DOI:
10.1039/C2RA00038E
(Paper)
RSC Adv., 2012,
2, 5693-5700
Received
21st June 2011
, Accepted 24th April 2012
First published on 21st May 2012
Abstract
A comparative study was performed on the sorption capacity of the phenoxy acid herbicide (4-chloro-2-methylphenoxyacetic acid), also known as MCPA, on a single-walled carbon nanotube (SWCNT), three multi-walled carbon nanotubes with average outer diameters of 15, 30 and 50 nm (MWCNT15, MWCNT30 and MWCNT50), and nanoscale metal oxides (Al2O3, TiO2 and ZnO). The most efficient sorbent was the SWCNT, followed by the three MWCNTs. The metal oxide nanoparticles showed a much lower sorption capacity. The herbicide bound to the tested carbon nanotubes, by a combination of electron donor–acceptor (π–π EDA) interactions and hydrogen bonds. On the contrary, MCPA bound to the considered metal oxide nanoparticles by electrostatic interactions occurring between the carboxylate anions of the same herbicide and OH2+ of the sorbent. Experiments of cyclic sorption showed the pesticides to be totally removed by only two sorption cycles on the SWCNT and MWCNT15, whereas the same waste water was purified after four cycles on nano Al2O3. Finally, desorption studies carried out in ethanol showed a potential re-use of the SWCNT.
1. Introduction
The use of nanomaterials has enormously increased in recent years leading to the development of a new generation of technologies for environmental and public health protection. This nanotechnology has the potential to create novel and effective in situ treatment technologies for water pollution control, groundwater remediation, potable water treatment and air quality control.1 Nanoparticles, due to their high reactivity as a result of their small size and smallest pores, can accelerate the removal and degradation rate of contaminants in the source zone, via sorption or complexation, with a consequent decrease of the cost and time of remediation with respect to traditional treatment technologies. Nanomaterials can be grouped into four types:2 (1) carbon-based materials, such as fullerene, single walled carbon nanotubes (SWCNTs) and multi walled carbon nanotubes (MWCNTs); (2) metal-based materials such as quantum dots, nanogold, nanozinc, nanoaluminium, and nanoscale metal oxides like TiO2, ZnO and Al2O3; (3) dendrimers; and (4) composites.
Carbon nanotubes (CNTs) represent advanced materials that, due to their high surface area, good thermal stability and resistance, have been proposed as components for enzymatic biosensors,3 DNA probes4 and solid phase extraction.5,6 With particular regard to environmental technologies, their use has been investigated for the sorption of herbicides,5–8 phenols,9 heavy metals10 and nonpolar compounds.11–13 More specifically, a SWCNT is a tubular graphene sheet, with a diameter of about 1 nm, made up of benzene-type hexagonal rings, whereas MWCNTs are tubular graphene sheets, with diameters of 2–100 nm, rolled up into concentric cylinders with a layer spacing of 0.3–0.4 nm.
The nanoparticles of metal oxides represent a class of inorganic sorbing nanomaterials, widely used for numerous technological applications, i.e. pigments, semiconductors, food, cosmetics, biomedical areas and waste water treatment reactors.14–16 The great interest in these materials as sorbents for environmental remediation is linked not only to their high surface area but also to their fast contaminant sorption kinetics. Metal oxides, due to their oxidation states, coordination numbers, density and acid–base properties, have very different physical, chemical and structural properties and reactivity. For example aluminium oxide has high points of zero charge between 8–9.517 that make it positively charged over most typical pH ranges; consequently the removal efficiency of an anionic pollutant can be greatly increased.
The utilization of pesticides for agricultural practices, widely employed to increase yields and improve the quality and quantity of products, can be the source of soil and water contamination. The quality of soil and surface water deserves particular attention because of their importance in the ecosystem as well as in the supply of water for drinking and amenity use. Thus, their protection and, eventually, their remediation is a necessity of primary importance. The release of pesticides as a consequence of incorrect operations, accidental release and machinery washings, defined as point source contamination, can contribute to between 18 and 84% of their quantity measured in individual catchments.18,19
4-Chloro-2-methylphenoxyacetic acid (MCPA) is a phenoxy systemic herbicide used in post-emergence for the control of annual and perennial weeds in cereals, grassland and turf.20 It is very mobile and in aqueous solution occurs mainly in the anionic form, with a solubility at neutral pH of 270.9 mg L−121 and a half life of several weeks.22,23 This pesticide, which is classified by the U.S. Environmental Protection Agency (EPA) as a potential groundwater contaminant,24 is suspected to have mutagenic and carcinogenic properties. Because of its toxicity and environmental fate, the European Union has included it in the list of priority pollutants and has established a maximum allowed concentration of 0.1 μg L−1 for individual pesticide and 0.5 μg L−1 for total pesticides in drinking water.25
Therefore, the main objective of this research was to find the most efficient and convenient sorbents, among the four CNTs and the three metal oxide nanoparticles mentioned above, as potential filters for the decontamination of waste water polluted with MCPA in point sources. To this end, we report: (i) the sorption data of MCPA on the nanomaterials, elaborated according to the Freundlich equation, and the sorption mechanism; (ii) the results obtained by cyclic sorption experiments of MCPA on the nanomaterials, renewed at each cycle, for the removal of the herbicide from simulated waste water in point sources, and (iii) the results obtained by experiment for the specific desorption of MCPA from SWCNT for the potential reuse of this sorbent.
2. Materials and methods
2.1. Chemicals
Solvents were of HPLC grade (Carlo Erba, Milan, Italy) and were used without further purification. MCPA was purchased from the Sigma-Aldrich chemical company (Poole, Dorset, UK; both 99.0% purity). Labelled 14C-MCPA was purchased from the “Institute of Isotopes” (Budapest).
2.2. Sorbents
Four types of carbon based nanoparticles were used: one SWCNT and three MWCNTs (MWCNT15, MWCNT30 and MWCNT50). In addition three metal oxide nanoparticles (Al2O3, TiO2 and ZnO) were also used. The carbon based nanoparticles were from Chengdu Organic Chemistry Ltd., Chinese Academy of Sciences, whereas the metal oxide nanoparticles were from Zhejiang Hongsheng Material Technology Co., China. The CNTs used in this study are the same materials reported in a previous paper by Yang and Xing26 while the metal oxide nanoparticles were used previously by Wang et al.27 The point of zero charge (PZC) was determined according to the method described by Mustafa et al.28 Dry samples (100 mg) were dissolved in 40 mL of 0.001 M KCl solution as a background electrolyte and equilibrated for 30 min by means of a magnetic stirrer. Successively, the initial pH of the solution was adjusted to pH 4.0 by the addition of either 0.1 M HCl or 0.1 M KOH. The suspension was equilibrated for another 10 min, and the pH was then measured. The suspension pH was recorded every 2 min as a function of the volume of the titrant added until pH 10 was reached. The PZC of the two sorbents was determined from the variation of the surface charge density as a function of the pH. The characterization of all the sorbents is reported in Table 1.
Table 1 Properties of the CNTsa and nano-oxidesb
|
Outer diameter (nm)a |
Inner diameter (nm)a |
Length (μm)a |
Surface area (m2 g−1) |
PZC |
Particle size (nm)b |
Data reported by Yang and Xing.26
Data reported by Wang et al.27
|
SWCNT
|
1–2 |
0.8–1.6 |
10–50 |
541a |
5.7 |
— |
MWCNT15
|
8–15 |
3–5 |
10–50 |
174a |
6.2 |
— |
MWCNT30
|
20–30 |
5–10 |
10–50 |
107a |
6.3 |
— |
MWCNT50
|
30–50 |
5–15 |
10–50 |
94.7a |
6.2 |
— |
Al2O3
|
— |
— |
— |
187.8b |
8.9 |
60 ± 16 |
TiO2
|
— |
— |
— |
325.3b |
5.2 |
50 ± 10 |
ZnO
|
— |
— |
— |
53.4b |
8.4 |
19 ± 7 |
Diffuse reflectance infrared Fourier transform (DRIFT) analysis was performed using a Perkin-Elmer Spectrum One. Sample preparation was performed as follows: a quantity of MCPA corresponding to the maximum sorption value obtained on the isotherm of the herbicide was added to each sorbent. After incubation, the samples were centrifuged, and the precipitates washed twice with ultrapure water to remove the excess herbicide; then the samples obtained were lyophilized. Finally, 0.20 mg of each sample was mixed with 200 mg of KBr (FT-IR grade, Aldrich, Chemical, Co., Milwaukee, WI, USA). The mixture was finely ground in an agate mortar and transferred to a sample holder. Its surface was smoothed with a microscope glass slide, and DRIFT spectra were recorded. The interpretation of the signals was carried out according to that in ref. 29.
Sorption experiments were carried out by a batch equilibration technique at 25 ± 1 °C utilizing 1 mg of SWCNT, MWCNT15, MWCNT30 and MWCNT50 and 100 mg of Al2O3, TiO2 and ZnO in a final volume of 8 mL for SWCNT and 16 mL for the other sorbents. No electrolyte buffer and no pH variation were applied. Solid-to-solution ratios were adjusted to reach 30–80% solute uptake by the sorbents. Labelled and unlabelled 14C-MCPA was mixed to give an initial concentration ranging from 2.5 to 100 mg L−1 in water. These solutions were added to 8 mL and 16 mL screw cap vials, until a minimum headspace was achieved. Vials were immediately sealed with aluminium foil-lined Teflon® screw caps and shaken for three days. After this time the vials were centrifuged at 12
000 rpm for 30 min and 1 mL of the supernatant was removed and added to a 7 mL Scintiverse cocktail (Fisher Scientific Co., Pittsburgh) for liquid scintillation counting (LSC) (Beckman LS 6500, CA). Experimental uncertainties evaluated in vials without nanomaterials indicated that the total uncertainty was less than 4% of the initial concentrations of pesticide.
The amount of MCPA sorbed by sorbents (S) in the sorption system was calculated using the mass balance equation:
where
V is the solution volume (L),
m is the amount of sorbent (kg), and
Ci and
Ce (mg L
−1) are the initial and equilibrium MCPA concentrations, respectively.
The sorption data were analyzed according to the linearized form of the Freundlich equation that can be written as follows:
where
S is the concentration of
pesticide sorbed (mg kg
−1);
Ce is the equilibrium concentration of
pesticide (mg L
−1);
KF [(mg kg
−1)/(mg L
−1)
N] and
N (dimensionless) are the Freundlich sorption parameters.
The single point sorption coefficient, Kd, was calculated based on the Freundlich equation at a given equilibrium concentration (Ce) corresponding to 5 mg L−1:
Cyclic sorption experiments were carried out on the SWCNT, MWCNT15 and Al2O3. In the first cycle, a predetermined quantity of MCPA stock solution corresponding to 10 mg L−1 was pipetted into the flasks, in the same experimental conditions reported for the sorption experiments. After the contact time (three days), the sorbents were separated by centrifugation and the amount of MCPA in the supernatants was analyzed by high performance liquid chromatography (HPLC). For the second cycle, the supernatants were treated with fresh sorbent maintaining the constant solid–liquid ratio. The experiments were repeated until the equilibrium concentrations were constant.
The amount of MCPA in the supernatants was measured by an Agilent 1200 Series HPLC equipped with a DAD array and ChemStation Agilent Software. A Macharey-Nägel Nucleosil 100-5 C18 column (stainless steel 250 × 4 mm) was used. The mobile phase, comprising a binary system of 50
:
50 acetonitrile
:
phosphate buffer (0.1%, pH 3.0) was pumped at a 1 mL min−1 flow in an isocratic mode. The detector was set at 225 nm. The injection volume was 20 μL. Quantitative determinations of MCPA were performed by a calibration curve.
2.5. Desorption experiments
Desorption studies were conducted using the dilution method. An amount of MCPA corresponding to 5 mg L−1 was sorbed on the SWCNT in the same experimental conditions described for the sorption isotherms except that no labelled MCPA was used. After the equilibrium time (three days) half the volume of the supernatant was removed and substituted immediately with the same volume of ethanol or water. The vials were resealed, shaken for an additional three days and centrifuged as described above. The supernatant was removed and analyzed by HPLC while the sorbent was washed twice with 2 mL of ultrapure water and reused for a second sorption–desorption cycle. The percentage of MCPA desorbed was calculated by the difference between the amounts sorbed and those desorbed. The study of recyclability consisted of a repetition of three successive sorption and desorption cycles.
3. Results and discussion
All of the isotherms for the sorption of MCPA on the selected carbon based nanomaterials fitted with the linearized form of the Freundlich isotherm (R2 = 0.99) and showed 1/N values that varied from 0.46 to 0.62 (Table 2 and Fig. 1). The intensity parameter, 1/N, indicates the deviation of the adsorption isotherm from linearity. 1/N = 1 indicates the sorption is linear with homogeneous sorption sites and there is no interaction between the adsorbed MCPA. 1/N < 1 shows that the adsorption is favourable, new sorption sites are available and the sorption capacity increases. 1/N > 1 indicates that the adsorption bonds are weak, adsorption capacities decrease and are unfavourable. The Kd values reported in Table 2 clearly highlight the higher sorption capacity of the SWCNT compared to MWCNTs. In fact, the SWCNT removed MCPA four fold more than MWCNT15 and six fold more than MWCNT30 and MWCNT50.
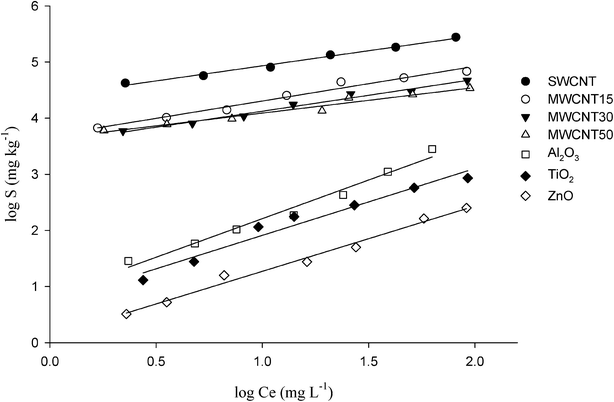 |
| Fig. 1 Sorption experiments of MCPA ranging from 2.5 to 100 mg L−1 conducted using 1 mg of SWCNT, MWCNT15, MWCNT30 and MWCNT50, and 100 mg of Al2O3, TiO2 and ZnO in a final volume of 8 mL for the SWCNT and 16 mL for the other sorbents. | |
Table 2 Freundlich parameters and Kd for the sorption of MCPA on CNTs and nano-oxides
|
alogKF |
a1/N |
R2 |
K
d
|
The values were given as a mean of the duplicate samples ± standard error.
|
SWCNT
|
4.41 ± 0.025 |
0.52 ± 0.02 |
0.99 |
11 871 |
MWCNT15
|
3.69 ± 0.053 |
0.62 ± 0.04 |
0.99 |
2657 |
MWCNT30
|
3.56 ± 0.044 |
0.57 ± 0.03 |
0.99 |
1817 |
MWCNT50
|
3.64 ± 0.050 |
0.46 ± 0.03 |
0.99 |
1830 |
Al2O3
|
0.83 ± 0.11 |
1.38 ± 0.09 |
0.99 |
12 |
TiO2
|
0.72 ± 0.13 |
1.19 ± 0.10 |
0.99 |
7 |
ZnO
|
0.11 ± 0.07 |
1.16 ± 0.05 |
0.98 |
2 |
Considering that CNTs are condensed aromatic rings with a PZC between 5.7–6.3 (Table 1) and MCPA is an aromatic weak acid with a pKa of 3.07, the nature of the binding depends on the solution pH and the unique structure and electronic properties of the sorbents. More specifically, in our studies the pH of the solutions decreased from about 5.0 up to 3.2 by increasing the MCPA concentration from 2.5 up to 100 mg L−1. At pH 3.2 the herbicide was present in both the neutral and negative form, therefore, both hydrophobic and electrostatic sorption mechanisms could be anticipated, although it was not possible to distinguish between them in this work.
CNTs sorbed MCPA through a combination of van der Waals and π–π electron donor–acceptor interactions (π–π EDA) that occurred between the condensed aromatic rings of the sorbents and the aromatic ring of the pesticide. The π–π EDA binding has been widely used to address the sorption mechanism of organic compounds on graphene surfaces.30 Organic compounds with a functionalized benzene ring could be sorbed on CNTs, because the polarisable graphene structure might act as an amphoteric sorbate attracting π-acceptors or π-donors. It is well-known that the electron density of an aromatic ring is strongly influenced by the nature of the bound groups. MCPA, because of its chemical structure, has one polar carboxylic group and one chloride group. The chloride group on the aromatic ring of MCPA acts as an electron-withdrawing group, therefore reducing the overall electron density in the π ring system. Hence, MCPA can act as an acceptor in such complexes. Also, the oxygen-group dipole moment is the determining factor in the strength of the donor–acceptor complex formed. Carbonyl oxygen has a larger dipole moment than carboxylic acid oxygen, and hence, it acts as a strong donor.31 Consequently, it is possible that MCPA molecules adsorb by a donor–acceptor complex mechanism involving carbonyl oxygen, with the surface of the nanomaterials acting as the electron donor and the aromatic ring of MCPA acting as the acceptor. Chen et al., 2008,32 indicated that the strong adsorptive interaction between hydroxyl-substituted aromatics and CNTs was mainly due to the electron-donating effect of the hydroxyl group, which caused a strong EDA interaction between the sorbate and the π-electron-depleted regions on the graphene surfaces of the CNTs. The presence of the carboxylic group also allowed the pesticide to be sorbed through H–bonds occurring between the MCPA sorbed on the surface of the CNTs and that dissolved in water.
To clarify the sorption mechanism CNTs–MCPA complexes were studied by DRIFT spectroscopy. Because the DRIFT spectra of CNTs–MCPA complexes were similar, in Fig. 2 is reported the only spectrum of the SWCNT–MCPA complex. The DRIFT spectrum of MCPA found bands corresponding to the C
C vibrations of the aromatic ring (1493 cm−1), bands assigned to the antisymmetric and symmetric vibration of C–O–C (1243 cm−1 and 1079 cm−1, respectively), and bands corresponding to the carboxylic group (monomeric and dimeric C
O, respectively, at 1742 cm−1 and 1706 cm−1, C–O stretching at 1297 cm−1 and O–H bending at 1428 cm−1) and the carboxylate anion (antisymmetric and symmetric stretching at 1598 and 1401 cm−1, respectively). The DRIFT spectrum of the SWCNT showed a band at 3436 cm−1 attributable to water molecules while the peaks at 1633 and 1382 cm−1 are assigned to the sp2-hybridized carbon in the graphitic structure of the CNTs. The DRIFT spectrum of the MCPA–SWCNT complex system, compared to that of MCPA, showed the appearance of a new band at 1454 cm−1 due to the stretching of the MCPA benzene ring, which was shifted with respect to the band at 1493 cm−1 observed in the spectrum of the pesticide. The shift of this signal can be attributed to the formation of van der Waals and π–π EDA interactions that occurred between the condensed aromatic rings of the CNTs and the aromatic ring of MCPA. In addition two new bands at 1731 and 1684 cm−1 evidenced a shift and reduction with respect to those observed at 1742 and 1706 cm−1 in the spectrum of MCPA and were attributed to the signals of the monomeric and dimeric carboxylic acid. These absorptions are in accordance with the formation of one or two layers of MCPA sorbed on the surfaces of the CNTs. As confirmation of this sorption mechanism, Lin and Xing33 demonstrated that at high equilibrium concentrations pyrogallol, a phenol with 3 –OH groups, showed a Kd more elevated than catechol and phenol (2 and 1 –OH groups, respectively) indicating that the increase of –OH allowed pyrogallol to be sorbed through H–bonds between the surface adsorbed with the pyrogallol and that dissolved in water. Pyrzynska et al.8 reported that the sorption capacity of the herbicide dicamba, a phenoxyalkanoic acid, by CNTs increased remarkably at lower pH of the sample solution (pH 3) and occurred by electrostatic and hydrophobic interactions. Moreover, the same authors observed that at low pH the ionization of the herbicide and the surface charge of the CNTs decreased. Because in this study the sorption of MCPA occurred without pH adjustment with respect to the activated carbons a more accurate investigation at low pH is necessary to define the maximum amount sorbed by CNTs.
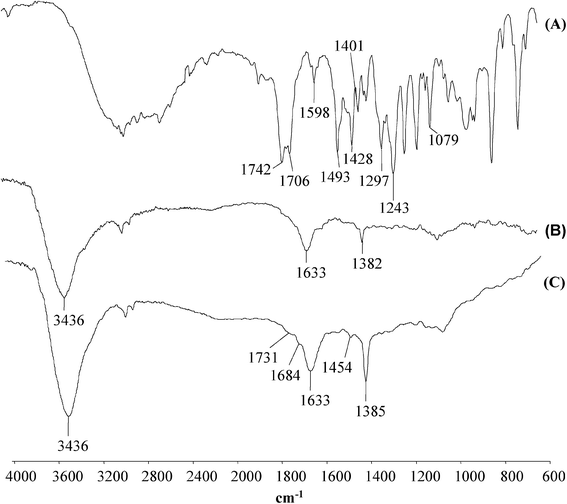 |
| Fig. 2 DRIFT spectra of MCPA (A), SWCNT (B) and the SWCNT–MCPA complex (C). | |
The properties of the metal oxide nanoparticles are shown in Table 1. It is noted that the particle size and surface area of Al2O3 and TiO2 are significantly greater than that of ZnO, whereas the PZC of TiO2 showed a different range with respect to the other two nano-oxides.
The sorption data of MCPA on the metal oxide nanoparticles, fitted with the linearized form of the Freundlich isotherm (R2 > 0.98), showed 1/N values of 1.38, 1.19 and 1.16 for Al2O3, TiO2 and ZnO, respectively (Fig. 1 and Table 2). The Kd values showed that the sorption capacity of MCPA followed the order Al2O3 > TiO2 > ZnO.
Generally, the sorption of chemicals on a particle’s surface is a function of pH and the ionic strength of the solution and of the characteristics of the organic molecule, such as the molecular size and charge.34 The strongest interactions between metal oxides and an organic anion may occur when the charge on the organic molecule is opposite to that on the surface sites. Therefore, the different amount of MCPA sorbed on the three matrices could be explained considering the pKa value (3.07) of MCPA and the PZC of the three metal oxide nanoparticles (Table 1). In particular, the values of PZC of Al2O3 and ZnO showed a more basic character with respect to that of TiO2. So the different amounts of MCPA sorbed on the three metal oxide nanoparticles can be explained by the different PZC values, which leads to more or less protonation of the surface at working pH conditions. As an example, the final pH of a solution containing 100 mg L−1 of MCPA was 5.5, 5.9 and 3.2 for Al2O3, ZnO and TiO2, respectively. In these experimental conditions the metal oxide nanoparticles always had a positive surface of OH2+ but the density of the positive charge was different and followed the order Al2O3 > ZnO > TiO2. When the pH < PZC, positive charges start to prevail over negative ones, making the surface positive. The density of the positive charge increases with the decreasing of the pH. Organic acids can form stronger interactions with metal oxides when pKa of the acid is lower than the PZC of the metal oxide. In the presence of Al2O3 and ZnO the carboxylic group of MCPA was completely in the ionized form (–COO−) and the pesticide was sorbed by electrostatic interactions occurring on the positive surfaces of both the sorbents. In particular, Al2O3, for higher surface area, sorbed more MCPA than ZnO (188 m2 g−1 against 54 m2 g−1; Table 1). For TiO2 the amount of MCPA sorbed was the result of a balance between the PZC and the surface area. In fact, at pH 3.2 the higher surface area corresponding to 325 m2 g−1 (Table 1) balanced the low density of the positive charge and the equilibrium between the unionized and ionized carboxylic groups of MCPA (estimated to be about 50
:
50).
Cyclic sorption for the removal of MCPA from simulated waste water
The experiments of cyclic sorption investigated the potential to remove MCPA from simulated waste water. The results showed that a total removal of MCPA on the SWCNT and MWCNT15 required only two sorption cycles whereas the total removal of the herbicide by Al2O3 was performed by four cycles (Fig. 3). Therefore, the SWCNT and MWCNT15 were found to be the most efficient sorbents for the removal of MCPA from contaminated water.
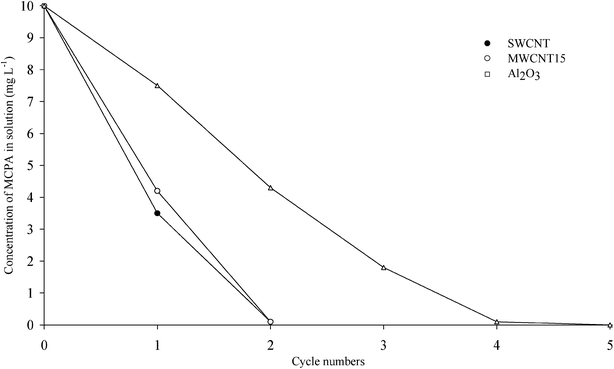 |
| Fig. 3 Cyclic sorption of MCPA (10 mg L−1) on the SWCNT, MWCNT15 and Al2O3. | |
Cyclic desorption for the recovery of the SWCNT
For the successful application of a sorption system, the efficient regeneration of the used sorbent is very important from an economic point of view. In general, there are many regeneration techniques such as thermal, steam, acid or base and solvent regenerations. The choice of a certain regeneration method should depend upon the physical and chemical characteristics of both the sorbate and the sorbent. The SWCNT was shown to sorb MCPA with a higher capacity than the other CNTs. Therefore, we conducted desorption experiments with water and ethanol to obtain additional information on a possible recovery of the SWCNT for many sorption cycles. In particular, the pesticide was desorbed by 92% with ethanol, but to a lesser extent (45%) with water, indicating that the hydrophobic interactions between MCPA and the organic solvent were more important than the electrostatic bonds between water and the pesticide. This experiment confirmed the nature of the weak links between MCPA and the SWCNT consisting prevalently of π–π EDA interactions. The remaining 8% not desorbed with ethanol was probably due an irreversible link occurring between the MCPA and impurities, such as metal catalyst and the amorphous carbons of the SWCNT.26 The regenerated SWCNT after desorption of the pesticide with ethanol was used again for another two sorption–desorption cycles. It is observed that the sorption percentage of MCPA decreased slightly (about 5%) by increasing the number of cycles while a total desorption was obtained after every cycle. Many authors have reported that CNTs can be reused for many sorption–desorption cycles. In particular, Madrakian et al., 2011,35 and Lu et al., 2011,36 reported that the reusability of MWCNTs was greater than 5 cycles using as the desorption agent acetonitrile and hydrochloric acid, respectively. In another study, López-Feria et al., 2009,37 reported that cartridge of CNTs eluted with ethyl acetate can be reused 100 times for the determination of pesticides in virgin olive oil. Su et al., 2011,38 demonstrated that adsorption capacities and the physicochemical properties of MWCNTs functionalized with 3-aminopropyltriethoxysilane are preserved for 100 adsorption–desorption cycles. Zhou et al.6 reported that MWCNTs can be reused more than 200 times with excellent spiked recoveries in the range of 88–110%.
CNTs are sorbents that can be 50 times more expensive than activated carbon39 but adsorption on activated carbon can be disadvantageous for the high energy demands and consequently high costs of the regeneration of the adsorbent.40 Ravelo-Pérez et al.,41 reported that highly cheap CNTs are also available and may represent a convenient alternative. Some studies reported that CNTs are more efficient than carbon black and can be recycled for many sorption cycles. Zhou et al.42 in a comparative study reported that MWCNTs are more efficient than activated carbon and C18 for solid phase extraction (SPE) from environmental waters of atrazine and simazine. Ravelo-Pérez et al.41 in another comparative study in terms of the analytical performance, application to environmental water, cartridge reuse, adsorption capacity and the cost of CNTs and activated carbon reported that the adsorption capacity of CNTs was almost three times higher than that of activated carbon. In Table 3 are reported the values of the sorption capacity of MCPA on the SWCNT compared with some activated carbons. It can be observed that the sorption capacity of the SWCNT (25.7 g kg−1) conducted in native experimental conditions was of a similar magnitude of order compared to the activated carbons.
Table 3 Values of the sorption capacity of MCPA on the SWCNT compared with those of the activated carbons (n.r, not reported)
Materials |
pH |
Sorption capacity (g kg−1) |
By Nyazi et al.43
By Gimeno et al.44
|
SWCNT
|
native |
25.7 |
CA N115
a
|
n.r |
37.1 |
CO N375
a
|
n.r |
48.2 |
CA N375
a
|
n.r |
44.9 |
CO N539
a
|
n.r |
23.0 |
CA N660
a
|
n.r |
18.9 |
SRB N762
a
|
n.r |
5.8 |
Norit 0.8
b
|
7 |
63.2 |
Aquacarb 207C
b
|
7 |
35.2 |
Aquacarb 208A
b
|
7 |
29.5 |
Aquacarb 208EA
b
|
7 |
11.5 |
4. Conclusions
This study demonstrates that CNTs and in particular SWCNTs have higher sorption capacities for MCPA than metal oxide nanoparticles. More specifically, a SWCNT proves to be the most effective for the possible use as a filter for the control of waste water contamination with MCPA in point sources. In fact, this matrix allows the total removal of the herbicide by only two sorption cycles, with a usual procedure of centrifugation in native conditions for the separation of purified water. In addition, in potential large scale applications, its solid form could be easily used, for example in a biobed system, which needs solid materials. Finally, the high efficiency of the desorption process of MCPA from the SWCNT demonstrates a potential reuse of this sorbent for many sorption cycles, balancing its high cost. Competitive sorbent materials represented by activated carbons require controlled experimental conditions while the sorption of MCPA on SWCNTs occurs more advantageously in native conditions.
References
-
K. J. Klabunde, Nanoscale materials in chemistry, Wiley-Interscience, New Jersey, 2001 Search PubMed.
- U.S. Environmental Protection Agency, Nanotechnology White Paper-External Review Draft, 2005; Available from: http://www.epa.gov/osa/pdfs/EPA_nanotechnology_white_paper_external_review_draft_12-02-2005.pdf.
- J. Wang, Nanomaterial-based electrochemical biosensors, Analyst, 2005, 130, 421–26 RSC.
- G. Cheng, J. Zhao, Y. Tu, P. He and Y. A Fang, Sensitive DNA electrochemical biosensor based on magnetite with a glassy carbon electrode modified by multi-walled carbon nanotubes in polypyrrole, Anal. Chim. Acta, 2005, 533, 11–16 CrossRef CAS.
- Q. Zhou, J. Xiao and W. Wang, Comparison of multiwalled carbon nanotubes and a conventional absorbent on the enrichment of sulfonylurea herbicides in water samples, Anal. Sci., 2007, 23, 189–92 CrossRef.
- Q. Zhou, Y. Ding and J. Xiao, Simultaneous determination of cyanazine, chlorotoluron and chlorbenzuron in environmental water samples with SPE multiwalled carbon nanotubes and LC, Chromatographia, 2007, 65, 25–30 CAS.
- M. Biesaga and K. Pyrzynska, The evaluation of carbon nanotubes as a sorbent for dicamba herbicide, J. Sep. Sci., 2006, 29, 2241–44 CrossRef CAS.
- K. Pyrzynska, A. Stafiej and M. Biesaga, Sorption behavior of acidic herbicides on carbon nanotubes, Microchim. Acta, 2007, 159, 293–8 CrossRef CAS.
- Y. Cai, G. Jiang, J. Liu and Q. Zhou, Multiwalled carbon nanotubes as a solid-phase extraction adsorbent for the determination of bisphenol, 4-n-nonylphenol, and 4-tert-octylphenol, Anal. Chem., 2003, 75, 2517–21 CrossRef CAS.
- P. Liang, Y. Liu, L. Guo, J. Zeng and H. Lu, Multiwalled carbon nanotubes as solid-phase extraction adsorbent for the preconcentration of trace metal ions and their determination by inductively coupled plasma atomic emission spectrometry, J. Anal. At. Spectrom., 2004, 19, 1489–92 RSC.
- D. Tasis, N. Tagmatarchis, A. Bianco and M. Prato, Chemistry of carbon nanotubes, Chem. Rev., 2006, 106, 1105–36 CrossRef CAS.
- K. Yang, L. Zhu and B. Xing, Adsorption of polycyclic aromatic hydrocarbons by carbon nanomaterials, Environ. Sci. Technol., 2006, 40, 1855–61 CrossRef CAS.
- K. Yang, X. Wang, L. Zhu and B. Xing, Competitive sorption of pyrene, phenanthrene, and naphthalene on multiwalled carbon nanotubes, Environ. Sci. Technol., 2006, 40, 5804–10 CrossRef CAS.
- Y. Pang and X. Bao, Aluminum oxide nanoparticles prepared by water-in-oil microemulsions, J. Mater. Chem., 2002, 12, 3699–704 RSC.
- L. K. Adams, D. Y. Lyon and P. J. J. Alvarez, Comparative ecotoxicity of nanoscaled TiO2, SiO2, and ZnO water suspensions, Water Res., 2006, 40, 3527–32 CrossRef CAS.
- D. B. Warheit, T. R. Webb, K. L. Reed, S. Frerichs and C. M. Sayes, Pulmonary toxicity study in rats with three forms of ultrafine-TiO2 particles: Differential responses related to surface properties, Toxicology, 2007, 230, 90–104 CrossRef CAS.
-
D. L. Sparks, Environmental Soil Chemistry, Academic Press, Amsterdam, 2nd edn, 2003 Search PubMed.
- D. C. Yoder, B. K. Corwin, T. C. Mueller, W. E. Hart, J. B. Wills and C. R. Mote, Development of a system to manage pesticide contaminated wastewater, Trans. ASAE, 2001, 44, 877–90 CAS.
- K. Muller, M. Bach, H. Hartmann, M. Spiteller and H. G. Frede, Point- and non point-source pesticide contamination in the Zwester Ohm catchement, Germany, J. Environ. Qual., 2002, 31, 309–18 CrossRef CAS.
-
C. Tomlin, The Pesticide Manual, British Crop Protection Council, Surrey GU9 7PH, UK, 10th edn, 1994 Search PubMed.
-
M. Muccinelli, Prontuario degli agrofarmaci, Edagricole, 11th edn, 2006 Search PubMed.
- M. J. Cerejeira, P. Viana, S. Batista, T. Pereira, E. Silva and M. J. Valério, Pesticides in Portuguese surface and ground waters, Water Res., 2003, 37, 1055–63 CrossRef CAS.
- A. Laganá, A. Bacaloni, I. De Leva, A. Faberi, G. Fago and A. Marino, Occurrence and determination of herbicides and their major transformation products in environmental waters, Anal. Chim. Acta, 2002, 462, 187–98 CrossRef.
-
M. Walker, H. Lawrence, EPA's Pesticide Fact Sheet Database, Lewis Publishers Inc., Chelsea MI, 1992 Search PubMed.
- EU Council, Directive on the Quality of Water Intended for Human Consumption, 98/83/CE, 1998.
- K. Yang and B. Xing, Desorption of polycyclic aromatic hydrocarbons from carbon nanomaterials in water, Environ. Pollut., 2007, 145, 529–37 CrossRef CAS.
- X. Wang, J. Lu, M. Xu and B. Xing, Sorption of pyrene by regular and nanoscaled metal oxide particles: influence of adsorbed organic matter, Environ. Sci. Technol., 2008, 42, 7267–72 CrossRef CAS.
- S. Mustafa, S. Tasleem and A. Naeem, Surface charge properties of Fe2O3 in aqueous and alcoholic mixed solvents, J. Colloid Interface Sci., 2004, 275, 523–29 CrossRef CAS.
-
R. M. Silverstein, G C. Bassler, T. C. Morrill, Spectrometric identification of organic compounds, Wiley, London-New York, 5th edn, 1991 Search PubMed.
- D. Q. Zhu and J. J. Pignatello, Characterization of aromatic compound sorptive interactions with black carbon (charcoal) assisted by graphite as a model, Environ. Sci. Technol., 2005, 39, 2033–41 CrossRef CAS.
- M. Streat and D. J. Horner, Adsorption of highly soluble herbicides from water using activated carbon and hypercrosslinked polymers, Trans IChemE, 2000, 78, 363–82 CrossRef CAS.
- W. Chen, L. Duan, L. Wang and D. Zhu, Adsorption of Hydroxyl- and Amino-Substituted Aromatics to Carbon Nanotubes, Environ. Sci. Technol., 2008, 42, 6862–68 CrossRef CAS.
- D. Lin and B. Xing, Adsorption of phenolic compounds by carbon nanotubes: role of aromaticity and substitution of hydroxyl groups, Environ. Sci. Technol., 2008, 42, 7254–59 CrossRef CAS.
- S. E. Mylon, K. L. Chen and M. Elimelech, Influence of natural organic matter and ionic composition on the kinetics and structure of hematite colloid aggregation: implication to iron depletion in estuaries, Langmuir, 2004, 20, 9000–6 CrossRef CAS.
- T. Madrakian, A. Afkhami, M. Ahmadi and H. Bagheri, Removal of some cationic dyes from aqueous solutions using magnetic-modified multi-walled carbon nanotubes, J. Hazard. Mater., 2011, 196, 109–114 CrossRef CAS.
- S. Lu, J. Xu, C. Zhang and Z. Niu, Adsorption and desorption of radionuclide europium(III) on multiwalled carbon nanotubes studied by batch techniques, J. Radioanal. Nucl. Chem., 2011, 287, 893–898 CrossRef CAS.
- S. López-Feria, S. Cárdenas and M. Valcárcel, One step carbon nanotubes-based solid-phase extraction for the gas chromatographic-mass spectrometric multiclass pesticide control in virgin olive oils, J. Chromatogr., A, 2009, 1216, 7346–7350 CrossRef.
- F. Su, C. Lu and H. S. Chen, Adsorption, desorption, and thermodynamic studies of CO2 with high-amine-loaded multiwalled carbon nanotubes, Langmuir, 2011, 27, 8090–8098 CrossRef CAS.
- A. H. El-Sheikh, J. A. Sweileh, Y. S. Al-Degs, A. A. Insisi and N. Al-Rabady, Critical evaluation and comparison of enrichment efficiency of multi-walled carbon nanotubes, C18 silica and activated carbon towards some pesticides from environmental waters, Talanta, 2008, 74, 1675–80 CrossRef CAS.
- I. Vergili and H. Barlas, Removal of 2,4-D, MCPA and metalaxyl from water using Lewatit VP OC 1163 as sorbent, Desalination, 2009, 249, 1107–14 CrossRef CAS.
- L. M. Ravelo-Pérez, A. V. Herrera-Herrera, J. Hernández-Borges and M. A. Rodríguez-Delgado, Carbon nanotubes: Solid-phase extraction, J. Chromatogr., A, 2010, 1217, 2618–41 CrossRef.
- Q. Zhou, J. Xiao and W. Wang, Trace analysis of triasulfuron and bensulfuronmethyl in water samples using a carbon nanotubes packed cartridge in combination with high-performance liquid chromatography, Microchim. Acta, 2007, 157, 93–98 CrossRef CAS.
- K. Nyazi, A. Bacaoui, A. Yaacoubi, H. Darmstadt, A. Adnot and C. Roy, Influence of carbon black surface chemistry on the adsorption of model herbicides from aqueous solution, Letters to the Editor/Carbon, 2005, 43, 2215–34 Search PubMed.
- O. Gimeno, P. Plucinski, S. T. Kolaczkowski, F. J. Rivas and P. M. Alvarez, Removal of the herbicide MCPA by commercial activated carbons: equilibrium, kinetics, and reversibility, Ind. Eng. Chem. Res., 2003, 42, 1076–1086 CrossRef CAS.
|
This journal is © The Royal Society of Chemistry 2012 |
Click here to see how this site uses Cookies. View our privacy policy here.