DOI:
10.1039/C2RA00217E
(Paper)
RSC Adv., 2012,
2, 2128-2136
Kinetic characteristics of enhanced photochromism in tungsten oxide nanocolloid adsorbed on cellulose substrates, studied by total internal reflection Raman spectroscopy†
Received
25th May 2011
, Accepted 1st December 2011
First published on 18th January 2012
Abstract
The nanostructured tungsten(VI) oxide (WO3)/cellulose derivatives (cellulose (CE) and triacetyl cellulose (TACE)) hybrid films were prepared by a solution-dipping adsorption process, and their structure and optical properties have been investigated. Various techniques, including adsorption isotherm, transmission electron microscopy (TEM), X-ray diffraction (XRD), atomic force microscopy (AFM), energy-dispersive X-ray spectroscopy (EDX), in situUV-Vis absorption, and in situ total internal reflection Raman spectroscopy, were used for the characterization of the WO3/CE and WO3/TACE hybrid materials. Under UV irradiation, the photochromism (colorless → blue) was confirmed from the WO3/CE hybrid film, although no coloration of the WO3/TACE hybrid film was observed. This distinct difference in the coloration suggested that the interfacial interaction between hydroxyl groups present on the surface of the CE substrate and WO3 nanoparticlesviahydrogen bonding plays a major role in the enhancement of photochromism in the WO3/CE hybrid system. Moreover, the joint evidence in in situUV-Vis absorption and in situ total internal reflection Raman studies clearly revealed that the photogenerated coloration is related to a partial reduction of W6+ cations into W5+ cations in the WO3/CE hybrid film. The findings in this study have great implications for the development of the novel green-functional inorganic/organic hybrid materials in optical devices.
1. Introduction
Metal oxide semiconductors (MOSs) are able to be considered as inorganic polymer complexes with oxygen bridges, which can form donor–acceptor complexes with various kinds of electron donors and electron acceptors.1 MOSs have caused particular attention for their applications in many fields of science, such as catalysis, medicine, and photoelectricity, owing to their special structures and properties.2–4 Especially, a chromogenic phenomenon of MOSs is important in an optoelectronic technology.5 Despite their potential applications, MOSs are difficult to manipulate into practical chromogenic devices (i.e., electrochromic, gaschromic, photochromic, and thermochromic devices) for which low response speed, fatigue, and high cost for manufacture are most often criticized. Recently, many articles have been published on the hybridization of MOS nanomaterials and polymeric matrix (such as poly(acrylamide),6 poly(vinyl pyrrolidone),7 poly(vinyl alcohol)8 and poly(ethyleneimine)9) to form charge-transfer composite via chemical bonding and/or hydrogen bonding, which can effectively improve physical, chemical stability, and chromogenic capability, and open new avenues to functional inorganic/organic hybrid materials. However, when disposing by incineration, these hybrid materials may bring about environmental issues. Consequently, there are growing demands for environmentally friendly composites.
Interest in studying and utilizing biologically derived materials has lately risen due to advances in fields such as polymer technology and biotechnology and their possible use as alternative sources for biofuel.10 Among the many type of biomaterials, cellulose is the most abundant natural homopolymer on earth, and it plays a significant role in the structural support of plant cell walls because of its high mechanical properties.11 Cellulose and its derivatives have been explored as an organic matrix for inorganic/organic hybrid materials because of the presence of several functional groups that can be employed in various activation processes.12–14 The incorporation of nanoscale inorganic particles into a cellulose matrix led to a specific inorganic/organic interfacial interaction via weak non-covalent bonding (i.e., ionic bonds, hydrogen bonds, van der Waals forces). In addition, the biodegradable property of cellulose has brought about the new trend toward eco-friendly inorganic/organic hybrid materials.15
Hence, we focused on the hybridization of nanostructured tungsten(VI) oxide (WO3) and cellulose derivatives using a solution-dipping adsorption technique to prepare the novel eco-functional inorganic/organic hybrid materials. In this context, the adsorption means the formation of non-covalent bonds between WO3 nanoparticles and the functional groups present on the surface of cellulose derivatives. Two cellulose derivatives, containing cellulose (CE) and triacetyl cellulose (TACE), were chosen in this study. On the other hands, WO3 is one of the important n-type MOSs with a wide band-gap (Eg ∼ 3.3 eV), high chemical and thermal stability, and thus has been widely investigated as photocatalysts and photoelectrodes for visible-light utilization.16 In addition, there has been a great deal of recent interest in the WO3 compounds for the development of smart windows for energy-efficient architecture of buildings and automobiles, flat-panel displays, optical memory and writing-reading-erasing.17,18 Herein, the adsorption characteristics of WO3 colloid particles on the CE and TACE and the kinetics of the photochromic reaction in the WO3/CE and WO3/TACE hybrid film system have been systematically investigated by means of adsorption isotherm, XRD, AFM, EDX, in situUV-Vis absorption, and in situ total internal reflection Raman spectroscopies. The findings in this study will propose a new design of MOS/cellulose derivative hybrid photochromic materials.
2. Experimental
2.1. Materials
Sodium tungstate(VI) dihydrate (Na2WO4·2H2O), concentrated hydrochloric acid (conc. HCl) (Analytical Reagent, Wako Pure Chemical, Osaka, Japan), and dialytic membranes with 3500 dialytic modulus (Spectrum Laboratories, CA, USA) were used to prepare tungsten(VI) oxide (WO3) nanocolloids. Microcrystalline cellulose (CE) and triacetyl cellulose (TACE) were obtained from Kanto Chemical Co., Inc. (Tokyo, Japan). Trifluoroacetic acid, chloroform, ethanol, and n-pentane were purchased from Nacalai Tesque Inc. (Kyoto, Japan). The water used for all sample preparation was first distilled and then passed through a Milli-Q system (Millipore, USA), resulting in the specific resistivity of 18.2 MΩcm. All other chemicals were of reagent grade from Tokyo Chemical Industry Co., Ltd. (Tokyo, Japan). All chemicals were used as received without further purification.
In following the procedure of Thode et al.,19solvent displacement was carried out to make the fine surface of CE and TACE available for nitrogen gas adsorption as follows. (i) CE and TACE powder samples were swollen and macerated in dried ethanol; the mixture was shaken and centrifuged. (ii) The operation was repeated ten times. (iii) Changing the solvent from ethanol to n-pentane, the same operation was repeated a further ten times. (iv) The CE and TACE samples dispersed in n-pentane were finally dried in vacuo using a cold trap for 24 h. (v) Dried CE and TACE powder samples (ca. 0.5 g) were outgassed with helium flow for 2 h at 200 °C prior to the adsorption measurement. Nitrogen adsorption isotherm was measured at –195.8 °C with a Gemini V2375 analyzer (Shimadzu, Japan). The Brunauer-Emmett-Teller (BET) method was utilized to calculate the specific surface area. By using the Barrett-Joyner-Halenda (BJH) model, the pore size distributions were derived from the adsorption isotherms.
2.3. Preparation of WO3 nanocolloids
The preparation procedure of WO3 colloids is similar to that described by Zou et al.20 Na2WO4·2H2O (100 g, 0.3 mol) was dissolved in 100 mL of water. Conc. HCl (7 mL, 0.7 M) was added dropwise into the solution with efficient stirring. Eventually, a colorless transparent aqueous WO3 colloid solution (pH 3.3, 0.023 M WO3) was obtained, which was then closed in a dialytic membrane pipe and dialyzed in a 1000 mL beaker containing Milli-Q water for a period of 8 h. The Milli-Q water was periodically replaced until chloride ions could not be detected by ion chromatography. The concentration of chloride ion in the WO3 colloid solution was determined by using portable-type IC analyzer (PIA-1000, Shimadzu, Japan) equipped with an anion-exchange Shim-pack IC-A3 column (Shimadzu, Japan) at 30 °C. The eluent used in this study was 4-hydroxybenzoic acid (8.0 × 10−3 M)/bis(2-hydroxyethyl)iminotris-(hydroxymethyl)methane (Bis-Tris; 3.2 × 10−3 M), and the flow rate was 300 μL min−1.
2.4. Adsorption experiments of WO3 nanocolloid onto CE and TACE
The adsorption of the WO3 nanocolloid onto the CE and TACE was investigated in batch equilibrium experiments. The stock solution of the WO3 nanocolloid (1.0 × 10−2 M) was prepared using the obtained solution, in distilled water. The pH of the WO3 nanocolloid stock solution was kept at 4.0. Because the pI value of tungsten(VI) oxide is 0.2–0.5,21 the WO3 colloid surface is negatively charged in this aqueous solution. The experiments were carried out in 100 mL glass bottles containing 50 mL of the WO3 nanocolloid solutions of different concentrations and 0.100 g of the CE or TACE powder. The ionic strength was kept at 0.1 M with sodium sulfate in all experiments. As a blank, the WO3 nanocolloid solution was added into the bottle without addition of the CE or TACE powder. The change in the pH after addition of the CE and TACE powder sample into WO3 nanocolloid solutions was negligible. These bottles were hermetically sealed with a polyethylene cap and a PTFE seal, and then left to stand for 8 h in a room thermostated at 25 °C. Eight-hours standing was confirmed to be sufficient for the adsorption equilibration. After equilibration, the supernatant of the solution was withdrawn for determination of the tungsten concentration, using inductively coupled plasma atomic emission spectroscopy (ICP-AES; Liberty Series II, Varian, USA). The concentration of WO3 nanocolloids adsorbed on the CE and TACE was calculated using the following equation (eqn(1)).
|
| (1) |
where [WO
3]
ad is the concentration of WO
3 adsorbed per unit area of the adsorbent (mol dm
−2); [WO
3]
ini and [WO
3] are the concentrations of WO
3 in the initial solution (mol dm
−3) and after adsorption, respectively.
S and
V are the total surface area of the WO
3 colloid (calculated using BET specific area value) and the volume of the aqueous phase, respectively.
2.5. Preparation of transparent WO3/CE and WO3/TACE hybrid films
Trifluoroacetic acid and chloroform were used as the co-solvent for CE22 and TACE,23 respectively, for preparation of the colorless-transparent films. The sequence for dissolution in the co-solvent and preparation of the films was the following. Although TACE chloroform solution was able to be obtained without difficulty, in the case of CE, it took approximately 2 days for complete dissolution in trifluoroacetic acid at room temperature without stirring. Both solutions were then diluted to control the viscosity for casting and to avoid the formation of foam in the film during evaporation of the solvents. After casting the solution on the flat glass plate, the solvents were removed by natural evaporation at room temperature. Colorless-transparent CE and TACE films can be obtained by peeling them off from the glass plate. They were then washed with water, and dried by being placed between a stainless metal plate and a filter paper at room temperature, for approximately 16 h.
The CE and TACE film substrates were dipped in a 30 mL glass vial containing 25 mL of the 0.1 M WO3 nanocolloid solution (pH 4) in a room thermostated at 25 °C for 1 h to obtain WO3/CE and WO3/TACE hybrid films. The dipping CE and TACE substrates were withdrawn vertically from the WO3 nanocolloid solution in the vial at a speed of 40 mm min−1 at room temperature. The prepared hybrid films were stored under dried conditions (desiccator) at room temperature before testing.
2.6.
In situ observation on photochromic coloration of WO3/CE and WO3/TACE hybrid films
The photochromic coloration of the WO3/CE and WO3/TACE hybrid films under the black light irradiation (4 W × 4, the wavelength range of ca. 320–400 nm, λmax = 366 nm) were measured by an in situ single fiber absorption measurement technique. The fiber optic arrangement is shown in Fig. 1(a). For single fiber absorption measurement, collimated light beam of a tungsten halogen light source (20 W; DH-2000, Ocean Optics, USA) through an optical fiber was incidented into the hybrid film sample from downside, and the transmitted light was then collected with the upside condenser lens and introduced to the spectrometer (SEC2000-UV/VIS, BAS Inc., Japan) through an optical fiber. A reference spectrum was measured using CE and TACE films without WO3 nanocolloid in the synthetic quartz (SQ) optical glass sample holder. The background absorbances due to the SQ sample holder, CE, and TACE films were subtracted from the total absorbance to get the corrected photochromic spectrum. All measurements were performed in a thermostated room at 25 ± 1 °C.
2.7.
In situ total internal reflection raman measurement of WO3/CE and WO3/TACE hybrid films under UV irradiation
The Raman spectral measurements of the WO3/CE and WO3/TACE hybrid films under the black light irradiation (4 W × 4, the wavelength range of ca. 320–400 nm, λmax = 366 nm) were carried out using a Raman microscope apparatus (Photon Design, Japan) shown in Fig. 1(b). A continuous-wave argon ion laser (457.9 nm, Stabilite2017, Spectra-Physics, Inc., USA) irradiated the SQ optical glass/CE or TACE hybrid interface with an angle of 80°. Since this angle was larger than the critical angle of SQ optical glass/cellulose (ca. 77°), the laser was totally reflected at the interface and only the evanescent region inside the CE or TACE hybrid film (∼100 nm) was irradiated. Therefore, the total internal reflection Raman technique can provide the specific information relating the electronic state of WO3 colloid particles adsorbed on the cellulose derivative substrates. The upside objective (10×, NA 0.25) was used to collect the Raman scattered light. The scattered light was passed through a notch filter and a spatial filter and then introduced to the spectrometer (HR-320, Jobin Yvon, USA) equipped with a liquid nitrogen cooled CCD detector (LN/CCD-1100-PB/UVAR/1, Roper Scientific, USA). The detected area was limited with the spatial filter to cut the reflected light or stray light. We also analyzed the difference Raman spectra obtained by subtracting the spectrum of the hybrid film before UV irradiation from that after UV irradiation. All measurements were performed in a thermostated room at 25 ± 1 °C.
2.8. Other apparatus
The TEM images of WO3 colloids were measured with a JEM-2000EX II instrument (JEOL, Japan). The TEM samples were prepared by dropping the WO3 colloid sample solution onto a copper grid covered with a carbon film. Each size distribution histogram of WO3 colloids was obtained by averaging the sizes of 100 particles directly from the TEM images.
The dried WO3 colloids were analyzed by X-ray diffraction (XRD, RINT-2500, Rigaku, Japan) with Cu-Kα radiation (40 kV, 100 mA) from 2θ = 5° to 60° with a scan speed of 4° min−1.
The absorption spectrum of the as-prepared WO3 colloid solution was obtained using a UV-visible spectrophotometer (UV-2500, Shimadzu, Japan)
The WO3/CE and WO3/TACE hybrid films were imaged with contact-mode AFM (SPA-400, Seiko Instruments Industry, Japan) to determine the morphology and surface roughness. Topographic (height) and phase images were recorded under ambient air conditions (23 °C and 50% relative humidity).
Energy-dispersive X-ray (EDX) spectra were obtained on a scanning electron microscope (S4100, Hitachi, Japan) coupled with an energy dispersive spectrometer and operating at 25 kV, using non-metal coated hybrid film samples.
3. Results and discussion
3.1. Characterization of as-prepared WO3 colloids, CE, and TACE
Fig. S1 (see ESI†) shows the bright-field TEM image of typical samples of the as-prepared WO3 colloid and the corresponding size distribution histogram. As can be seen in Fig. S1, most of the WO3 particles except for some agglomerate are spherical in shape. The corresponding size distribution histogram shows that the size of the as-prepared WO3 colloids is 6–32 nm with an average diameter of 16.8 nm.
A typical XRD pattern of dried WO3 colloids is shown in Fig. S2 (see ESI†). Except for the small reflection at 2θ = ca. 17° (probably due to WO3·H2O), all the reflections in the figure can be indexed to the WO3·2H2O layered structure that has lattice constants a = 10.484 Å, b = 13.804 Å and c = 10.573 Å,24 which are consistent with the values in the standard card (JCPDS 18–1419, see Fig. S2 below). It has been proposed that the WO3·2H2O crystal consists of corner-sharing [WO6] octahedra moieties bound to each other through water bridges, hydrogen bonding, and van der Waals forces. Furthermore, it can be seen that the diffraction peaks are relatively narrow, implying that the WO3·2H2O crystallizes well. The crystallite size measurements were calculated employing Debye–Scherrer analysis;25
|  | (2) |
where
D is the crystallite size,
k is a Scherrer constant (= 0.9 assuming that the particles are spherical),
λ is the wavelength of the X-ray radiation, β is the line width (obtained after correction for the instrumental broadening), and
θ is half of the measured diffraction angle. The average crystallite size of dried WO
3 colloid samples from
XRD data was about 16 nm.
Fig. S3 (see ESI†) shows UV-visible light absorption spectrum of the as-prepared WO3 colloid solution. The as-prepared WO3 colloid solution exhibited an absorption edge starting from around 385 nm, but had no absorption in the longer wavelength range. For WO3 semiconductor, the optical band gap value can be determined by considering an indirect transition between the 2p electrons from the valence band of the oxygen and the 5d conduction bands of tungsten.26 Using a Tauc plot (Fig. S3† inset) which shows the square root of the absorption intensity multiplied by photon energy as a function of photon energy, the band gap energy of the as-prepared WO3 colloid was determined as to be 3.21 eV, corresponding to an indirect band gap of crystalline WO3·2H2O.27
Fig. 2(a) shows the nitrogen adsorption isotherms for the CE and TACE powders. From the figure, it is obvious that less nitrogen is adsorbed with TACE. No leveling off of the volume adsorbed at high relative pressures was observed, and both isotherms could be essentially regarded as type II based on Brunauer's classification.28 Such isotherms usually have no or very narrow hysteresis between adsorption and desorption isotherms arising from V-shaped or conical pores (their diameters greater than approximately 50 nm).29 Indeed, both BJH pore-size distribution charts show a uniform distribution, centered at ca. 30–40 nm (see Fig. 2(b)). It should be noted that the pore diameter in the CE and TACE powders is larger than the size of dried WO3 colloids (ca. 17 nm), suggesting that the WO3 colloid particles would fully access to the macropores of the CE and TACE powders in an aqueous system. The calculated BET specific areas of the CE and TACE powders are listed in Table 1.
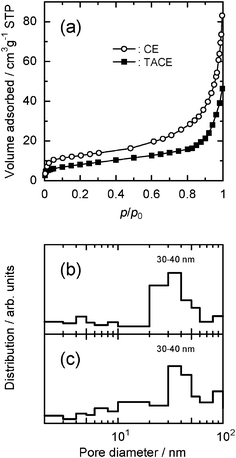 |
| Fig. 2 (a) Nitrogen adsorption isotherms at −195.8 °C for the dried CE and TACE powders. The corresponding pore size distribution histograms for (b) CE and (c) TACE. | |
Table 1 BET surface area of CE and TACE powder, saturated surface concentrations (a), and adsorption constants (Kad) of WO3 colloids onto CE and TACE powder at 25 °C
|
BET surface area/m2 g−1 |
a/mol dm−2 |
K
ad/dm |
CE |
42.4 |
(4.63 ± 0.39)× 10−7 |
(2.63 ± 0.23) × 10−4 |
TACE
|
28.6 |
(4.31 ± 0.33) × 10−7 |
(0.94 ± 0.15) × 10−4 |
3.2. Adsorption behavior of WO3 colloids onto CE and TACE powders
Fig. 3 shows the adsorption isotherms of the WO3 colloid particles on the surface of CE and TACE powders in the aqueous solution at pH 4. The adsorption behavior of the WO3 nanocolloid varied remarkably with the kind of cellulose derivative substrates. To access the substituent effect of substrates, the isotherm data could be analyzed by a Langmuir adsorption equation.
![Adsorption isotherms of the WO3 colloid particles on the surface of CE and TACE powders in the aqueous solution. Concentration conditions: [CE] and [TACE] = 0.1 g/50 mL, [WO3] = 1.0 × 10−4–1.0 × 10−2 M, [Na2SO4] = 0.033 M, pH 4. The solid lines are fitted by eqn (3).](/image/article/2012/RA/c2ra00217e/c2ra00217e-f3.gif) |
| Fig. 3 Adsorption isotherms of the WO3 colloid particles on the surface of CE and TACE powders in the aqueous solution. Concentration conditions: [CE] and [TACE] = 0.1 g/50 mL, [WO3] = 1.0 × 10−4–1.0 × 10−2 M, [Na2SO4] = 0.033 M, pH 4. The solid lines are fitted by eqn (3). | |
|
| (3) |
where
a and
Kad refer to the saturated surface concentration and the adsorption constant onto the cellulose substrate surface, respectively. Herein, the
Kad is defined as follows:
| 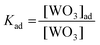 | (4) |
The lines drawn in Fig. 3 are the results calculated by eqn (3) using the a and Kad values determined from the least-squares method. The determined a and Kad values are also summarized in Table 1. While both values of a are the almost same, the Kad value for the CE substrate is about three-times larger than that for the TACE substrate, indicating the high affinity between the WO3 nanoparticles and the CE surface. Indeed, many researchers have concluded that cellulose, hemicellulose, lignin, etc., containing hydroxyl (–OH) groups, can more strongly bind to metal ions than the corresponding acetyl-modified ones.30–32 Although the difference in metal ions and metal oxide nanocolloid particles does exist, this result may explain that the hydroxyl groups can more easily form non-covalent interactions with the WO3 colloid particles than the acetyl ones.
3.3 Characterization of WO3/CE and WO3/TACE hybrid films
The WO3/CE and WO3/TACE hybrid films were characterized by AFM, EDX, and total internal reflection Raman spectroscopy. The AFM and EDX data of WO3/CE and WO3/TACE hybrid films are shown in Fig. 4. As references, AFM and EDX data of as-prepared CE film are displayed in this figure. All AFM images were recorded with an area of 500 × 500 nm2. The AFM images of as-prepared CE (Fig. 4(a)) and TACE (not shown) film indicate that both films have a relatively smooth surface without pores, cracks, or particulates, which is important to recognize because WO3 colloid adsorption layers usually exhibit particulates. Somewhat sharp tips on the as-prepared CE surface are probably due to a cellulose fibrillar network structure.33AFM images of the WO3/CE (Fig. 4(b)) and WO3/TACE (Fig. 4(c)) hybrid films are almost similar and indicate the agglomeration of small grains, 10–30 nm in size, spreading uniformly on the film surface. Additionally, the average roughness of the WO3/CE and WO3/TACE hybrid film surface was 18.1 nm and 17.6 nm, respectively. These results are similar to the TEM result one described above. The EDS analysis of the as-prepared CE (Fig. 4(d)) and TACE (not shown) film demonstrates that only carbon (C) and oxygen (O) elements are detected. Meanwhile, the WO3/CE and WO3/TACE hybrid films contain elements of C, O, and tungsten (W), as the EDX spectra are shown in Fig. 4(e) and (f), respectively. The C and most O belong to the CE and TACE film substrate, and the W should be assigned to the WO3 nanocolloid particles adsorbed on the film substrates. From the XRD result of dried WO3 colloid (see Section 3.1), WO3 colloid particles adsorbed on CE and TACE substrates are more likely to WO3·2H2O crystallites, though the appropriate evidence is not provided at present.
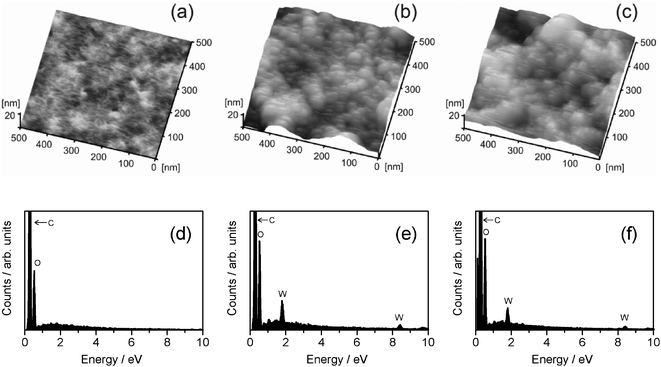 |
| Fig. 4 Topographical AFM images of (a) as-prepared CE film, (b) WO3/CE hybrid film, and (c) WO3/TACE hybrid film. The corresponding EDX spectra of (d) as-prepared CE film, (e) WO3/CE hybrid film, and (f) WO3/TACE hybrid film. | |
Fig. 5 shows the total internal reflection Raman spectra of the CE, TACE, WO3/CE, and WO3/TACE hybrid films. As references, Raman spectrum of dried WO3 powder is also displayed in this figure. In the Raman spectrum of the dried WO3 powder (spectrum A), a broad peak at around 670 cm−1 is due to the O–W6+–O single bonds.34,35 There is also a relatively sharp peak at around 990 cm−1, which has been assigned to the stretching mode of the W6+
O double bonds involving terminal oxygen atoms on the surfaces of colloid particles.34,35 Additionally, detailed peak assignments of the CE and TACE main absorption bands are listed in Table S1 (see ESI†). These band assignments were made according to standard spectroscopy literature and by considering previous Raman studies of various cellulose derivatives.36 As compared with spectra A and E in Fig. 5, two Raman bands due to WO3 colloids adsorbed on CE film shift to lower frequencies (red-shifts) compared to the dried WO3 powder. Raman spectroscopy is more sensitive to the slight change of metal–O bond lengths, that is, a metal–O bond length can be correlated to its Raman stretching frequency.37 The red-shift of the two O–W6+–O and W6+
O bond peaks reveals a decreasing bonding energy and increasing bond distance of the bond between W and O atoms in the lattice network. Therefore, this fact suggests that a more open WO3 structure is formed with lower internal stress on the CE substrate. On the other hand, it is noted here that the Raman bands of WO3 in the WO3/TACE hybrid system seem to be unshifted, even though the Raman band of O–W6+–O bridgings at approximately 673 cm−1 may interfere with that of O–C
O vibrations at 659 cm−1 (see spectra A and C in Fig. 5). This difference may be caused by the formation of hydrogen bonds between –OH groups in the cellulose skeleton and WO3 colloid particle. Unfortunately, the Raman spectrum of WO3/CE hybrid film did not exhibit a clear profile in the 3500–3000 cm−1 range originating from –OH groups of CE substrate (not shown), because the intensity of these modes is quite weak in Raman spectra. However, it is well-known that all of the W6+
O and O–W6+–O modes are sensitive to hydrogen-bonding interaction.38 Additionally, the high affinity between the WO3 nanoparticles and the CE surface in the adsorption experiment described in previous Section 3.2 would also be one of the arguments which favour the formation of hydrogen bond between WO3 and CE. Much further work is required to clarify this hydrogen-bonding effect on the adsorption phenomena of WO3 colloid particles onto the cellulose substrates. The local structure variations of WO3 colloid could influence the photochromic behaviors between the WO3/CE and WO3/TACE hybrid films.
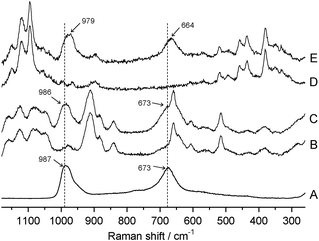 |
| Fig. 5
Raman spectra of (A) dried WO3 colloids, (B) TACE, (C) WO3/TACE hybrid film, (D) CE, (E) WO3/CE hybrid film. For peak assignments of CE and TACE, see Table S1 (ESI†). | |
These AFM, EDX, and Raman results reveals that the WO3 colloid particles are adsorbed on the CE and TACE film surfaces under the hybrid film preparation condition employed in this study.
3.4
In situ
UV-Vis absorption and total internal reflection Raman spectral investigation of photochromism process of WO3/CE hybrid film
At first, the preliminary experiments were carried out by batch method to check the photochromism of WO3/CE and WO3/TACE hybrid films. The hybrid film specimen (3 cm × 5 cm) was irradiated by weak UV light from a black light bulb (15 W, 10 cm away from the specimens) for 30 min. The wavelength of the most intense line was 366 nm. A distinct difference in the coloration between the WO3/CE and WO3/TACE hybrid films was perceived. In this measurement condition, no coloration of the WO3/TACE hybrid film was observed. The photochromism (colorless → blue) was confirmed only from the WO3/CE hybrid film. Since no coloration was found in the dried WO3 powder under the same UV irradiation condition, this difference should be emphasized as “the specific behavior observed only for the adsorbed state of the WO3 colloid on CE substrate” and will be discussed in a later section of this paper. The following in situ measurements of UV-Vis and total internal reflection Raman spectra are limited for the WO3/CE hybrid system.
Fig. 6 shows the in situUV-Vis spectral changes of the WO3/CE hybrid film as a function of the elapse time of UV irradiation. The absorbance of the WO3/CE hybrid film in the region above 500 nm increased. It is characteristic of colored, reduced molecular species (heteropoly blues), with d–d band charge transfer intervalence transitions (W5+ → W6+) in the visible region.39 In order to investigate the coloration behavior of the WO3/CE hybrid film under UV irradiation, change in absorbance at the absorption maximum wavelength (640 nm) was analyzed. As shown in Fig. 6(b), this coloration of the WO3/CE hybrid film gave the greatest absorption in the time of 60 s, nearly reaching saturation. After the UV irradiation was turned off, the blue WO3/CE hybrid film began to gradually discolor in air. However, this bleaching speed is very low and it needs about 2 h to reach complete decoloration (not shown). The inset in Fig. 6(b) shows the good reversibility of the coloration–decoloration cycling of the WO3/CE hybrid film. The absorption spectra of the completely bleached films are consistent with that of the film without UV irradiation. Therefore, the UV irradiated WO3/CE hybrid film can be bleached and recover their initial state.
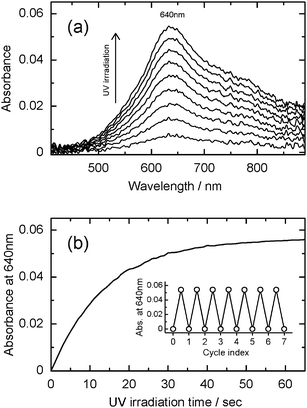 |
| Fig. 6 (a) Absorption spectral change of the WO3/CE hybrid film system upon UV irradiation. The absorption spectra were recorded at 1 s intervals until the equilibrium was attained. (b) The corresponding time profile of the absorbance at the maximum wavelength (640 nm). The inset shows its reversibility in the coloration–decoloration process at 640 nm. | |
Fig. 7 shows the in situ total internal reflection Raman spectra of the WO3/CE hybrid film in the coloring process. UV irradiation of the film caused the two Raman bands (664 and 979 cm−1 due to the O–W6+–O and W6+
O bonds, respectively) to decrease, while novel band(s) at around 360–440 cm−1 came up simultaneously. The upper part of Fig. 7 shows the difference between the first Raman spectrum and the successive ones, thus making clear that the four bands are correlated by a uniform reaction. According to previous Raman studies of tungsten compounds,40,41 two novel Raman bands at 372 and 436 cm−1 are attributed to the O–W5+–O single bonds and the W5+
O double bonds, respectively. The Raman peak at 1095 cm−1 (due to C–O–C asymmetric stretching in cellulose skeleton, see Table S1†) is very strong, fully resolved and is invariant with the extent of photochromism. It is therefore an ideal candidate as an internal standard peak. The observed temporal changes of the Raman intensity ratio (I664/I1095 and I979/I1095 for W6+ species, I372/I1095 and I436/I1095 for W5+ species) from the photochromism of the WO3/CE hybrid film were plotted in Fig. 8. As shown in the upper and middle parts of Fig. 8, I664/I1095 and I979/I1095 ratio were decreased obviously with time before 20 s, and then decreased slowly; finally, there was no change after approximately 60 s. Meanwhile, I372/I1095 and I436/I1095 ratio was increased upon UV irradiation, and saturated at a time larger than 60 s (see the lower part of Fig. 8). Although the observed intensity ratio is very different, the trend of these kinetics curves is the same in this figure. Moreover, the kinetic profiles of Raman intensity in the WO3/CE hybrid film system were in good agreement with one of the absorbance at 640 nm. This fact clearly indicates that the part of W6+ of WO3 nanocolloids adsorbed on the CE substrate is reduced to W5+ by UV irradiation, and the blue coloration is responsible for the appearance of the distinct absorption bands in the visible light region due to the charge transfer intervalence transitions between W6+ and W5+.
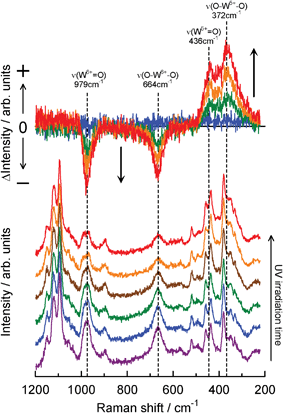 |
| Fig. 7 Total internal reflection Raman spectral change of the WO3/CE hybrid film system upon UV irradiation. Laser power was 40 mW and Raman spectra were recorded at 2.5 s intervals until the equilibrium was attained. The upper part is the difference Raman spectra obtained by subtracting the spectrum of the WO3/CE hybrid films before UV irradiation from that after UV irradiation. Changes in peaks marked are discussed in the text. | |
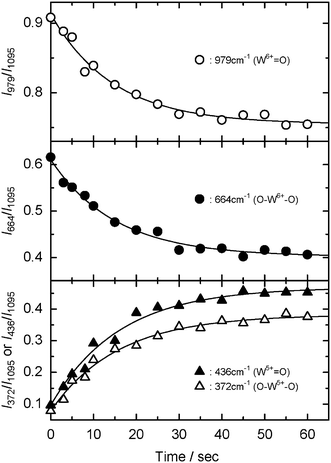 |
| Fig. 8 Time profiles of I979/I1095 (upper part), I664/I1095 (middle part), I372/I1095, and I436/I1095 (lower part) ratio of the WO3/CE hybrid film upon UV irradiation. Experimental conditions are the same with those of Fig. 7. | |
3.5 Enhanced photochromism of WO3 colloid adsorbed onto CE substrate
From the above results of adsorption isotherm following Langmuir model, TEM, XRD, AFM, EDX, UV-Vis absorption, and total internal reflection Raman spectra, we now consider the photochromism behavior of WO3 colloid adsorbed onto cellulose derivative substrates. As a typical case, we just discuss the WO3/CE hybrid film system which shows an enhanced photochromism performance. The experimental results support the following facts. (i) The WO3 colloids are predominantly WO3·2H2O crystal particles with a size of ca. 16 nm, and would be adsorbed with a monolayer format on the surface of CE film (adsorption isotherm, TEM, XRD, AFM, and EDX); (ii) The formation of hydrogen bonds between –OH groups present on the surface of the CE substrate and WO3 colloid particle causes an increase in the length of the O–W6+–O and W6+
O bond in the WO3 lattice (adsorption measurement and total internal reflection Raman); (iii) The photogenerated coloration (colorless → blue) is related to a partial reduction of W6+ cations into W5+ cations in the WO3/CE hybrid film (UV-Vis absorption and total internal reflection Raman).
Generally, when crystalline WO3·2H2O is irradiated by UV light (hν > the band gap energy (Eg)), electrons are excited to the conduction band, leaving holes in the valence band. The photogenerated holes (h+) can weaken the O–H bonds of water molecules in the WO3·2H2O and cause the water molecules to decompose into protons and highly reactive oxygen radicals. The oxygen radicals may bind to each other and be released to the ambient in molecular form. Then, the separated protons (H+) will combine with O2− in the chromogenics to form H2O with the help of photon energy. As a result, the photochromic reaction in WO3·2H2O can be expressed as eqn (5).42
|  | (5) |
Therefore, the photogenerated electron-hole pairs give rise to the reduction of transparent W6+ ions into colored W5+ ions.
Given that the displacement of hydrogen from the organic component toward the [WO6] framework enhances the photochromism under the excitation described above, it is reasonable to assume that those W6+ ions in the WO3·2H2O nanocrystal adsorbed onto the CE substrate via –OH hydrogen bonds are preferentially photoreduced. Indeed, Kuboyama et al.43 and Hwang et al.44 concluded that the WO3 photochromism-enhancing compounds have the –OH group as a common chemical structure. Moreover, according to the studies on the photochromism of molybdenum oxide (MoO3)-organoammonium hybrid materials,45 the photoexcitation in the MoO3 ligand-to-metal charge transfer (LMCT) bands results in the transfer of a hydrogen atom from an ammonium group to the bridge oxygen atom at the photoreduced site in the edge-shared [MoO6] octahedral lattice. This is followed by the interaction of one electron with the proton that was transferred to the oxygen atom. Simultaneously, the hole left at the oxygen atom as a result of the O → Mo LMCT transfer interacts with non-bonding electrons on the amino nitrogen atom to form a charge-transfer complex. Hereupon, it is noted that the hydrogen bond between the MoO3 and organoammonium is crucial for the photochromism, and the bond length of Mo–O also plays an important role in the photochemical properties.46 Furthermore, since the structures and photochromic behaviors of the hydrated oxides such as MoO3·2H2O are closely related to those of WO3·2H2O,47,48 a similar reduction step may be expected to occur in the WO3/CE hybrid film system. The illuminating evidence of enhanced photochromism in the WO3/CE hybrid system is still not known. Further research on this aspect is under way.
4. Conclusions
This study has described the fabrication and characterization of novel photochromic inorganic/organic hybrid films containing tungsten(VI) oxide (WO3) and cellulosic material components (cellulose (CE) or triacetyl cellulose (TACE)), constructed by the solution-dipping adsorption technique. The WO3 colloid particles, which consisted predominantly of WO3·2H2O crystallites, with a size of ∼16 nm, and a minor additional component of WO3·H2O, were adsorbed with a monolayer format. Detailed analysis of adsorption isotherms for WO3 colloid particles on CE and TACE substrates confirmed that CE had a higher adsorption activity than TACE. Under UV irradiation, the photochromism (colorless → blue) was confirmed from the WO3/CE hybrid film, although no coloration of the WO3/TACE hybrid film was observed. Raman spectrum of the WO3/CE hybrid film suggested that charge-transfer bridges were built at the interface of CE substrate and WO3 colloid particle viahydrogen bonding, also supporting the adsorption isotherm results. Moreover, the photochromic behavior of the WO3/CE hybrid film was investigated in situ by use of a combinational technique of optical absorption and the total internal reflection Raman spectroscopies. The joint evidence provided by in situUV-Vis and Raman results revealed that the photogenerated coloration is related to a partial reduction of W6+ cations into W5+ cations in the WO3/CE hybrid film. Thus, this work shed light on the utility of Raman spectroscopy technique not only for the analysis of individual photochromic films but also the complete functionality of the photochromic device such as smart window applications. The WO3/CE hybrid film prepared in this study exhibits extraordinary properties of high transparence in visible range, good photochromism, and reversibility. We believe that such a hybrid film system might be used to fabricate a series of photochromic transparent films by incorporating other cellulosic materials and/or photochromic metal oxides, which would represent promising materials for future applications.
Acknowledgements
This work was partly supported by the Grant-in-Aid for Scientific Research (No. 20550179) of the Ministry of Education, Culture, Sports, Science and Technology of Japan, and CASIO Science Promotion Foundation. The author (K.A.) thanks Prof. K. Akamatsu and Prof. H. Nawafune (Konan Univ., Kobe, Japan) for TEM measurements. All ICP-AES and XRD measurements documented in this report were performed at the Center for Instrumental Analysis, Yamaguchi University.
References
-
Polyoxometalate Chemistry for Nano-Composite Design; Nanostructure Science and Technology Series, ed. T. Yamase and M. T. Pope, Springer, New York, USA, 2002 Search PubMed.
- C. L. Hill and C. M. Prosser-McCartha, Coord. Chem. Rev., 1995, 143, 407–455 CrossRef CAS.
- D.-L. Long, R. Tsunashima and L. Cronin, Angew. Chem., Int. Ed., 2010, 49, 1736–1758 CrossRef CAS.
- K. Adachi, T. Mita, T. Yamate, S. Yamazaki, H. Takechi and H. Watarai, Langmuir, 2010, 26, 117–125 CrossRef CAS.
- T. He and J. Yao, Prog. Mater. Sci., 2006, 51, 810–879 CrossRef CAS.
- W. Feng, T. Zhang, J. Liu, R. Lu, Y. Zhao and J. Yao, J. Mater. Res., 2003, 18, 709–713 Search PubMed.
- X. Chen and Y. Lin, J. Sol-Gel Sci. Technol., 2005, 36, 197–201 Search PubMed.
- S. Yano, K. Kurita, K. Iwata, T. Furukawa and M. Kodomari, Polymer, 2003, 44, 3515–3522 Search PubMed.
- B. Qin, H. Chen, H. Liang, L. Fu, X. Liu, X. Qiu, S. Liu, R. Song and Z. Tang, J. Am. Chem. Soc., 2010, 132, 2886–2888 CrossRef CAS.
- M. Prabaharan, J. Biomater. Appl., 2008, 23, 5–36 CrossRef CAS.
-
J.-L. Wertz, J. P. Mercier, O. Bédué, Cellulose Science and Technology (Fundamental Sciences: Chemistry), EFPL Press, Boca Raton, USA, 2010 Search PubMed.
- S. Sequeira, D. V. Evtuguin, I. Portugal and A. P. Esculcas, Mater. Sci. Eng., C, 2007, 27, 172–179 CrossRef CAS.
- S. A. Nabi, M. Naushad and R. Bushra, Chem. Eng. J., 2009, 152, 80–87 Search PubMed.
- D. Romanov, Y. Baklagina, G. Gubanova, V. Ugolkov, V. Lavrentâev, A. Tkachenko, V. Sinyaev, T. Sukhanova and A. Khripunov, Glass Phys. Chem., 2010, 36, 484–493 Search PubMed.
- V. Polshettiwar, M. N. Nadagouda and R. S. Varma, Aust. J. Chem., 2009, 62, 16–26 CrossRef CAS.
- Z.-G. Zhao and M. Miyauchi, Angew. Chem., 2008, 120, 7159–7163 CrossRef.
- P. V. Kamat, Chem. Rev., 1993, 93, 267–300 CrossRef CAS.
- C. G. Granqvist, Sol. Energy Mater. Sol. Cells, 2000, 60, 201–262 CrossRef CAS.
- E. F. Thode, J. W. Swanson and J. J. Becher, J. Phys. Chem., 1958, 62, 1036–1039 Search PubMed.
- Y. He, Z. Wu, L. Fu, C. Li, Y. Miao, L. Cao, H. Fan and B. Zou, Chem. Mater., 2003, 15, 4039–4045 CrossRef CAS.
-
M. Kosmulski, Chemical Properties of Material Surfaces, Marcel Dekker Inc., New York, USA, 2001 Search PubMed.
- A. Isogai and R. H. Atalla, Carbohydr. Polym., 1992, 19, 25–28 Search PubMed.
- A. Sharples and F. L. Swinton, J. Polym. Sci., 1961, 50, 53–64 Search PubMed.
- K. Kalantar-zadeh, A. Vijayaraghavan, M.-H. Ham, H. Zheng, M. Breedon and M. S. Strano, Chem. Mater., 2010, 22, 5660–5666 CrossRef CAS.
- H. S. Kaufman and I. Fankuchen, Anal. Chem., 1949, 21, 24–29 Search PubMed.
- C. G. Grandqvist, A. Azens, A. Hjelm, L. Kullman, G. A. Niklasson, D. Ronnow, M. Stromme Matsson, M. Veszelei and G. Vaivars, Sol. Energy, 1998, 63, 199–216 CrossRef CAS.
- A. Wolcott, T. R. Kuykendall, W. Chen, S. Chen and J. Z. Zhang, J. Phys. Chem. B, 2006, 110, 25288–25296 CrossRef CAS.
-
S. Brunauer, The Adsorption of Gases and Vapors, Oxford University Press, Oxford, UK, 1944 Search PubMed.
- K. S. W. Sing, D. H. Everett, R. A. W. Haul, L. Moscou, R. A. Pierotti, J. Rouquerol and T. Siemieniewska, Pure Appl. Chem., 1985, 57, 603–619 CrossRef CAS.
- M. Ajmal, R. A. K. Rao, R. Ahmad and J. Ahmad, J. Hazard. Mater., 2000, 79, 117–131 CrossRef CAS.
- B. Nasernejad, T. E. Zadeh, B. B. Pour, M. E. Bygi and A. Zamani, Process Biochem., 2005, 40, 1319–1322 Search PubMed.
- M. Thirumavalavan, Y.-L. Lai, L.-C. Lin and J.-F. Lee, J. Chem. Eng. Data, 2010, 55, 1186–1192 Search PubMed.
- C. Aulin, S. Ahola, P. Josefsson, T. Nishino, Y. Hirose, M. Österberg and L. Wågberg, Langmuir, 2009, 25, 7675–7685 CrossRef CAS.
- M. F. Daniel, B. Desbat, J. C. Lassegues, B. Gerand and M. Figlarz, J. Solid State Chem., 1987, 67, 235–247 CrossRef CAS.
- C. Guery, C. Choquet, F. Dujeancourt, J. M. Tarascon and J. C. Lassegues, J. Solid State Electrochem., 1997, 1, 199–207 CrossRef CAS.
-
G. Socrates, Infrared and Raman Characteristic Group Frequencies: Tables and ChartsJohn Wiley & Sons, New York, USA, 3rd edn, 2001 Search PubMed.
- J. Q. Yu and A. Kudo, Adv. Funct. Mater., 2006, 16, 2163–2169 CrossRef CAS.
- U. L. Štangar, N. Grošelj, B. Orel and P. Colomban, Chem. Mater., 2000, 12, 3745–3753 CrossRef CAS.
- K. V. Yumashev, A. M. Malyarevich, N. N. Posnov, I. A. Denisov, V. P. Mikhailov, M. V. Artemyev and D. V. Sviridov, Chem. Phys. Lett., 1998, 288, 567–575 Search PubMed.
- J. V. Gabrusenoks, P. D. Chikmach, A. R. Lusis, J. J. Kleperis and G. M. Ramans, Solid State Ionics, 1984, 14, 25–30 CrossRef.
- S.-H. Lee, H. M. Cheong, J.-G. Zhang, A. Mascarenhas, D. K. Benson and S. K. Deb, Appl. Phys. Lett., 1999, 74, 242–244 CrossRef CAS.
- J. G. Zhang, D. K. Benson, C. E. Tracy, S. K. Deb, A. W. Czanderna and C. Bechinger, J. Electrochem. Soc., 1997, 144, 2022–2026 CAS.
- K. Kuboyama, K. Hara and K. Matsushige, Jpn. J. Appl. Phys., 1994, 33, 4135–4136 Search PubMed.
- D. K. Hwang, H. J. Kim, H. S. Han and Y. G. Shul, J. Sol-Gel Sci. Technol., 2004, 32, 137–141 Search PubMed.
- T. Yamase, Chem. Rev., 1998, 98, 307–325 CrossRef CAS.
- L. H. Bi, E. B. Wang, L. Xu and R. D. Huang, Inorg. Chim. Acta, 2000, 305, 163–171 CrossRef CAS.
- K. Ajito, L. A. Nagahara, D. A. Tryk, K. Hashimoto and A. Fujishima, J. Phys. Chem., 1995, 99, 16383–16388 Search PubMed.
- B. Krebs, Acta Crystallogr., Sect. B: Struct. Crystallogr. Cryst. Chem., 1972, 28, 2222–2231 CrossRef CAS.
Footnotes |
† Electronic supplementary information (ESI) available: TEM image of the as-prepared WO3 colloid, XRD pattern of dried WO3 colloids, UV-Vis absorption spectrum of as-prepared WO3 colloid solution, and assignments for vibrational Raman bands for CE and TACE. See DOI: 10.1039/c2ra00217e |
‡ Present address: Nondestructive Evaluation Laboratory, Analytical Science Division, National Food Research Institute (NFRI), Tsukuba, Ibaraki, 305-8642, Japan. |
|
This journal is © The Royal Society of Chemistry 2012 |
Click here to see how this site uses Cookies. View our privacy policy here.