UVA and endogenous photosensitizers – the detection of singlet oxygen by its luminescence†
Received
12th May 2011
, Accepted 26th August 2011
First published on 11th October 2011
Abstract
UVA irradiation (320–400 nm) comprises about 95 percent of incident midday solar ultraviolet irradiation. It penetrates skin much deeper than UVB irradiation. The absorption of UVA irradiation in endogenous chromophores frequently leads to the generation of reactive oxygen species such as singlet oxygen (1O2). 1O2 is an important biochemical intermediate in multiple biological processes. Beside other procedures, the direct detection of 1O2 by its luminescence is a powerful tool that helps to understand the generation of 1O2 during UVA exposure in solution, in vitro and in vivo. This article describes the endogenous photosensitizers, their ability to generate 1O2 under UVA irradiation, and the detection technology to visualize the action of 1O2.
1. Introduction
Solar UV radiation causes many adverse effects in tissue such as skin, which can be attributed to DNA damage. Consequently, skin cancer has accounted for about 40 percent of all cancers in the United States and their frequency has been increasing.1UVB (280–320 nm) is directly absorbed by cellular DNA, resulting in dipyrimidine lesions that include cyclobutane pyrimidine dimers (CPD), especially thymine dimers or pyrimidine photoproducts.2
The major component of solar radiation (∼95%) is UVA (320–400 nm), which has been shown to produce likewise deleterious biological effects.3 Exposure to UVA irradiation has been recognized as a source of aging of eye lens proteins and as a risk factor for cataract formation.4 Revisiting the photochemistry of solar UVA in human skin, it was stated that the importance of UVA in skin cancer is undeniable.5,6 Cyclobutane pyrimidine dimers were detected in significant yield in whole human skin exposed to UVA radiation.7UVA irradiation is also responsible for the most frequent photo-dermatosis of the skin.8 Recent epidemiological data showed that UVA radiation is even involved in the genesis of cutaneous melanoma.9
UVA radiation can penetrate deeper into tissue than UVB, which may lead to interaction with more tissue constituents as compared to UVB. However, UVA radiation is not sufficiently absorbed in proteins or DNA, which leads to different mechanisms of action in tissue for UVA and UVB. It is known that also UVA radiation leads to oxidative damage, single- and doublestrand breaks, produces secondary photoreactions, damages DNA by indirect photosensitizing reactions, and induces the photoproduct 8-oxo-7, 8-dihydro-2′-deoxyguanosine (8oxoG) in human skin.10 Thus, UVA radiation acts indirectly by producing reactive oxygen species (ROS), in which the highly reactive singlet oxygen (1O2) plays a major role.3
2. Photodynamic generation of singlet oxygen
The first prerequisite of photodynamic generation of 1O2 is the absorption of radiation in molecules (Fig. 1). These could be either endogenous photosensitizers or exogenous molecules, which are accidentally present in UVA exposed skin.11,12 Many molecules convert the absorbed energy simply to heat. However, after light absorption, some molecules can effectively cross over to a long-lived triplet T1 state. This is known as intersystem crossing (ISC). In contrast to the short-lived singlet S1 state, which shows a lifetime in the order of nanoseconds, the triplet T1 state is long-lived with lifetimes in the order of microseconds to milliseconds. This allows an efficient transfer of energy or charge to substrate or molecular oxygen generating radicals or 1O2, respectively (Fig. 2).
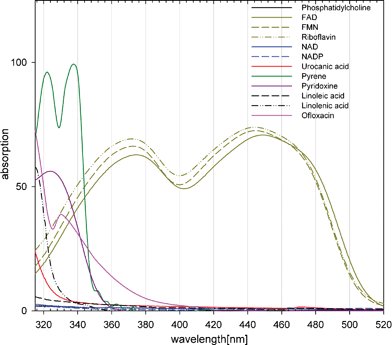 |
| Fig. 1 The absorption of some molecules that may act as endogenous photosensitizers to generate singlet oxygen. For a better illustration, absorption is given in percent (absorption = 100% − transmission). The values are measured with spectrophotometer (Beckman, DU 640) in polar or nonpolar solvents at a concentration of 50 μmol L−1, except for phosphatidylcholine (2.2 mmol L−1), fatty acids (10 mM), ofloxacin (10 μg ml−1) and pyridoxine (200 μM). The fatty acids (purity: 99%) contain traces of oxidized products that enables absorption of UVA radiation. Two substances were exemplarily added to the figure that may be present in skin accidentally and can act as UVA photosensitizer: pyrene (in black tattoo inks), ofloxacin (systemic treatment with antibiotics). | |
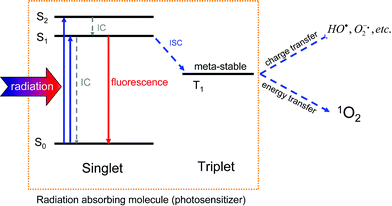 |
| Fig. 2 The scheme shows the generation of reactive oxygen species that is initiated by radiation absorption in a photosensitizer molecule. The absorption leads to population of excited singlet states in the molecule, which rapidly (picoseconds) yields S1 population via internal conversion (IC). Within nanoseconds, the absorbed energy is either converted to heat (IC) or to fluorescence. A third pathway is the intersystem crossing (ISC) in the molecule to the metastable triplet T1 state (lifetime: μs to ms), which allows energy or charge transfer to other molecules such as oxygen. | |
These specific generation mechanisms of ROS comprise the same mechanisms as known in photodynamic therapy of tumors (PDT)13 or in antimicrobial PDT (aPDT).14 Those molecules in tissue that convert the energy to reactive oxygen species (ROS) are called endogenous photosensitizers. Some of the endogenous photosensitizers in cells or tissue are identified such as flavins,15NADH/NADPH,16urocanic acid,15,17sterols,18 and anthraquinones.19
3.
Detection of singlet oxygen
The detection of 1O2, in particular when excited with UVA, is performed by using various methods that can be assigned to indirect and direct procedures. The main methods applied are the use of quenchers, measurement of 1O2 luminescence, and electronic paramagnetic spin resonance (Table 1).
Table 1 Singlet oxygen generation and detection under UVA exposure
Indirect detection
An important indirect proof of 1O2 is the chemical analysis of its reaction products,20–22 although this method frequently yields no unequivocal results. Specific quenchers such as sodium azide shorten the lifetime of 1O2, whereas the use of the solvent deuterium oxide (D2O) extends the lifetime of 1O2. By shortening or extending the 1O2 lifetime, the related damage of cellular structures is reduced or pronounced, which can be detected with different cellular parameters (e.g. mitochondrial activity or cell survival).23 When adding spin traps, ESR signals show the presence of 1O2.24 In addition, 1O2 can be detected by exploiting its chemical reactivity in different settings such as RNO-bleaching25,26 or chemoluminescence assays.27 The chemoluminescence assays are frequently based on switch-on fluorescence of rhodamines or fluoresceins such as ‘1O2 Sensor Green (SOSG)’.
Except for chemical analysis, the disadvantages of these indirect methods are obvious since they require the application of reporter molecules, which may show limited access to living cellsin vitro. It is hence not warranted that the quencher reach the site of 1O2 concentration with an appropriate concentration. The application in vivo is also limited because some of these substances are toxic or do not penetrate tissue to a sufficient extent.
Direct detection
The non-radiative deactivation of 1O2 is accompanied by radiative deactivation yielding infrared luminescence at about 1270 nm. The luminescence detection is a great tool to directly detect 1O2 without adding any reporter molecules, in particular for experiments with UVA radiation (Fig. 3). This technology can be applied for experiments in solution, in vitro and in vivo.
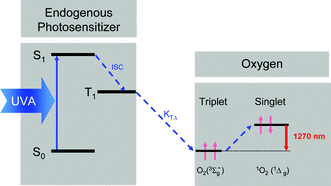 |
| Fig. 3 The rate constant KTΔ describes the formation of 1O2, which corresponds to the transition from the molecular oxygen ground state (triplet state) to its first singlet state. 1O2 can be detected by its weak luminescence that occurs at 1270 nm. | |
The major disadvantage of luminescence detection is the very low quantum yield of the radiative decay in the order of 10−7, which requires a very sensitive detection system. For more than 10 years, however, special photomultipliers have been available that show a high sensitivity in the infrared spectrum up to 1400 nm. Now, these photomultipliers are successfully and frequently used for 1O2 detection.28–34
Several groups world-wide have undertaken much effort to optimize the detection technology and to gain more detailed information from such 1O2 luminescence signals.35 The detection of 1O2 luminescence in living cells or tissue has proven to be a technically challenging problem for the following reasons. Due to low oxygen concentrations,29,36 short 1O2 lifetime,37 and changing oxygen diffusion coefficients in cells or tissue,28 the signal intensity may be substantially weaker than in solution, showing diverse rates. Scattering in turbid media, e.g.cell suspensions, may lead to an increased superposition of the signals with other radiation sources, which was recently investigated by the groups of Wilson29 and Röder.28 In addition, flash photolysis experiments can be applied for triplet spectroscopy and the results can be compared to singlet oxygen luminescence at 1270 nm.28 Spatially resolved singlet oxygen luminescence were detected either on a microscopic scale, in particular in living cells,38 or in vivo using a scanning laser system.39
Using excitation lasers with short pulse durations and kHz repetition rates, along with the highly sensitive IR photomultipliers, the single photon counting allows very sensitive luminescence detection with a high time resolution. This technique, meanwhile rather standardized, shows advantages over the conventional analogue detection mode that was used in the past.40
To generate 1O2 by UVA radiation, the emission of a frequency tripled Nd:YAG laser (355 nm) or an appropriate OPO laser can be applied to excite endogenous photosensitizers. The laser pulse energy for luminescence experiments should be kept low (a few μJ) to minimize the damage of photosensitizer and cellular structures.28 The 1O2 luminescence at 1270 nm can be detected in either perpendicular or near-backward direction with respect to the excitation beam using infrared sensitive photomultipliers (e.g. Hamamatsu).15,28,29,33,38 To avoid detection of non-1O2photons, appropriate interference filters and cut-off-filters are usually placed in front of the photomultiplier (Fig. 4).
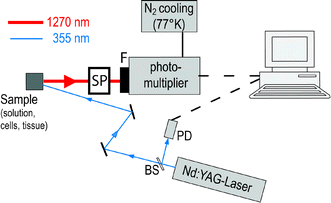 |
| Fig. 4 A schematic setting for detection of singlet oxygen luminescence. The sample is excited with a frequency tripled Nd:YAG laser that emits at 355 nm. The luminescence is collected and transferred to the infrared sensitive photomultiplier, which is equipped with a 1270 nm interference filter. The single photon counting setting enables the time-resolved detection, whereas the stop signal is provided by a photodiode (PD): via beam splitter (BS). The spectral resolution is either achieved with different interference filters from 1100 to 1400 nm or by using a spectrometer (SP). | |
Due to the very low luminescence signals, which is potentially superposed by other near infrared signal such as fluorescence or phosphorescence, it is important to detect the luminescence signal spectrally resolved in order to confirm that the signal can be attributed to 1O2.29,41,42 When combining the time- and spectrally resolved luminescence, the resulting 3D-image provides evidence for clear luminescence signal of 1O2, an example is displayed in Fig. 5.
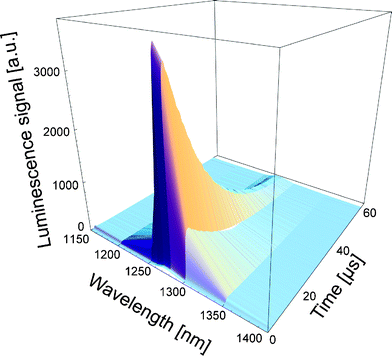 |
| Fig. 5 The time- and spectrally resolved signal can be combined to have a clear confirmation of 1O2 signal. This is important in case of weak luminescence signals, in particular from cells and tissue. | |
Mathematical fit of time-resolved singlet oxygen luminescence
After detection of such time resolved signals, it is a first and practical step to fit the gained signal curve using a constant C, the rise (τR) and decay (τD) times as well as different fit routines such as least square fit.43 |  | (1) |
When applying different experiments, the values can be attributed to the respective rates and rate constants in the respective experiment.42 The counted luminescence photons can be summed to calculate the total amount of 1O2 detected. The spectrally resolved luminescence signal, either using a monochromator34 or different interference filters,36 is fitted with a Lorentzian function.
The time resolved luminescence signal provides information about the generation and deactivation of 1O2 in its present environment. The in-depth evaluation of time-resolved luminescence signals can be performed along with mathematical equations, which describe the generation and decay of 1O2.29,42 Briefly, the coupling of a photosensitizer molecule with molecular oxygenvia energy transfer from its triplet T1 state to molecular oxygen yields a coupled system of differential equations, which can be reduced to the rate constants of the triplet T1 state or first 1O2 state:
| 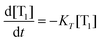 | (2) |
| 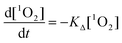 | (3) |
The signal will rise with the largest of the two rates and decay with the lowest. The assignment of the measured rise (
τR) and decay (
τD) times to the rates of
1O
2 or photosensitizer T
1 state remains challenging.
In non-viscous media (e.g.solvents), for high oxygen concentrations, the rise time is equivalent to KT and the decay time to KΔ, whereas for small oxygen concentrations it is the other way around. After this classification, the rates can be assigned to the respective lifetimes. In highly viscous media (e.g. in cells or tissue), the diffusion of oxygen molecules plays an additional role that complicates the evaluation of time resolved measurements and the assignment of the lifetimes.
The rates are intrinsically tied to the concentration and diffusion of oxygen in the environment of 1O2 production. When evaluating such luminescence signals, it is therefore important to know, to measure or to estimate the oxygen concentration at the site of 1O2 production during luminescence detection. In solution or cell suspensions, small needle sensors can be applied to monitor the oxygen concentration.44
Quantification of singlet oxygen
To quantify the 1O2 generation of endogenous photosensitizers, the quantum yield ΦΔ can be determined by using the Wilkinson definition45 or by comparing the luminescence signals of the photosensitizer with unknown ΦΔ to photosensitizer with known ΦΔ.15 Values are listed for a selection of photosensitizers in Table 2. It should be noted that the quantum yields are predominantly determined for photosensitizers in aerated solvents. In case of low oxygen concentrations (e.g. inside cells or tissue), ΦΔ values may clearly differ from those values as shown in Table 2. For example, ΦΔ of Riboflavin in solution decreases from 0.54 (oxygen concentration: 280 μM) to about 0.20 (oxygen concentration: 2 μM).15 This decrease can be different for different photosensitizers.
4. Photosensitized generation of singlet oxygen
In contrast to UVB, UVA radiation mainly provokes photosensitized reactions.46 The major prerequisite of photosensitized reactions is UVA absorption in such molecules. The list of molecules has been fairly extended during recent years. It starts with endogenous porphyrins of heme synthesis, flavins, and the cellular pyrimidine nicotinamide cofactors (NADH and NADPH).47 Meanwhile, the list also contains exogenous molecules, which are frequently administered to skin along with medical treatments. The papers cited below represent a non-exhausting selection of endogenous photosensitizers.
Endogenous photosensitizers
Among others, endogenous porphyrin molecules such as Protoporphyrin IX, the precursor of heme, efficiently generate 1O2 under UVA irradiation leading to activation of heme oxygenase-1 (HO-1).48
Urocanic acid produces 1O2 with UVA irradiation (355 nm) that was directly proven by luminescence signal.15 In the same work, excitation with UVA of Riboflavin, FMN and FAD yielded strong luminescence signals and the respective quantum yields could be determined with ΦΔ = 0.54 (riboflavin), ΦΔ = 0.51 (FMN), and ΦΔ = 0.07 (FAD). Depending on their concentration in the skin, the flavins are potential generators of 1O2, even more effective than exogenous porphyrins used for cell killing in photodynamic therapy. In view of these high values, it seems to be reasonable that these substances, even though at low concentrations, can provide sufficient amount of 1O2 during UVA exposure that leads to gene regulation, photoaging, and possibly carcinogenesis.
The human retinal pigment epithelial (RPE) layer contains a complex mixture of components called lipofuscin; this mixture forms with age and with various genetic disorders such as Stargardt's disease. It is well accepted that lipofucsin generates 1O2 when excited with UVA, which contributes to retinal maculopathies.49
The protein phosphatase calcineurin has been gradually revealing itself as the central controller of our immune response. UVA1 radiation suppresses calcineurin activity. Evidence was provided that this activity loss is partly due to 1O2 generated by photosensitization.50 Recently, experiments showed that the malondialdehyde-derived protein epitope dihydropyridine (DHP)-lysine is a potent endogenous UVA-photosensitizer of human skin cells.51
The photophysics and photochemistry of thiopurine DNA bases are far less understood than those of normal DNA bases, although some of them, such as azathioprine, 6-mercaptopurine, and 6-thioguanine, have been used as cancer therapeutic and immunosuppressive agents for five decades. The incorporation of 6-thioguanine into DNA increases the risk of 1O2-initiated skin cancer. It was very recently shown that UVA irradiation of 6-thioguanines in solution produced 1O2 with quantum yields ΦΔ from 0.49 to 0.58.52 6-Thioguanines absorb UVA radiation in a broad range from 320–370 nm with a maximum at around 340 nm.
Pterins (2-amino-4-hydroxypteridin derivatives) are a family of heterocyclic compounds present in a wide variety of biological systems. Pteroyl-L-glutamic acid (folic acid) is a precursor of coenzymes involved in the metabolism of nucleotides and amino acids. All investigated pterins produced significantly amounts of 1O2 with ΦΔ in the range from 0.30 to 0.47 (pD Value 10.5). Only folic acid showed a very small quantum yield of less than 0.02.53
Anhydroretinol is a metabolite of vitamin A (retinol) and a major photodecomposition product of retinyl palmitate and retinyl acetate. There is sufficient evidence that irradiation of anhydroretinol with UVA light generates reactive oxygen species, e.g.1O2, which mediate the induction of lipid peroxidation.54
Fluorescent proteins are increasingly applied in different sections of experimental biology. One of those techniques is chromophore-assisted laser inactivation, which is employed to specifically inactivate the function of target proteins or organelles by producing photochemical damage.55 Using time resolved luminescence detection, singlet oxygen was proven to be generated by the red fluorescent protein TagRFP with an estimated quantum yield of 0.004.
Many substances, which are used in antibiotics such as quinoline derivatives,56 are known to generate 1O2 under UVA exposure. Ciprofloxacin produced ROS by Type I and Type II photodynamic reactions, interacted with nucleic acid moiety and inhibited cell viability.25 The production of 1O2 by various antibiotics was found to be concentration dependent. In the fluoroquinolone group, enoxacin generated most 1O2 under UVA irradiation followed by lomefloxacin, norfloxacin, and ofloxacin.57 In light of the undesirable photosensitized reactions of fluoroquinolones, new selenium-containing heterocyclic compounds were investigated. However, also these substances produced 1O2.58
Other medical drugs
Several classes of drugs including thiazide diuretics, nonsteroidal anti-inflammatory drugs (NSAIDs), and tricyclic antidepressants, even when not toxic by themselves, may become reactive under exposure to environmental radiation, inducing undesired side effects. Providing a few examples, the following section should highlight the problems with 1O2 that is generated by medical drugs exposed to UVA.
Nonsteroidal anti-inflammatory drugs (NSAIDs) are a chemically heterogeneous group of drugs mainly used as anti-inflammatory particularly in the treatment of rheumatic diseases. Some of the NSAID are potent photosensitizers when exposed to UVA radiation.59 Due to the presence of the naphthalene chromophore, substances like Nabumetone or Naproxen generate 1O2 with quantum yields of 0.19 or 0.28 in solution.60
The neuroleptic drug levomepromazine (known as methotrimeprazine) is photolabile under UVA and UVB radiation in aerobic conditions. Irradiation of a methanol solution of this drug produces one photoproduct, resulting from oxidation. It is demonstrated that photodegradation occurs via type II mechanism involving irreversible trapping of self-photogenerated singlet molecular oxygen.61Lamotrigine (LTG) is an anticonvulsant (antiepileptic) drug specifically blocking voltage-gated sodium channels. LTG occasionally causes cutaneous problems including exaggerated sunburn or skin photo-irritation. Depending on the solvent, the quantum yield ΦΔ of LTG shows values up to 0.22.62Chlorpromazine (CPZ), a phenothiazine derivative, is a neuroleptic drug widely used in medicine because of its tranquilizing and antipsychotic properties. Experimental data indicate that in hydrophobic environment CPZ is a relatively efficient generator of 1O2.63
Imidazopyridine derivatives are designed as 5-HT4 receptor agonist for the clinical treatment of gastroesophageal reflux disease. Also these substances produce 1O2 under UVA exposure.64Dihydropyridine-type calcium channel antagonists such as nifedipine and nitrendipine are important drugs for the treatment of hypertension and coronary heart disease. A novel substance and some of the derivates produced significant amounts of 1O2 under simulated solar radiation.65
Nano-materials such as water-soluble fullerenes have shown potential uses as drug carriers to bypass the brain and ocular barriers. However, photoexcitation of fullerene derivatives may produce 1O2.66 The water-soluble fullerene derivative γ-cyclodextrin bicapped C60 efficiently generates 1O2 under UVA exposure. Since this compound has an absorbance maximum of 349 nm, it has the potential to cause lenticular damage when in the human eye.67
Other exogenous compounds
Polycyclic aromatic hydrocarbons (PAHs) are widely spread substances in environment that have been identified as essential risk factors for various benign or malignant human diseases, either alone or in combination with UVA. Specific sources are cigarette smoke and diesel particles extracts (DPE). In vitro experiments showed that sodium azide significantly inhibited both cellular and DNA damage induced by DPE + UVA treatment, which provide evidence of 1O2 generation by PAHs 68
PAHs are injected into the skin along with black tattoo inks and may stay intradermally for years. After incubation of skin cells with extracts of black tattoo inks, the cells were exposed to UVA and cell viability decreased in a dose-dependent manner. The quantum yield ΦΔ of different PAHs were determined yielding high values of up to 0.85 for benz[a]anthracene.12
Nanoparticles such as silicon nanoparticles can generate 1O2 on its surface via energy transfer from an exciton singlet state to oxygen.69 This might be of particular interest when using nanoparticles in ointments and medical drugs.
5.
UVA-mediated singlet oxygen generation
Besides the photosensitized generation triggered by UVA, 1O2 can be produced by various chemical reactions involving different radicals and other reactive species.70 In the presence of oxidizable biomolecules like lipids, proteins or DNA, photosensitization and chemical reactions (e.g.lipid peroxidation) may occur at the same time yielding various products.71,72
Usually lipids and fatty acids are the target of 1O2 that has been generated by any photosensitizer. However, we have shown that 1O2 is generated in suspensions of egg yolk phosphatidylcholine during irradiation with UVA that included the action of oxygen radicals.36 Under 355 nm irradiation, different fatty acids (oleic acid 18
:
1, linoleic acid 18
:
2, linolenic acid 18
:
3) in aerated ethanol solution (50 mmol L−1 each) showed clear 1O2 luminescence signals, without any photosensitizer (Fig. 6), which was confirmed by the spectrally resolved detection of the signals (Fig. 7). The decay time of the signal τΔ = (KΔ)−1 was in the range from 13 to 14 μs, which is the life time of 1O2 in ethanol.42
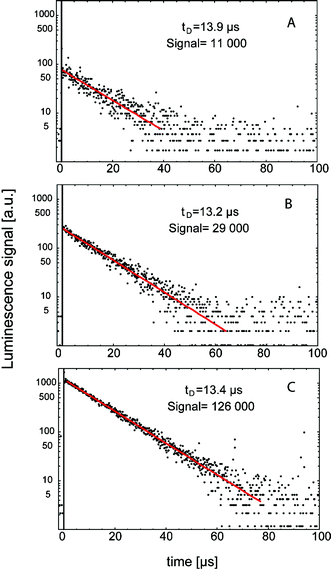 |
| Fig. 6 The time-resolved 1O2 luminescence signals of different fatty acids, oleic acid 18 : 1 (A), linoleic acid 18 : 2 (B), linolenic acid 18 : 3 (C) in aerated ethanol solution at 50 mmol L−1. The decay time tD and the values of integrated signal are shown (Signal, arbitrary units). | |
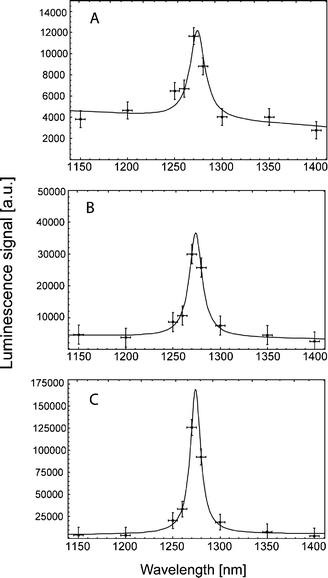 |
| Fig. 7 The spectrally resolved 1O2 luminescence signals of different fatty acids, oleic acid 18 : 1 (A), linoleic acid 18 : 2 (B), linolenic acid 18 : 3 (C) in aerated ethanol solution at 50 mmol L−1. All signals show the transition of 1O2 at 1270 nm. | |
Oxidized products of such fatty acids must be present to enable initial absorption of UVA radiation.73 Once singlet oxygen is generated, the amount of oxidized products increases, which in turn enhances radiation absorption. Usually, luminescence signals of photosensitizer induced 1O2 show a rise time and a decay time according to the eqn (1)–(3). However, time-resolved luminescence signals of 1O2 in fatty acid solutions showed a decay time but no rise time. That is, the luminescence signal was maximal within a time span of less than 200 ns. The difference of such signals shapes, with and without rise time, is illustrated in Fig. 8. A photosensitized generation of 1O2 by a porphyrin photosensitizer in water shows a clear rise time, which is τT = (KT)−1 = 1.9 μs, whereas the decay time was τΔ = (KΔ)−1 = 3.6 μs. We suggested that due to the lack of rise time, the light absorbing molecules are not able to form such a triplet state (e.g. linear-shaped molecules like fatty acids). Thus, we assumed that 1O2 is generated with the assistance of chemical reactions, but initiated by the applied UVA radiation.74
In addition, hydroperoxides of fatty acids can be further decomposed to acid-peroxyl radicals and/or alkoxyl radicals, which are responsible for the propagation of peroxidation.75 The decomposition of lipid hydroperoxides into peroxyl radicals has been shown to be a potential source of 1O2 in biological systems.3,76 In 1957, Russell proposed a self-reaction mechanism of such peroxyl radicals involving the formation of a cyclic mechanism from a linear tetraoxide intermediate that decomposes to give different products as well as 1O2.77 It could be demonstrated that such a self-reaction formation of peroxyl radicals deriving from fatty acids generates predominantly 1O2.78Fig. 9 shows a scheme of UVA-mediated generation of 1O2 generation in fatty acids, which is probably not yet complete.
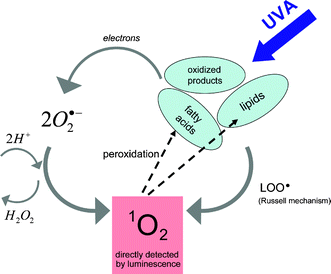 |
| Fig. 9 Proposed scheme of UVA-initiated singlet oxygen generation with the assistance of radicals (taken from reference Baier et al.74). | |
Such fatty acids are major constituents of many cellular membranes that should underline their potential role in UVA mediated activation of cellular signaling. Skin contains sufficient amount of oxygen (pO2 ∼ 20 Torr).79 When exposed to UVA radiation, initial concentrations of oxidized fatty acids are present in skin80,81 to initiate the generation of 1O2 (see Fig. 9). In cells, a molecule such as ceramide is a key component of stress responses. UVA radiation and 1O2 both generated ceramide in protein-free, sphingomyelin-containing liposomes.82 Furthermore, human skin, especially the stratum corneum, contains free saturated and unsaturated fatty acids with mostly chain lengths of C16 to C18 atoms.83
Fatty acids are also constituents of creams and ointments, in particular used in sunscreens that protect skin from solar UV radiation. When exposing different creams or ointments in ethanol solution to UVA laser radiation at 355 nm, we detected a clear and impressive signal of 1O2 generation in many of the samples investigated. As for experiments with fatty acids, the decay time of 1O2 was about 14 μs according to the used solvent ethanol. The maximum signal intensity among the investigated creams yielded the sample shown in Fig. 10. The impact of such photosensitizing compounds, which are topically administered to skin and exposed to solar radiation, should be elucidated.
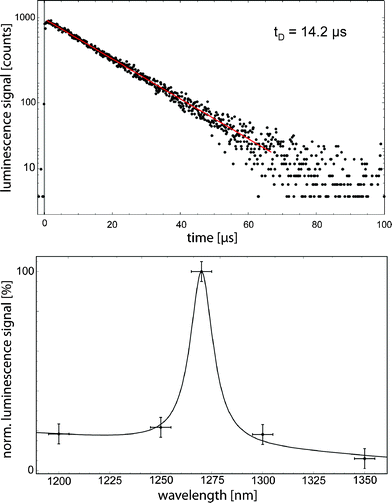 |
| Fig. 10 The clear and impressive time- and spectrally resolved 1O2 luminescence signals of skin moisture. | |
6.
Detection of singlet oxygen generation in skin
Solar ultraviolet A (UVA; 320–400 nm) radiation is a well-known trigger of signaling responses in dermal fibroblasts in human skin in vivo.48,84 Investigations have been performed to assess the contribution of 1O2 to lipid peroxidation under in vivo conditions. It is known that the generation of 1O2 in turn activates interstitial collagenase like matrix-metalloproteinases (MMPs)80,85 such as MMP-1, which causes extracellular protein degradation and thereby contributes to photoaging of human skin.86 The activation of MMP-9 is an essential step in the skin photoaging on exposure to ultraviolet A (UVA). MMP-9 activity is clearly related to the presence of cholesterol-hydroperoxides such as cholesterol 5α-hydroperoxide, which is specifically occurs with 1O2.87Gene expression in keratinocytes, which is induced by solar UVA radiation, is initiated at the level of the cell membranevia generation of 1O2 and subsequent formation of ceramide from sphingomyelin.88
Additionally, UVA radiation is now recognized as a class I carcinogen89 and is suspected to play a significant role in the induction of melanoma,90,91 although the latter is still a controversial issue.921O2 is clearly induced in living skin by UVA radiation, but one has to consider that the quantum yield ΦΔ depends critically on the respective oxygen concentration, which is in skin substantially smaller as compared to aerated solutions (Fig. 11).15
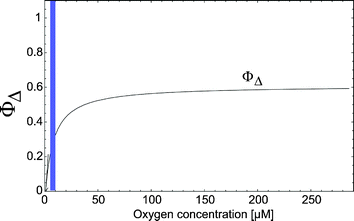 |
| Fig. 11 The quantum yield of 1O2 generation for different oxygen concentrations. The vertical bar indicates the oxygen concentration in a living cell (taken from Schenkman et al.103). | |
In light of these facts, there is a need for a detection system that monitors the generation of 1O2 in tissue in vivo for different experimental conditions. The results should be correlated to the biological findings. By means of the highly sensitive luminescence detection, we succeeded in detecting 1O2 in living cells and even in skin in vivo, without any exogenous photosensitizer.36 At present, the luminescence technology seems to be the only method to detect 1O2in vivo since it is difficult or impossible to apply quencher or other reporter substances.
Conclusion
The direct detection of 1O2 by its luminescence is a fascinating tool, which has been used in photodynamic therapy and UVA-mediated photoreactions. The luminescence may report on the generation and the lifetime of 1O2 in various environments. Due to the weak signal intensity, the luminescence detection was limited to experiments in solution at the beginning. For more than ten years, the development of new infrared sensitive photomultipliers has enabled many researchers to extend such luminescence detection to living cellsin vitro and to tissue in vivo. However, there is still need to improve this technology, e.g. by looking for detectors that are even more sensitive.
• The high sensitivity of new detection technology should be used to reduce the excitation energy, which still affects the cell integrity during such experiments.
• The luminescence curve appears as a simple signal but contains a lot of information about 1O2 dynamics, which requires a careful analysis and interpretation.
• In the case of living cells, it would be of great importance to develop a CCD camera-like detector of 1O2 that allows the luminescence detection with spatial resolution.
• Computer-assisted modeling may help to simulate the diffusion of excitation and luminescence photons as well as the movement of 1O2 in cells.
Acknowledgements
This study was partly supported by the Deutsche Forschungsgeneinschaft (DFG, grant BA 1741/3-2).
References
- S. L. Parker, T. Tong, S. Bolden and P. A. Wingo, Cancer statistics, Ca-Cancer J. Clin., 1997, 47, 5–27 Search PubMed.
- S. Seite, A. Fourtanier, D. Moyal and A. R. Young, Photodamage to human skin by suberythemal exposure to solar ultraviolet radiation can be attenuated by sunscreens: a review, Br. J. Dermatol., 2010, 163, 903–914 CrossRef CAS.
- S. Miyamoto, G. R. Martinez, M. H. Medeiros and P. Di Mascio, Singlet molecular oxygen generated from lipid hydroperoxides by the russell mechanism: studies using 18(O)-labeled linoleic acid hydroperoxide and monomol light emission measurements, J. Am. Chem. Soc., 2003, 125, 6172–6179 CrossRef CAS.
- C. A. McCarty and H. R. Taylor, Recent developments in vision research: light damage in cataract, Invest. Ophthalmol. Vis. Sci., 1996, 37, 1720–1723 CAS.
- D. Mitchell, Revisiting the photochemistry of solar UVA in human skin, Proc. Natl. Acad. Sci. U. S. A., 2006, 103, 13567–13568 CrossRef CAS.
- K. Wertz, P. B. Hunziker, N. Seifert, G. Riss, M. Neeb, G. Steiner, W. Hunziker and R. Goralczyk, beta-Carotene interferes with ultraviolet light A-induced gene expression by multiple pathways, J. Invest. Dermatol., 2005, 124, 428–434 CrossRef CAS.
- S. Mouret, C. Baudouin, M. Charveron, A. Favier, J. Cadet and T. Douki, Cyclobutane pyrimidine dimers are predominant DNA lesions in whole human skin exposed to UVA radiation, Proc. Natl. Acad. Sci. U. S. A., 2006, 103, 13765–13770 CrossRef CAS.
- T. Hasan, F. Nyberg, E. Stephansson, P. Puska, M. Hakkinen, S. Sarna, A. M. Ros and A. Ranki, Photosensitivity in lupus erythematosus, UV photoprovocation results compared with history of photosensitivity and clinical findings, Br. J. Dermatol., 1997, 136, 699–705 CrossRef CAS.
- P. Autier, J. F. Dore, A. M. Eggermont and J. W. Coebergh, Epidemiological evidence that UVA radiation is involved in the genesis of cutaneous melanoma, Curr. Opin. Oncol., 2011, 23, 189–196 CrossRef.
- A. K. von Thaler, Y. Kamenisch and M. Berneburg, The role of ultraviolet radiation in melanomagenesis, Exp. Dermatol., 2010, 19, 81–88 CrossRef CAS.
- N. J. Neumann, A. Blotz, G. Wasinska-Kempka, M. Rosenbruch, P. Lehmann, H. J. Ahr and H. W. Vohr, Evaluation of phototoxic and photoallergic potentials of 13 compounds by different in vitro and in vivo methods, J. Photochem. Photobiol., B, 2005, 79, 25–34 CrossRef CAS.
- J. Regensburger, K. Lehner, T. Maisch, R. Vasold, F. Santarelli, E. Engel, A. Gollmer, B. Konig, M. Landthaler and W. Baumler, Tattoo inks contain polycyclic aromatic hydrocarbons that additionally generate deleterious singlet oxygen, Exp. Dermatol., 2009, 19, e275–281 CrossRef.
- C. A. Morton, K. E. McKenna and L. E. Rhodes, Guidelines for topical photodynamic therapy: update, Br. J. Dermatol., 2008, 159, 1245–1266 CrossRef CAS.
- T. Maisch, S. Hackbarth, J. Regensburger, A. Felgentrager, W. Baumler, M. Landthaler and B. Roder, Photodynamic inactivation of multi-resistant bacteria (PIB) - a new approach to treat superficial infections in the 21(st) century, J. Dtsch. Dermatol. Ges., 2011 Search PubMed.
- J. Baier, T. Maisch, M. Maier, E. Engel, M. Landthaler and W. Baumler, Singlet Oxygen Generation by UVA Light Exposure of Endogenous Photosensitizers, Biophys. J., 2006, 91, 1452–1459 CrossRef CAS.
- R. S. Sohal and R. Weindruch, Oxidative stress, caloric restriction, and aging, Science, 1996, 273, 59–63 CAS.
- K. M. Hanson and J. D. Simon, Epidermal trans-urocanic acid and the UV-A-induced photoaging of the skin, Proc. Natl. Acad. Sci. U. S. A., 1998, 95, 10576–10578 CrossRef CAS.
- P. W. Albro, P. Bilski, J. T. Corbett, J. L. Schroeder and C. F. Chignell, Photochemical reactions and phototoxicity of sterols: novel self-perpetuating mechanisms for lipid photooxidation, Photochem. Photobiol., 1997, 66, 316–325 CrossRef.
- Q. Xia, J. J. Yin, P. P. Fu and M. D. Boudreau, Photo-irradiation of Aloe vera by UVA–formation of free radicals, singlet oxygen, superoxide, and induction of lipid peroxidation, Toxicol. Lett., 2007, 168, 165–175 CrossRef CAS.
- C. Schweitzer and R. Schmidt, Physical mechanisms of generation and deactivation of singlet oxygen, Chem. Rev., 2003, 103, 1685–1757 CrossRef CAS.
- J. Wassell, S. Davies, W. Bardsley and M. Boulton, The photoreactivity of the retinal age pigment lipofuscin, J. Biol. Chem., 1999, 274, 23828–23832 CrossRef CAS.
- A. W. Girotti and T. Kriska, Role of lipid hydroperoxides in photo-oxidative stress signaling, Antioxid. Redox Signaling, 2004, 6, 301–310 Search PubMed.
- A. Morita, T. Werfel, H. Stege, C. Ahrens, K. Karmann, M. Grewe, S. Grether-Beck, T. Ruzicka, A. Kapp, L. O. Klotz, H. Sies and J. Krutmann, Evidence that singlet oxygen-induced human T helper cell apoptosis is the basic mechanism of ultraviolet-A radiation phototherapy, J. Exp. Med., 1997, 186, 1763–1768 CrossRef CAS.
- M. Y. Jung and D. B. Min, ESR study of the singlet oxygen quenching and protective activity of Trolox on the photodecomposition of riboflavin and lumiflavin in aqueous buffer solutions, J. Food Sci., 2009, 74, C449–455 CrossRef CAS.
- N. Agrawal, R. S. Ray, M. Farooq, A. B. Pant and R. K. Hans, Photosensitizing potential of ciprofloxacin at ambient level of UV radiation, Photochem. Photobiol., 2007, 83, 1226–1236 CrossRef CAS.
- D. Ali, R. S. Ray and R. K. Hans, UVA-induced cyototoxicity and DNA damaging potential of benz (e) acephenanthrylene, Toxicol. Lett., 2010, 199, 193–200 CrossRef CAS.
- D. Costa, E. Fernandes, J. L. Santos, D. C. Pinto, A. M. Silva and J. L. Lima, New noncellular fluorescence microplate screening assay for scavenging activity against singlet oxygen, Anal. Bioanal. Chem., 2007, 387, 2071–2081 CrossRef CAS.
- S. Hackbarth, J. Schlothauer, A. Preuss and B. Roder, New insights to primary photodynamic effects–Singlet oxygen kinetics in living cells, J. Photochem. Photobiol., B, 2010, 98, 173–179 CrossRef CAS.
- M. T. Jarvi, M. J. Niedre, M. S. Patterson and B. C. Wilson, The influence of oxygen depletion and photosensitizer triplet-state dynamics during photodynamic therapy on accurate singlet oxygen luminescence monitoring and analysis of treatment dose response, Photochem. Photobiol., 2011, 87, 223–234 CrossRef CAS.
- A. Jimenez-Banzo, X. Ragas, S. Abbruzzetti, C. Viappiani, B. Campanini, C. Flors and S. Nonell, Singlet oxygen photosensitisation by GFP mutants: oxygen accessibility to the chromophore, Photochem. Photobiol. Sci., 2010, 9, 1336–1341 RSC.
- P. R. Ogilby, Singlet oxygen: there is still something new under the sun, and it is better than ever, Photochem. Photobiol. Sci., 2010, 9, 1543–1560 RSC.
- J. Baier, M. Maier, R. Engl, M. Landthaler and W. Baumler, Time-resolved investigations of singlet oxygen luminescence in water, in phosphatidylcholine, and in Aqueous suspensions of phosphatidylcholine or HT29 cells, J. Phys. Chem. B, 2005, 109, 3041–3046 CrossRef.
- J. Regensburger, T. Maisch, A. Felgentrager, F. Santarelli and W. Baumler, A helpful technology–the luminescence detection of singlet oxygen to investigate photodynamic inactivation of bacteria (PDIB), J. Biophotonics, 2010, 3, 319–327 CrossRef CAS.
- S. Miyamoto, G. R. Martinez, A. P. Martins, M. H. Medeiros and P. Di Mascio, Direct evidence of singlet molecular oxygen [O2(1Deltag)] production in the reaction of linoleic acid hydroperoxide with peroxynitrite, J. Am. Chem. Soc., 2003, 125, 4510–4517 CrossRef CAS.
- J. R. Kanofsky, Measurement of singlet-oxygen in vivo: progress and pitfalls, Photochem. Photobiol., 2011, 87, 14–17 CrossRef CAS.
- J. Baier, T. Maisch, M. Maier, M. Landthaler and W. Baumler, Direct Detection of Singlet Oxygen Generated by UVA Irradiation in Human Cells and Skin, J. Invest. Dermatol., 2007, 127, 1498–1506 CrossRef CAS.
- A. Baker and J. R. Kanofsky, Quenching of singlet oxygen by biomolecules from L1210 leukemia cells, Photochem. Photobiol., 1992, 55, 523–528 CrossRef CAS.
- M. K. Kuimova, G. Yahioglu and P. R. Ogilby, Singlet oxygen in a cell: spatially dependent lifetimes and quenching rate constants, J. Am. Chem. Soc., 2009, 131, 332–340 CrossRef CAS.
- M. J. Niedre, M. S. Patterson, A. Giles and B. C. Wilson, Imaging of photodynamically generated singlet oxygen luminescence in vivo, Photochem. Photobiol., 2005, 81, 941–943 CrossRef CAS.
- A. Jimenez-Banzo, X. Ragas, P. Kapusta and S. Nonell, Time-resolved methods in biophysics. 7. Photon counting vs. analog time-resolved singlet oxygen phosphorescence detection, Photochem. Photobiol. Sci., 2008, 7, 1003–1010 RSC.
- M. Niedre, M. S. Patterson and B. C. Wilson, Direct near-infrared luminescence detection of singlet oxygen generated by photodynamic therapy in cells in vitro and tissues in vivo, Photochem. Photobiol., 2002, 75, 382–391 CAS.
- J. Baier, T. Fuß, C. Pöllmann, C. Wiesmann, K. Pindl, R. Engl, D. Baumer, M. Maier, M. Landthaler and W. Bäumler, Theoretical and experimental analysis of the luminescence signal of singlet oxygen for different photosensitizers, J. Photochem. Photobiol., B, 2007, 87, 163–173 CrossRef CAS.
- D. Baumer, M. Maier, R. Engl, R. M. Szeimies and W. Baumler, Singlet oxygen generation by 9-acetoxy-2,7,12,17-tetrakis-(Beta-methoxyethyl)-porphycene (ATMPn) in solution, Chem. Phys., 2002, 285, 309–318 CrossRef CAS.
- T. Maisch, J. Baier, B. Franz, M. Maier, M. Landthaler, R. M. Szeimies and W. Bäumler, The role of singlet oxygen and oxygen concentration in photodynamic inactivation of bacteria, Proc. Natl. Acad. Sci. U. S. A., 2007, 104, 7223–7228 CrossRef CAS.
- F. Wilkinson, W. P. Helman and A. B. Ross, Rate constants for the decay and reactions of the lowest electronically excited singlet state of molecular oxygen in solution. An expanded and revised compilation, J. Phys. Chem. Ref. Data, 1995, 24, 663–1021 CrossRef CAS.
- J. Cadet, T. Douki, J. L. Ravanat and P. Di Mascio, Sensitized formation of oxidatively generated damage to cellular DNA by UVA radiation, Photochem. Photobiol. Sci., 2009, 8, 903–911 RSC.
- M. Dalle Carbonare and M. A. Pathak, Skin photosensitizing agents and the role of reactive oxygen species in photoaging, J. Photochem. Photobiol., B, 1992, 14, 105–124.
- R. M. Tyrrell, Solar ultraviolet A radiation: an oxidizing skin carcinogen that activates heme oxygenase-1, Antioxid. Redox Signal., 2004, 6, 835–840 CAS.
- L. B. Avalle, J. Dillon, S. Tari and E. R. Gaillard, A new approach to measuring the action spectrum for singlet oxygen production by human retinal lipofuscin, Photochem. Photobiol., 2005, 81, 1347–1350 CrossRef CAS.
- R. E. Musson, P. J. Hensbergen, A. H. Westphal, W. P. Temmink, A. M. Deelder, J. van Pelt, L. H. Mullenders and N. P. Smit, UVA1 radiation inhibits calcineurin through oxidative damage mediated by photosensitization, Free Radical Biol. Med., 2011 Search PubMed.
- S. D. Lamore, S. Azimian, D. Horn, B. L. Anglin, K. Uchida, C. M. Cabello and G. T. Wondrak, The malondialdehyde-derived fluorophore DHP-lysine is a potent sensitizer of UVA-induced photooxidative stress in human skin cells, J. Photochem. Photobiol., B, 2010, 101, 251–264 CrossRef CAS.
- Y. Zhang, X. Zhu, J. Smith, M. T. Haygood and R. Gao, Direct observation and quantitative characterization of singlet oxygen in aqueous solution upon UVA excitation of 6-thioguanines, J. Phys. Chem. B, 2011, 115, 1889–1894 CrossRef CAS.
- A. H. Thomas, C. Lorente, A. L. Capparelli, C. G. Martinez, A. M. Braun and E. Oliveros, Singlet oxygen (1deltag) production by pterin derivatives in aqueous solutions, Photochem. Photobiol. Sci., 2003, 2, 245–250 RSC.
- J. J. Yin, Q. Xia and P. P. Fu, UVA photoirradiation of anhydroretinol–formation of singlet oxygen and superoxide, Toxicol. Ind. Health, 2007, 23, 625–631 CrossRef CAS.
- X. Ragas, L. P. Cooper, J. H. White, S. Nonell and C. Flors, Quantification of photosensitized singlet oxygen production by a fluorescent protein, ChemPhysChem, 2011, 12, 161–165 CrossRef CAS.
- S. Jantova, K. Konarikova, S. Letasiova, E. Paulovicova, V. Milata and V. Brezova, Photochemical and phototoxic properties of ethyl 1,4-dihydro-8-nitro-4-oxoquinoline-3-carboxylate, a new quinoline derivative, J. Photochem. Photobiol., B, 2011, 102, 77–91 CrossRef CAS.
- R. S. Ray, N. Agrawal, R. B. Misra, M. Farooq and R. K. Hans, Radiation-induced in vitro phototoxic potential of some fluoroquinolones, Drug Chem. Toxicol., 2006, 29, 25–38 CrossRef CAS.
- Z. Barbierikova, M. Bella, J. Kucerak, V. Milata, S. Jantova, D. Dvoranova, M. Vesela, A. Stasko and V. Brezova, Photoinduced superoxide radical anion and singlet oxygen generation in the presence of novel selenadiazoloquinolones (an EPR Study), Photochem. Photobiol., 2011, 87, 32–44 CrossRef CAS.
- B. Quintero and M. A. Miranda, Mechanisms of photosensitization induced by drugs: A general survey, Ars Pharmaceutica, 2000, 41, 27–46 Search PubMed.
- L. J. Martinez and J. C. Scaiano, Characterization of the transient intermediates generated from the photoexcitation of nabumetone: a comparison with naproxen, Photochem. Photobiol., 1998, 68, 646–651 CrossRef CAS.
- F. Vargas, K. Carbonell and M. Camacho, Photochemistry and in vitro phototoxicity studies of levomepromazine (methotrimeprazine), a phototoxic neuroleptic drug, Pharmazie, 2003, 58, 315–319 CAS.
- P. J. Bilski, M. A. Wolak, V. Zhang, D. E. Moore and C. F. Chignell, Photochemical reactions involved in the phototoxicity of the anticonvulsant and antidepressant drug lamotrigine (Lamictal), Photochem. Photobiol., 2009, 85, 1327–1335 CrossRef CAS.
- A. Wolnicka-Glubisz, M. Lukasik, A. Pawlak, A. Wielgus, M. Niziolek-Kierecka and T. Sarna, Peroxidation of lipids in liposomal membranes of different composition photosensitized by chlorpromazine, Photochem. Photobiol. Sci., 2009, 8, 241–247 RSC.
- S. Onoue, N. Igarashi, Y. Yamauchi, T. Kojima, N. Murase, Y. Zhou, S. Yamada and Y. Tsuda, In vitro phototoxic potential and photochemical properties of imidazopyridine derivative: a novel 5-HT4 partial agonist, J. Pharm. Sci., 2008, 97, 4307–4318 CrossRef CAS.
- S. Onoue, N. Igarashi, Y. Yamauchi, N. Murase, Y. Zhou, T. Kojima, S. Yamada and Y. Tsuda, In vitro phototoxicity of dihydropyridine derivatives: a photochemical and photobiological study, Eur. J. Pharm. Sci., 2008, 33, 262–270 CrossRef CAS.
- J. Kolosnjaj, H. Szwarc and F. Moussa, Toxicity studies of fullerenes and derivatives, Adv. Exp. Med. Biol., 2007, 620, 168–180 CrossRef.
- B. Zhao, Y. Y. He, C. F. Chignell, J. J. Yin, U. Andley and J. E. Roberts, Difference in phototoxicity of cyclodextrin complexed fullerene [(gamma-CyD)2/C60] and its aggregated derivatives toward human lens epithelial cells, Chem. Res. Toxicol., 2009, 22, 660–667 CrossRef CAS.
- L. Bao, A. Xu, L. Tong, S. Chen, L. Zhu, Y. Zhao, G. Zhao, E. Jiang, J. Wang and L. Wu, Activated toxicity of diesel particulate extract by ultraviolet a radiation in mammalian cells: role of singlet oxygen, Environ. Health Perspect., 2009, 117, 436–441 CAS.
- M. J. Llansola Portoles, P. M. Gara, M. L. Kotler, S. Bertolotti, E. San Roman, H. B. Rodriguez and M. C. Gonzalez, Silicon nanoparticle photophysics and singlet oxygen generation, Langmuir, 2010, 26, 10953–10960 CrossRef CAS.
- N. Krinsky, Singlet oxygen in biological systems, Trends Biochem. Sci., 1977, 2, 35–38 CrossRef CAS.
- J. Cadet, J. L. Ravanat, G. R. Martinez, M. H. Medeiros and P. Di Mascio, Singlet oxygen oxidation of isolated and cellular DNA: product formation and mechanistic insights, Photochem. Photobiol., 2006, 82, 1219–1225 CrossRef CAS.
- A. W. Girotti, Lipid hydroperoxide generation, turnover, and effector action in biological systems, J. Lipid Res., 1998, 39, 1529–1542 CAS.
- R. L. Arudi, M. W. Sutherland and B. H. Bielski, Purification of oleic acid and linoleic acid, J. Lipid Res., 1983, 24, 485–488 CAS.
- J. Baier, T. Maisch, J. Regensburger, C. Pollmann and W. Baumler, Optical detection of singlet oxygen produced by fatty acids and phospholipids under ultraviolet A irradiation, J. Biomed. Opt., 2008, 13, 044029 CrossRef.
- H. W. Gardner, Oxygen radical chemistry of polyunsaturated fatty acids, Free Radical Biol. Med., 1989, 7, 65–86 CrossRef CAS.
- S. Miyamoto, G. E. Ronsein, F. M. Prado, M. Uemi, T. C. Correa, I. N. Toma, A. Bertolucci, M. C. Oliveira, F. D. Motta, M. H. Medeiros and P. D. Mascio, Biological hydroperoxides and singlet molecular oxygen generation, IUBMB Life, 2007, 59, 322–331 Search PubMed.
- G. A. Russell, Deuterium isotope effects in the autooxidation of aralkyl hydrocarbons. Mechanisms of the interaction of peroxy radicals, J. Am. Chem. Soc., 1957, 79, 3871–3877 CrossRef.
- D. G. Mendenhall, C. Sheng and T. Wilson, Yields of excited carbonyl species from alkoxyl and from alkylperoxyl radical dismutations, J. Am. Chem. Soc., 1991, 113, 8976–8977 CrossRef.
-
H. Baumgärtl, A. Ehrly, K. Saeger-Lorenz, D. Lübbers, J. H. A. M. Ehrly and S. R. Huch, Initial results of intracutaneous measurements of pO2 profiles, in Clinical oxygen pressure measurement, ed. A. M. Ehrly, J. Hauss and R. Huch, Springer, Berlin, Heidelberg, New York, 1987, Berlin, Germany, pp. 121–128 Search PubMed.
- N. Bando, H. Hayashi, S. Wakamatsu, T. Inakuma, M. Miyoshi, A. Nagao, R. Yamauchi and J. Terao, Participation of singlet oxygen in ultraviolet-a-induced lipid peroxidation in mouse skin and its inhibition by dietary beta-carotene: an ex vivo study, Free Radical Biol. Med., 2004, 37, 1854–1863 CrossRef CAS.
- G. F. Vile and R. M. Tyrrell, UVA radiation-induced oxidative damage to lipids and proteins in vitro and in human skin fibroblasts is dependent on iron and singlet oxygen, Free Radical Biol. Med., 1995, 18, 721–730 CrossRef CAS.
- S. Grether-Beck, G. Bonizzi, H. Schmitt-Brenden, I. Felsner, A. Timmer, H. Sies, J. P. Johnson, J. Piette and J. Krutmann, Non-enzymatic triggering of the ceramide signalling cascade by solar UVA radiation, EMBO J., 2000, 19, 5793–5800 CrossRef CAS.
- M. A. Lampe, A. L. Burlingame, J. Whitney, M. L. Williams, B. E. Brown, E. Roitman and P. M. Elias, Human stratum corneum lipids: characterization and regional variations, J. Lipid Res., 1983, 24, 120–130 CAS.
- B. Catalgol, I. Ziaja, N. Breusing, T. Jung, A. Hohn, B. Alpertunga, P. Schroeder, N. Chondrogianni, E. S. Gonos, I. Petropoulos, B. Friguet, L. O. Klotz, J. Krutmann and T. Grune, The proteasome is an integral part of solar ultraviolet a radiation-induced gene expression, J. Biol. Chem., 2009, 284, 30076–30086 CrossRef CAS.
- Y. Minami, K. Yokoyama, N. Bando, Y. Kawai and J. Terao, Occurrence of singlet oxygen oxygenation of oleic acid and linoleic acid in the skin of live mice, Free Radical Res., 2008, 42, 197–204 CrossRef CAS.
- C. S. Sander, H. Chang, S. Salzmann, C. S. Muller, S. Ekanayake-Mudiyanselage, P. Elsner and J. J. Thiele, Photoaging is associated with protein oxidation in human skin in vivo, J. Invest. Dermatol., 2002, 118, 618–625 CrossRef CAS.
- Y. Minami, K. Kawabata, Y. Kubo, S. Arase, K. Hirasaka, T. Nikawa, N. Bando, Y. Kawai and J. Terao, Peroxidized cholesterol-induced matrix metalloproteinase-9 activation and its suppression by dietary beta-carotene in photoaging of hairless mouse skin, J. Nutr. Biochem., 2009, 20, 389–398 CrossRef CAS.
- S. Grether-Beck, M. Salahshour-Fard, A. Timmer, H. Brenden, I. Felsner, R. Walli, J. Fullekrug and J. Krutmann, Ceramide and raft signaling are linked with each other in UVA radiation-induced gene expression, Oncogene, 2008, 27, 4768–4778 CrossRef CAS.
- F. El Ghissassi, R. Baan, K. Straif, Y. Grosse, B. Secretan, V. Bouvard, L. Benbrahim-Tallaa, N. Guha, C. Freeman, L. Galichet and V. Cogliano, A review of human carcinogens–part D: radiation, Lancet Oncol., 2009, 10, 751–752 CrossRef.
- A. K. von Thaler, Y. Kamenisch and M. Berneburg, The role of ultraviolet radiation in melanomagenesis, Exp. Dermatol., 2010, 19, 81–88 CrossRef CAS.
- E. Kvam and R. M. Tyrrell, Induction of oxidative DNA base damage in human skin cells by UV and near visible radiation, Carcinogenesis, 1997, 18, 2379–2384 CrossRef CAS.
- M. R. Zaidi, S. Davis, F. P. Noonan, C. Graff-Cherry, T. S. Hawley, R. L. Walker, L. Feigenbaum, E. Fuchs, L. Lyakh, H. A. Young, T. J. Hornyak, H. Arnheiter, G. Trinchieri, P. S. Meltzer, E. C. De Fabo and G. Merlino, Interferon-gamma links ultraviolet radiation to melanomagenesis in mice, Nature, 2011, 469, 548–553 CrossRef CAS.
- A. A. Krasnovsky Jr. and V. E. Kagan, Photosensitization and quenching of singlet oxygen by pigments and lipids of photoreceptor cells of the retina, FEBS Lett., 1979, 108, 152–154 CrossRef CAS.
- C. M. Krishna, S. Uppuluri, P. Riesz, J. S. Zigler Jr. and D. Balasubramanian, A study of the photodynamic efficiencies of some eye lens constituents, Photochem. Photobiol., 1991, 54, 51–58 CrossRef CAS.
- B. M. Dzhagarov, N. N. Kruk, N. V. Konovalova, A. A. Solodunov and I. I. Stepuro, Quantum yield of photosensitized formation of singet oxygen by vitamines of the B6 group and their adducts with amino acids and proteins, J. Appl. Spectrosc., 1995, 62, 122–127 CrossRef CAS.
- E. Oliveros, F. Besançon, M. Boneva, B. Kräutler and A. Braun, Singlet oxygen (1Δg) sensitization and quenching by vitamin B12 derivatives, J. Photochem. Photobiol., B, 1995, 29, 37–44 CrossRef CAS.
- S. Dad, R. H. Bisby, I. P. Clark and A. W. Parker, Formation of singlet oxygen from solutions of vitamin E, Free Radical Res., 2006, 40, 333–338 CrossRef CAS.
- A. A. Gorman, I. Hamblett and M. A. Rodgers, Ergosterol (provitamin D2) triplet state: an efficient sensitiser of singlet oxygen, O2(1 delta g), formation, Photochem. Photobiol., 1987, 45, 215–221 CrossRef CAS.
- M. Rozanowska, J. Wessels, M. Boulton, J. M. Burke, M. A. Rodgers, T. G. Truscott and T. Sarna, Blue light-induced singlet oxygen generation by retinal lipofuscin in non-polar media, Free Radical Biol. Med., 1998, 24, 1107–1112 CrossRef CAS.
- L. J. Martinez, R. H. Sik and C. F. Chignell, Fluoroquinolone antimicrobials: singlet oxygen, superoxide and phototoxicity, Photochem. Photobiol., 1998, 67, 399–403 CAS.
- T. Arai, Y. Nishimura, M. Sasaki, H. Fujita, I. Matsuo, H. Sakuragi and K. Tokumaru, Mechanism of production of singlet oxygen on photoexcitation of drugs inducing photosensitivity, Bull. Chem. Soc. Jpn., 1991, 64, 2169–2173 CrossRef CAS.
- B. Ehrenberg, J. L. Anderson and C. S. Foote, Kinetics and yield of singlet oxygen photosensitized by hypericin in organic and biological media, Photochem. Photobiol., 1998, 68, 135–140 CrossRef CAS.
- K. A. Schenkman, Cardiac performance as a function of intracellular oxygen tension in buffer-perfused hearts, Am. J. Physiol. Heart Circ. Physiol., 2001, 281, H2463–2472 CAS.
Footnote |
† Contribution to the themed issue on the biology of UVA. |
|
This journal is © The Royal Society of Chemistry and Owner Societies 2012 |