenton-treated β). Obviously, as shown in Fig. 1a–d, Fenton-treated β nanozeolite particles display a high monodispersity and a uniform size distribution, centered close to 150 nm. The usual agglomeration of nanozeolite particles caused by calcination (calcined β, Fig. S1†) is avoided. Furthermore, Fenton-treated β shows almost the same XRD pattern (Fig. 1b and Fig. S2b†) as the as-prepared nanozeolite β (Fig. S2a†), while the calcined sample presents obviously widened and weakened diffraction peaks (Fig. S2c†). TEM images clearly show that all the crystal plane strings go through the whole of the particles (Fig. 1c and d), revealing the preservation of the highly ordered porous structure in this sample. Moreover, the size distribution of Fenton-treated β (Fig. 1e) is even the same as that of as-prepared β (Fig. 1f), indicating again that the Fenton-like treatment can well preserve the monodispersity and size of detemplated nanozeolites particles.
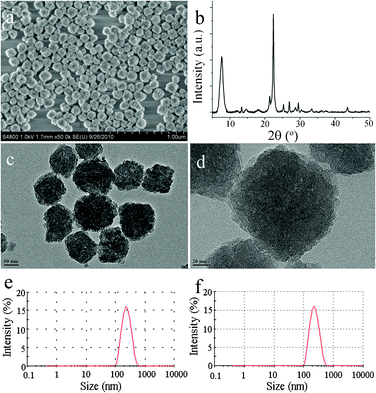 |
| Fig. 1 SEM image (a), XRD pattern (b), TEM images (c and d) and size distribution (e) of Fenton-treated β as well as size distribution of as-prepared β (f). | |
The efficiency of this process can be proven by FTIR, TGA and TG-MS analysis. As shown in Fig. 2a, the C–H stretching (2987, 2942 and 2880 cm−1) and bending (1483, 1459 and 1396 cm−1) vibrations owing to organic SDAs6,13 completely vanish after Fenton treatment. Furthermore, the vibration of H2O at 1640 cm−1 of Fenton-treated β (Fig. 2a-II) becomes stronger than that of as-prepared β (Fig. 2a-I) due to more adsorbed water in their empty channels. Correspondingly, the TGA plot (Fig. 2b-II) after Fenton oxidation shows a much larger mass loss (ca. 5.45 wt%) than that of the as-prepared β (ca. 1.54 wt%) for physisorbed water at about 70 °C.14,15 But no weight loss between 150 and 450 °C (Fig. 2b-II), which corresponds to the SDA signals as the as-prepared β shows (Fig. 2b-I), is observed. Moreover, the MS signals (Fig. S3†) further show that as-prepared β displays visible mass loss signals at m/z of 44 (CO2+), 46 (NO2+) and 18 (H2O+) during the whole heating treatment from 25 to 480 °C. By contrast, for the Fenton-treated β, all the corresponding mass loss signals become invisible, and only a mass signal with an m/z of 18 is observed at about 70 °C, which can be assigned to physisorbed water in the empty micropores. All these mean that there is no SDA left in the Fenton-treated β.
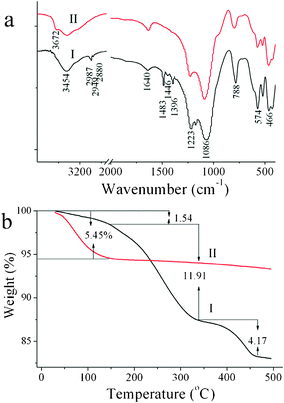 |
| Fig. 2 FTIR spectra (a) and TGA (b) of as-prepared β (I) and Fenton-treated β (II). | |
In addition, the complete removal of SDAs from the nanozeolites can also be proved by the results of N2 adsorption experiments (Table 1). According to Table 1, the microporous areas and volumes of Fenton-treated β (Sample 5) and calcined β (Sample 17) are very similar, which not only reveals the structure integrality of Fenton-treated β after the Fenton-like oxidation process, but also confirms its complete detemplation. Moreover, because the Fenton-like oxidation process avoids the aggregation of nanozeolites that would be caused by calcination, and preserves the monodispersity of zeolite nanoparticles, the Fenton-treated β sample possesses a much larger external surface area (258 m2 g−1) than the calcined one (139 m2 g−1).
27Al magic-angle-spinning nuclear magnetic resonance (27Al MAS NMR)15 is applied to detect the influence of the Fenton oxidation process on the framework Al atom of nanozeolites (Fig. 3).4 Similarly to as-prepared β (Fig. 3a), Fenton-treated β (Fig. 3b) exhibits only a resonance of 53 ppm corresponding to its tetrahedral coordinated framework Al(IV). However, for calcined β (Fig. 3c), a small but sharp peak appears at ca. 0 ppm, which can be attributed to the octahedral EF-Al(VI), and the asymmetry of its framework Al(IV) peak indicates the distortion of the symmetrical environment of Al(IV). Furthermore, the SiO2/Al2O3 ratio analysis of Fenton-treated β (SiO2/Al2O3 = 13.7) also shows that there is not an obvious loss of Al compared to that of as-prepared β (SiO2/Al2O3 = 13.3), implying that the Al species of Fenton-treated β is kept well within its framework.
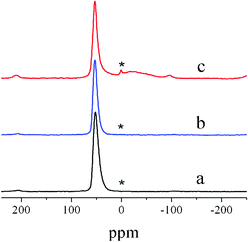 |
| Fig. 3
27Al MAS NMR spectra of as-prepared β (a), Fenton-treated β (b) and calcined β (c). | |
Nonaqueous potentiometric titration with n-butylamine16 is used to evaluate the acidity of Fenton-treated β, while the calcined β is adopted as a reference. It has been suggested that the initial electrode potential (Ei) indicates the maximum acid strength, and the consuming amount of amine per gram of catalyst at 100 mV reflects the number of very strong acid sites.17 The titration result (Fig. 4) shows that both samples have very strong acidity but Fenton-treated β displays a slightly higher Ei and a larger amount of very strong acid, which could result from its well preserved intrinsic framework structure and framework Al.
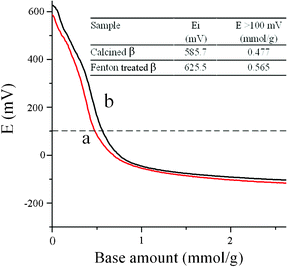 |
| Fig. 4 Acidity measurements of (a) calcined and (b) Fenton-treated β by nonaqueous potentiometric titration in acetonitrile using n-butylamine. The inset summarizes the acidic properties of these two samples. | |
The influence factor to template removing efficiency
The Fenton reaction is known to be very effective for the removal of many hazardous organic pollutants from water, whose main advantage is the complete destruction of contaminants to harmless compounds, e.g. CO2, water and inorganic salts. Similar to Fenton’s reagent, Fenton-like oxidation (Fe3+/H2O2) causes the formation of highly reactive hydroxyl radicals that could attack and destroy the organic pollutants. The reaction process can be described as follows: | Fe3+ + H2O2 → HO2· + Fe2+ + H+ | (1) |
| Fe2+ + H2O2 + H+ → Fe3+ + ·OH + H2O | (2) |
| Fe3+ + HO2· → Fe2+ + O2 + H+ | (3) |
| ·OH + H2O2 → H2O + HO2· | (4) |
| RH (organic molecules) + ·OH → CO2 + H2O + inorganic salts | (5) |
To deeply understand the microwave-assisted Fenton-like oxidation process, the effects of Fenton-like reagents (pH, concentration of Fe3+ and H2O2) and microwave operating conditions (system pressure and maximum microwave power) are investigated in detail. The results are listed in Table 1. Because the presence of H+ could not only avoid the formation of iron hydroxide but also favor the formation of hydroxyl radicals,18 0.5 M HCl is used to adjust the sol system of the as-prepared β from basicity (pH = 11) to acidity. It is found that SDAs in the as-prepared β nanozeolite are able to be completely removed at pH 3 (Sample 2). Its BET surface area and microporous volume can reach 0.181 cm3 g−1 and 652 m2 g−1, respectively. At the same time, EDX analysis shows that the survival of the Fe species after detemplation is very tiny (Fe/Si = 2.1 × 10−3 mol/mol).
The effects of H2O2 and Fe3+ concentrations on the removal of SDA are listed in Fig. 5 and Table 1. The optimum result can be obtained at H2O2/TEA+ = 95.2 mol/mol and Fe3+/H2O2 = 30.9 μmol/mol (Sample 5, Table 1). In this case, SDAs in the as-prepared β nanozeolites can be completely removed within 15 min. More or less H2O2 and Fe3+ are not so effective in obtaining a larger microporous area (Fig. 5). When the amount of H2O2 is too low, it is not enough to remove all the organic SDAs. Contrarily, although a larger amount of H2O2 could ensure the complete detemplation of nanozeolites β, too much O2 produced in a very short time will rapidly increase the pressure of the system, causing damage to the zeolite framework. As a result, Sample 4 (H2O2/TEA+ = 107.0 mol/mol) in Table 1 displays a decreasing micropore surface area but an increasing external surface area compared to Sample 5. On the other hand, a small quantity of Fe3+ (15.5–30.9 μmol/mol) is sufficient to form enough ·OH for the complete removal of SDAs in the samples at pH 3 (Fig. 5). However, as in the case of H2O2, too much Fe3+ will cause a very rapid increase in pressure and partly damage the nanozeolite framework, owing to the drastic decomposition of H2O2. Moreover, the residual Fe3+ content in the sample after the detemplation process will increase with increasing Fe3+ addition (Table S1†). Because of the ion exchange capacity of nanozeolite β after its micropores are emptied by the Fenton-like process, the Fe3+ ions can be partly exchanged into the micropores as balanced ions, which leads to residual Fe3+ ions in the fresh Fenton-treated β. However, as shown in the experimental part, all the obtained samples will experience an acid wash process using 0.05 M HCl. Thus, residual Fe3+ ions in the nanozeolite will be exchanged by H3O+, and the Fe content in the final Fenton-treated β becomes difficult to detect (Table S1†). Therefore, the influence of Fe species in the final sample is negligible.
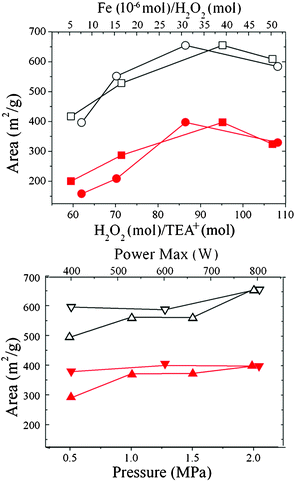 |
| Fig. 5 The influence of H2O2 (■, □) and Fe3+ (●, ○) concentration, reaction pressure (▲, △) and microwave power (▼, ▽) on the BET surface area (□, ○, △, ▽) and microporous area (■, ●, ▲, ▼) of Fenton-treated β. | |
Because the detemplation process is controlled by the system pressure, its influence is also investigated here. It is found that the SDAs can be completely removed within 10–15 min when the system pressure is fixed at 2.0 MPa (Fig. 5 and Table 1). Lowering the system pressure will slow the oxidation process of the SDAs. However, the power of the microwave oven is found to have a tiny influence on the detemplation of nanozeolite β. Most of the SDAs decomposed in 15 min at 400 W (Fig. 5 and Table 1), and no evident improvement is observed in the microporosity of the Fenton-treated samples when the microwave power is increased to 800 W. Thus, it is concluded that when the ratios of H2O2/TEA+ and Fe3+/H2O2 are up to 95.2 mol/mol and 30.9 μmol/mol, respectively, the SDAs distributed in the micropores of nanozeolite β and in solution could be drastically eliminated in 15 min at a pH of 3 and a system pressure of 2.0 MPa under microwave irradiation. In addition, as an important fact, it is worth mentioning that, if the microwave radiation is replaced by an electric oven, the SDAs cannot be completely removed even at 170 °C (about 2.0 MPa) for 120 min (Fig. S4†). This indicates that direct disturbance to organic molecules caused by microwaves can greatly improve the detemplation process. Moreover, in comparison to the calcination method, the Fenton-like process reduces the reaction time from hours to minutes, which will contribute to an improved and accelerated experimental process as well as a lower energy input.
Besides, the washed nanozeolite β is also detemplated by the same process. As shown in Table 2, it is remarkable to find that the molar ratio of H2O2/TEA+ must be up to 168.2 to achieve the complete removal of SDAs in the micropores of the nanozeolite. It is higher than the molar ratio of H2O2/TEA+ (95.2 mol/mol), which we discussed for the as-prepared nanozeolite β system without any washing. The Fe3+ ion content also needs a little increase. Clearly, compared to organic molecules dispersed in the solution, SDAs encaged in micropores are harder to remove, and a higher concentration of ·OH is needed to fully eliminate them. The desired detemplation conditions for these two different systems are summarized in Table 2.
Table 2 Summary of desired detemplation conditions of the as-prepared and washed nanozeolite β systems under microwave irradiation
Method |
H2O2/TEA+ (mol/mol) |
Fe3+/H2O2 (10−6 mol/mol) |
Timec (min) |
As-prepared β nanozeolite system.
Washed β nanozeolite system.
Max power of microwave is fixed at 800 W.
|
1a |
87.0–107.0 |
25.8–41.6 |
15 |
2b |
168.2–201.5 |
10.3–61.8 |
5–10 |
Furthermore, we try to relate the amounts of H2O2 we used to the stoichiometry demanded for the total oxidation of the SDAs in the system. The formation of ·OH can be described as eqn (6), which can be obtained by the reaction mechanism of Fenton-like oxidation [(1) + 2 × (2) + (3)].
|  | (6) |
The complete removal of TEA+ (C2H5)4N+) in the presence of ·OH in our work can be written as eqn (7).
| (C2H5)4N+ + 51·OH → 8CO2 + 0.5N2 + 35H2O + H+ | (7) |
Therefore, a total equation [51/2 × (6) + (7)] of the detemplation process can be described as eqn (8).
|  | (8) |
As eqn (8) shows, the elimination of one TEA+ molecule theoretically needs 76.5 molecules of H2O2. Taking this and the data of Table 2 into consideration, we can conclude that the efficiency of the Fenton-like oxidation method under microwave irradiation is very high in our system. For the as-prepared nanozeolite β system, 80–85% of the H2O2 is used in the detemplation process. Even for the washed nanozeolite β system, about 45% of the H2O2 is still consumed by TEA+. Because the SDAs (TEA+) embedded in the micropores are difficult to approach, a mass of ·OH produced within a short time is thereby quenched due to its instability. Therefore, such Fenton-like treatment conditions are very relevant to the detemplation of other porous materials, while the detailed processes and conditions have to be slightly regulated according to the different materials.
Catalytic study
The catalytic performance of Fenton-treated β is explored by the dehydration of fructose into 5-hydroxymethyl furfural (5-HMF), a very important reaction for biomass conversion. Calcined β is used as a reference here. As shown in Fig. 6, both catalysts show similar conversion of fructose, but the reaction catalyzed by Fenton-treated β presents a higher reaction selectivity, i.e. higher 5-HMF yield. The short active channel and good monodispersity of Fenton-treated β nanoparticles shorten the diffusion route for guest molecules, and so decrease the secondary reactions and formation of coke.19
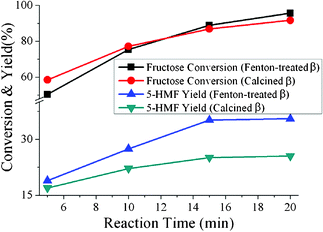 |
| Fig. 6 Conversion and yield of fructose dehydration to 5-HMF catalyzed by Fenton-treated β and calcined β in aqueous solution at different reaction times under microwave irradiation. | |
Conclusions
In summary, the microwave-assisted Fenton-like oxidation method is proven to be effective for the rapid detemplation of as-prepared nanozeolites β. Such a Fenton-like process can significantly reduce the detemplation time to 10–15 min and avoid the traditional calcination process as well as its destruction for the structure and composition of nanozeolites β. The influence factors are discussed in order to deeply understand the detemplation processes. And the optimum conditions are proposed to drastically eliminate SDAs within 15 min under microwave irradiation. Moreover, the good intrinsic framework structure and monodispersity of the nanozeolite preserved by Fenton-like oxidation promise good catalytic behavior in fructose dehydration.
Acknowledgements
This work is supported by NSFC (21171041, 20873025 and 20890122), STCSM (10QH1400300, 08DZ2270500 and 09DZ2271500), 973 programs (2009CB930400, 2009CB623506).
References
-
(a) M. Sugimoto, H. Katsuno, K. Takatsu and N. Kawata, Zeolites, 1987, 7, 503 CrossRef CAS;
(b) M. Yamamura, K. Chaki, T. Wakatsuki, H. Okado and K. Fujimoto, Zeolites, 1994, 14, 643 CrossRef CAS;
(c) G. Sartori and R. Maggi, Chem. Rev., 2006, 106, 1077 CrossRef CAS.
-
(a)
D. W. Breck, Zeolites and Molecular Sieves System, Wiley, New York, 1974 Search PubMed;
(b)
R. M. Barrer, Hydrothermal Chemistry in Zeolites, Academic Press, London, 1982 Search PubMed;
(c)
T. O. Do and S. Kaliaguine, Nanoporous Materials Science and Engineering, Imperial College Press,London, 2004 Search PubMed.
-
(a) V. Valtchev and L. Tosheva, Chem. Mater., 2005, 17, 2494 CrossRef;
(b) B. J. Schoeman, J. Sterte and J. E. Otterstedt, Zeolites, 1994, 14, 110 CrossRef CAS.
- M. Muller, G. Harvey and R. Prins, Microporous Mesoporous Mater., 2000, 34, 135 CrossRef CAS.
- I. Melian-Cabrera, F. Kapteijn and J. Moulijn, Chem. Commun., 2005, 21, 2744 RSC.
- T. Masuda, S. Otani, T. Tsuji, M. Kitamura and S. Mukai, Sep. Purif. Technol., 2003, 32, 181 CrossRef CAS.
-
(a) S. Heng, P. Lau, K. Yeung, M. Djafer and J. Schrotter, J. Membr. Sci., 2004, 243, 69 CrossRef CAS;
(b) J. Kuhn, M. Motegh, J. Gross and F. Kapteijn, Microporous Mesoporous Mater., 2009, 120, 35 CrossRef CAS.
- A. Parikh, A. Navrotsky, Q. Li, C. Yee, M. Amweg and A. Corma, Microporous Mesoporous Mater., 2004, 76, 17 CrossRef CAS.
- T. Maesen, H. Kouwenhoven, H. van Bekkum, B. Sulikowski and J. Klinowski, J. Chem. Soc., Faraday Trans., 1990, 86, 3967 RSC.
- G. A. Tompsett, W. C. Conner and K. S. Yngvesson, ChemPhysChem, 2006, 7, 296 CrossRef CAS.
- C. O. Kappe, Angew. Chem., Int. Ed., 2004, 43, 6250 CrossRef CAS.
- Y. Y. Hu, C. Liu, Y. H. Zhang, N. Ren and Y. Tang, Microporous Mesoporous Mater., 2009, 119, 306 CrossRef CAS.
-
L. J. Bellamy, The Infrared Spectra of Complex Molecules, Chapman and Hall, London, 1975 Search PubMed.
- I. M. Cabrera, F. Kapteijn and J. A. Moulijn, Chem. Commun., 2005, 2178 Search PubMed.
- A. E. W. Beers, J. A. van Bokhoven, K. M. de Lathouder, F. Kapteijn and J. A. Moulijn, J. Catal., 2003, 218, 239 CrossRef CAS.
-
(a) K. N. Rao, K. M. Reddy, N. Lingaiah, I. Suryanarayana and P. S. Prasad, Appl. Catal., A, 2006, 300, 139 CrossRef CAS;
(b) A. S. Khder and A. I. Ahmed, Appl. Catal., A, 2009, 354, 153 CrossRef CAS.
- R. Cid and G. Pecci, Appl. Catal., 1985, 14, 15 CrossRef CAS.
- F. J. Rivas, F. J. Beltran, O. Gimeno and J. Frades, J. Agric. Food Chem., 2001, 49, 1873 CrossRef CAS.
-
(a) R. Loenders, P. A. Jacobs and J. A. Martens, J. Catal., 1998, 176, 545 CrossRef CAS;
(b) Y. Shi, X. Li, J. K. Hu, J. H. Lu, Y. C. Ma, Y. H. Zhang and Y. Tang, J. Mater. Chem., 2011, 21, 16223 RSC;
(c) X. Li, Y. Shi, Z. J. Wang, Y. H. Zhang and Y. Tang, J. Catal., 2012, 288, 24 CrossRef CAS.
Footnote |
† Electronic Supplementary Information (ESI) available: Detailed calculation of H2O2/TEA+ and Fe3+/H2O2 for as-prepared β and washed β nanozeolites. Fe/Si ratios in Fenton-treated β with different compositions before and after HCl wash step. SEM image, XRD patterns and MS signals of as-prepared β, Fenton-treated β and calcined β. FTIR spectrum of Fenton-treated β heated by oven. See DOI: 10.1039/c2ra00947a/ |
|
This journal is © The Royal Society of Chemistry 2012 |