DOI:
10.1039/C1NR11271F
(Paper)
Nanoscale, 2012,
4, 239-250
Curcumin loaded chitin nanogels for skin cancer treatment via the transdermal route
Received
9th September 2011
, Accepted 8th October 2011
First published on 14th November 2011
Abstract
In this study, curcumin loaded chitin nanogels (CCNGs) were developed using biocompatible and biodegradable chitin with an anticancer curcumin drug. Chitin, as well as curcumin, is insoluble in water. However, the developed CCNGs form a very good and stable dispersion in water. The CCNGs were analyzed by DLS, SEM and FTIR and showed spherical particles in a size range of 70–80 nm. The CCNGs showed higher release at acidic pH compared to neutral pH. The cytotoxicity of the nanogels were analyzed on human dermal fibroblast cells (HDF) and A375 (human melanoma) cell lines and the results show that CCNGs have specific toxicity on melanoma in a concentration range of 0.1–1.0 mg mL−1, but less toxicity towards HDF cells. The confocal analysis confirmed the uptake of CCNGs by A375. The apoptotic effect of CCNGs was analyzed by a flow-cytometric assay and the results indicate that CCNGs at the higher concentration of the cytotoxic range showed comparable apoptosis as the control curcumin, in which there was negligible apoptosis induced by the control chitin nanogels. The CCNGs showed a 4-fold increase in steady state transdermal flux of curcumin as compared to that of control curcumin solution. The histopathology studies of the porcine skin samples treated with the prepared materials showed loosening of the horny layer of the epidermis, facilitating penetration with no observed signs of inflammation. These results suggest that the formulated CCNGs offer specific advantage for the treatment of melanoma, the most common and serious type of skin cancer, by effective transdermal penetration.
1. Introduction
Drug delivery through the transdermal route is one of the most important modes of drug administration when the oral route fails due to many reasons. Being a non-invasive route, it has advantages over injections. It is well known that skin impart barrier functions and does not easily permit the entry of the majority of drugs. The barrier property of the skin as part of its protective function is mainly due the stratum corneum, also known as horny layer. The stratum corneum consists of separated, non-viable, cornified, almost nonpermeable corneocytes embedded into a continuous lipid bilayer made of various classes of lipids. The stratum corneum is crucial for the barrier function of the skin, controlling percutaneous absorption affecting dermal and transdermal drug delivery. For any substance to penetrate the skin, the ideal physicochemical parameter limits for passive transdermal delivery of substances and that of curcumin are shown in Table 1.1
Table 1 Physico-chemical parameter limits for passive transdermal delivery
Critical properties for transdermal delivery |
Requisite for passive skin penetration |
Properties of curcumin |
Aqueous solubility |
>1 mg ml−1 |
Insoluble |
Lipophilicity |
10 < Ko/w < 1000 |
1950 |
Molecular weight |
<500 Daltons |
368.39 |
Melting point |
<200 °C |
183 °C |
pH of saturated aqueous solution |
5–9 |
∼6 |
Based on the properties of the substance, it may be necessary to use some technique to enhance the penetration in order to achieve effective transdermal delivery. Such methods include the use of chemical penetration enhancers, the use of physical methods like sonophoresis or the use of colloidal carriers like lipid vesicles or solid lipid nanoparticles. Nanogels are basically nanosized versions of their adult counterparts, the hydrogels. They have many advantages as do other nanovehicles, but in addition to these other properties like their high stability in aqueous media2 as well as biological fluid,3 high water uptake,4 and rapid response to external stimuli make them attractive candidates for drug delivery. Stimuli-responsive micro and nanogels offer unique advantages as drug delivery carriers including tunable size from the submicron range to tens of nanometres, a large surface area for bioconjugation, a porous structure to store drugs and the controllable release of a drug at a specific chemical and biological environment.5–8 Natural biopolymers have been widely used to prepare responsive hydrogels for biomedical applications due to their biocompatibility, low toxicity and their high content of functional groups.9
Chitin is a very versatile material for drug loading due to its capacity to form polyelectrolyte complexes and high functionality due to its large number of –OH and –NHCOCH3groups. Moreover it is the second-most-abundant natural biodegradable polymer, easily available and economic. Chitin is well known as an “organo template” for calcium and silica bio-mineralization as well as drug molecules.10–13 When compared to chitosan, chitin is less extensively exploited in biomedical fields due to its innate water insolubility. A chitin-based nanogel can be an ideal candidate for skin penetration for many reasons. As the permeability of a substance through skin is inversely proportional to its size under certain conditions,14 the nanoscale size of curcumin loaded chitin nanogels (CCNGs) is one major factor facilitating penetration. The cationic charge of chitin can also facilitate penetration through intact skin. Chitin, as well as curcumin, is lipophilic in nature and highly water insoluble, but the nanogel is hydrophilic; this hydrophilic–lipophilic balance of the formulation is highly beneficial. A good transdermal system, beyond allowing a desirable amount of drug to cross the skin barrier, has to be biocompatible, preferentially biodegradable and non-irritant to skin.15 Chitin is a very good candidate in these aspects as well. Curcumin is extensively studied for its wide variety of pharmacological effects.16,17 However, the most important limitation reported is the low bio-distribution and bioavailability, thus hindering the possible effectiveness against challenging diseases like cancer. The chitin nanogel was prepared and reported by our group18,19 in our previous studies and was found to be useful for various applications.
Skin cancer is one of the most common types of cancer with an increasing number of cases worldwide. There are three main types of skin cancer: (1) basal cell carcinoma; (2) squamous cell carcinoma; and (3) melanoma, affecting melanocytes. Cutaneous malignant melanoma is the most lethal form of skin cancer and it is one of the most treatment-refractory malignancies. The current treatment modality for metastatic melanoma is poor in terms of response and survival rate. Although some biochemotherapy trials have shown good outcomes, no agent or regimen has improved median response duration and overall survival in subsequent studies. New treatment options by using natural phytocompounds or herbal medicines either as such or in combination with some chemotherapeutic agents may provide a breakthrough in the treatment of malignant melanoma.20 This study focuses on the use of curcumin, a phytodrug well known for its multiple mechanism of action against cancer malignancies,21 for the treatment of melanoma through an effective transdermal delivery.
2. Materials and methods
2.1. Materials
Chitin (degree of acetylation–72.4%; molecular weight 150 kDa) was purchased from Koyo chemical Co., Ltd, Japan. Calcium chloride and methanol were purchased from Qualigens, India. Curcumin (C21H20O6) (derived from the rhizome of Curcuma longa), a member of the ginger family Zingiberaceae, with IUPAC name (1E,6E)-1,7-bis (4-hydroxy-3-methoxyphenyl)-1,6-heptadiene-3,5-dione and common name diferuloyl methane, was purchased from Merck, used as received. Human melanoma cell lines (A375) were received from the National Center for Cell Sciences, Pune, India. Human dermal fibroblast (HDF) cells were obtained from Procell, Germany. The chemicals were used without further purification.
2.2. Preparation of control chitin nanogels
Chitin nanogels were prepared by our reported method.19,20 The chitin solution was also prepared according to a reported method.22 5 g of chitin was added to 1 L of a saturated CaCl2 solution in methanol and stirred vigorously for 48 h at room temperature. The resulting transparent solution was filtered to remove traces of undissolved material. The obtained chitin solution was then stirred on a magnetic stirrer for 1 h to ensure complete uniformity. Methanol was then added in a drop-wise manner to the solution, while stirring. The chitin was regenerated on the addition of methanol and the solution turned turbid. This was then washed several times by centrifuging and resuspending the pellet in water. Every time the pellet was resuspended, it was subjected to a vigorous sonication, breaking the particles down. These repeated steps of centrifugation and sonication brought down the particles size to the nano-regime. The particles, devoid of any excess methanol and calcium chloride, were then resuspended in water for further study.
2.3. Preparation of curcumin loaded chitin nanogels (CCNGs)
5 mg of curcumin was dissolved in 5 mL of methanol, which was added drop-wise to 5 ml of chitin solution with constant stirring. This gives CCNGs with a 20% curcumin loading. The chitin solution was stirred for an hour before the addition of curcumin, in order to facilitate a uniform interaction since the chitin solution was very viscous in nature. When the chitin with entrapped curcumin is regenerated, the solution turned to a turbid yellow. This mixture was centrifuged and the yellow colored pellet was resuspended in 10 mL of water with the help of ultrasonication. Repeated centrifugation and resuspension of the pellet in water removes the methanol. Probe sonication was done with a Vibracell sonicator with working power of 130 W, frequency 20 KHz and 230 V of voltage. The result is the breakdown of the macrogels to nanogels loaded with the drug. The dispersion of the washed pellet in water gave a uniform yellow suspension.
2.4. Tagging fluorescent Rhodamine-123 dye with CCNGs (Rhod-CCNGs)
Since a laser for curcumin excitation (420 nm) was not available with our instrument, the fluorescent dye Rhodamine-123 (excitation 511nm & emission 534 nm) was tagged with the prepared CCNGs. This was done by the addition of 40 μL solution of 1 mg mL−1 concentration of Rhodamine-123 to 5 mL of CCNGs dispersion under magnetic stirring. This was then probe sonicated for 5 min. and continued magnetic stirring for another 2 h and further centrifuged for 30 min at 20
000 rpm to remove the unbound Rhodamine-123.
2.5
Curcumin loading efficiency (LE) of chitin nanogels
The LE of chitin nanogels was calculated during the preparation of the drug-loaded samples. The supernatant collected after every centrifugation step was combined and the amount of curcumin present is quantified by an HPLC.23 The concentration of curcumin in the samples was calculated against known standards via the method of area under the absorption time curves.
The LE is calculated using the formula given below.
2.6 Characterizations
FTIR spectrum data was obtained for all of the samples including control chitin, chitin nanogel, the CCNGs and the control curcumin as well; using a Perkin Elmer Spectrum RX1 Fourier transform infrared spectrophotometer by KBr tablets (1% w/w of product in KBr) with a resolution of 4 cm−1 and 100 scans per sample. The thermal studies were conducted using S II TG/DTA 6200 EXSTAR. The mean size and size distribution as well as the zeta potential of the prepared nanogels were determined by dynamic light scattering (DLS-ZP/Particle Sizer NicompTM 380 ZLS) measurements. The size of the particles was further confirmed using SEM (JEOLJSM-6490LA).
2.7 Swelling studies
The swelling behavior of the control chitin nanogels as well as CCNGs were studied at different pH conditions since the formulation was a nano hydrogel, which changes the physical properties with respect to pH. The swelling was studied at 3 different pHs as acidic, neutral and basic (pH 4, 7 and 9 respectively). The frozen chitin nanogel and CCNGs were pelletized using a hydraulic pelletiser. The dry weight of the pellet was noted (Wo). The pellet was then placed in a corresponding pH solution for 5 min, then removed from the solution and the surface adsorbed solution was removed using filter paper and wet weight was recorded (Ww). Three different pellets were used for the study. The swelling ratio was calculated by the following formula.
Swelling ratio = Ww − Wo/Wo |
The swelling at each pH was studied in triplicate.
2.8
Drug release studies
The in vitrodrug release study was carried out with 20% drug loaded formulations. Pellets of the prepared drug loaded formulations were dispersed in 10 mL PBS of 2 different pH values, 7.4 and 4.5 with temperature kept at 37 °C and placed in an incubator shaker at 50 rpm. At predetermined intervals, aliquots of 500 μl were withdrawn and replaced with an equal volume of fresh buffer. These samples were added with 500 μL ethanol, mixed well and centrifuged at 10
000 rpm for 15 min to separate the polymeric nanoparticles. The supernatant was filtered using a 22 μL syringe filter and 20 μL of the solution was injected into the HPLC system (LC 2010A HT SHIMADZU). A 75
:
25 mixture of acetonitrile–5% acetic acid was used as the mobile phase and a C18 column (5 μm, 3 × 150 mm) was used. At a flow rate of 1mL min−1, the retention time of curcumin was 1 ± 0.2 min. The detector was set at 429 nm. The concentration of curcumin in the samples was calculated against known standards via the method of area under the absorption time curves.
2.9
In vitro hemolysis assay
Blood compatibility of the formulation was evaluated by hemolysis assay using fresh human blood. 1.5 mL acid citrate dextrose (ACD) was added to 10 mL fresh blood. 100 μL samples of concentrations ranging from 0.1 to 10 mg mL−1 were added to a 1 mL of blood sample and incubated for 2 h with shaking in an incubator chamber at 37 °C. The samples were centrifuged at 4500 rpm for 10 min to obtain the plasma (The nature of the plasma gives the indication of hemolysis; it would be red in color if hemolysis occurs). The plasma was collected and 100 μL of the plasma was mixed with 1 μL of 0.01% Na2CO3. The OD values were read at 450, 380 and 415 nm. The plasma hemoglobin can be calculated using the following equation.
Plasma Hb = {(2A415) − [A380 + A450] × 76.25} |
The values obtained for samples were compared with that of normal saline as a negative control and Triton as a positive control. Each concentration was evaluated in triplicate.
2.10
Cell culture
Human dermal fibroblast (HDF) cells (Procell Germany) were maintained in Minimum Essential Medium (MEM) and A375 (Human melanoma cell lines, NCCS Pune) were maintained in Dulbecco's modified Eagles Medium (DMEM) supplemented with 10% fetal bovine serum (FBS). The cells were incubated in CO2 incubator with 5% CO2. After reaching confluency, the cells were detached from the flask with Trypsin-EDTA. The cell suspension was centrifuged at 3000 rpm for 3 min and then re-suspended in the growth medium for further study.
2.10.1 Confocal cell uptake studies.
For confocal cell uptake studies, Rhod-CCNGs were used. Acid etched cover slips kept in 24 well plates were loaded with A375 and HDF cells, respectively, with a seeding density of 20
000 cells per cover slip and incubated for 24 h for the cells to attach to the well. After 24 h incubation, the medium was removed and the wells were carefully washed with PBS buffer. Then the particles, at a concentration of 1 mg ml−1, were added along with the media in triplicate to the wells and incubated for a time period of 6 h. After each particular incubation time, media with sample were removed and the cover slips were processed for confocal microscopy. The processing involved washing the cover slips with PBS and fixing the cells in 3.7% paraformaldehyde (PFA) followed by a final PBS wash. The cover slips were air dried and mounted on to glass slides using DPX. The slides were then viewed under the confocal microscope (Leica SP 5 II) to study the internalization of CCNGs by the cells.
2.10.2. Cytotoxicity studies.
Cytotoxicity experiments were carried out on HDF and A375 cell lines by MTT assay. MTT [3-(4,5-dimethylthiazole-2-yl)-2,5-diphenyl tetrazolium] assay for cytotoxic evaluation is a colorimetric test based on the selective ability of viable cells to reduce the tetrazolium component of MTT to purple-colored formazan crystals. The cells were seeded on a 96 well plate with a density of 10
000 cells cm−2. Four different concentrations of the CCNGs (0.2, 0.4, 0.8 and 1 mg mL−1 where the curcumin concentration varied from 5 to 20 μg) were prepared by dilution with the medium. Control chitin nanogel and control curcumin solution were also prepared in the same concentration range. After reaching 90% confluency, the cells were washed with PBS buffer and different concentrations of the CCNGs (100 μL) were added and incubated. Cells in medium alone, devoid of nanogels, acted as a negative control and cells treated with Triton X-100 acted as a positive control for a period of 24 h. 5 mg of MTT (Sigma) was dissolved in 1 mL of PBS and filter sterilized. 10 μL of the MTT solution was further diluted to 100 μL with 90 μL of serum-free phenol-red-free medium. The cells were incubated with 100 μL of the above solution for 4 h to form formazan crystals by mitochondrial dehydrogenases. 100 μL of the solubilisation buffer (10% Triton X-100, 0.1 N HCl and isoproyl alcohol) was added in each well and incubated at room temperature for 1 h to dissolve the formazan crystals. The optical density of the solution was measured at a wavelength of 570 nm using a Beckmann Coulter Elisa plate reader (BioTek Power Wave XS). Triplicate samples were analyzed for each experiment.
2.10.3.
FACS analysis–apoptosis assay by flow cytometry.
Annexin V-FITC/PI staining.
Phosphatidylserine (PS) translocation from the inner to the outer layer of plasma membrane is one of the important earliest apoptotic features. The PS exposure in A375 cells was detected using an Annexin V-FITC/PI Vybrant apoptosis assay kit (Molecular probes, Eugene, OR). After reaching 90% confluency, the cells were treated with different concentrations of CCNGs, control chitin nanogels and control curcumin solution as described previously. After being exposed to samples for 24 h at 37 °C, cells were harvested by trypsinization and washed with PBS for 5 min followed by centrifugation at 500 g at 4 °C. The supernatant was discarded and the pellet resuspended in ice-cold 1X Annexin binding buffer (5 × 105–5 × 106cells mL−1). 2 μL of Annexin V-FITC solution and 0.5 μL of PI (100 μg mL−1) were added to 100 μL of the cell suspension and mixed gently. The samples were then incubated at room temperature for 15 min in the dark. After incubation, 400 μL of ice-cold 1X binding buffer was added, mixed gently, and analyzed by flow cytometry (FACS Aria II (Beckton and Dickinson, Sanjose, CA). Cells in media alone devoid of nanogels (negative control) and cells treated with control nanogels were also analyzed in the same way. Samples were analyzed in triplicate for each experiment.
2.11.
Ex
vivo skin permeation studies
Most evaluations in the area of skin permeation research are carried out using a large variety of set ups and experimental protocols. Also, various types of membranes are used for such experiments.24 In the case of animal skin, human skin finds its closest match in porcine tissue, comparing the results of several species.25–27 A permeation experiment was carried out using full thickness porcine skin in a vertical Franz diffusion (FD) cell (Fig.9A).
2.11.1 Porcine skin samples.
Fresh pig ear was collected from a local slaughterhouse. After thorough washing, the hair on the skin was removed using a razor and the skin was separated from the underlying cartilage and subcutaneous fat using a scalpel and cut into appropriate sizes, wrapped in aluminium foil and stored at −20 °C. The skin samples were stored for a maximum of 1 month only.
2.11.2 Permeation experiments.
For the experiment, the skin cut into proper size was sandwiched between the donor and acceptor compartment of the FD cell. 1 mL of the sample under test (the CCNGs dispersion as well as control curcumin solution (containing 500 μg of curcumin) was added in the donor compartment on the stratum corneum side of the skin and phosphate buffer pH 4.5 was taken in the acceptor compartment. The temperature was kept at 32 ± 1 °C by a circulating water jacket. The acceptor fluid was gently stirred with a magnetic stirrer. The preconditioning of the skin was done before each experiment by hydrating the skin with the phosphate buffer for 30 min. 0.5 mL of the acceptor fluid was taken at 1 h intervals from 0 to 5 h and then at 24 h and replaced with an equal quantity of fresh buffer. The amount of curcumin penetrated was quantified by HPLC analysis.
2.11.3
HPLC procedure.
The amount of curcumin penetrating through the skin into the acceptor fluid was quantified by an HPLC method.23 The sample collected from the acceptor fluid in the FD cell was added with equal volume of distilled ethanol and mixed well to dissolve the curcumin present in it. This was then centrifuged at 5000 rpm for 30 min and the supernatant was filtered using a 22 μm syringe filter. 20 μL of this solution was injected into the HPLC system under the conditions mentioned earlier to determine the curcumin concentration.
2.11.4
Drug concentration–depth profiles.
The skin samples from the above experiments in triplicate were collected after the exposure period. The skin was washed with PBS and dried with an absorbent towel and samples of 1 cm2 area were cut and placed on a cork disc. The freezing liquid was added on the skin sample and allowed to freeze at −20 °C. The frozen samples were sectioned using a cryotome (Leica CM 1505 S) into 50 μm sections.28 The cryosections were grouped into three groups as upper, middle and lower sections in three different containers and curcumin was extracted by adding 3 mL of ethanol and incubating for 24 h. These were then centrifuged at 5000 rpm for 30 min and the supernatant filtered using 22 μm syringe filter. The HPLC analysis for curcumin was then carried out as mentioned above.
2.11.5 Data analysis.
Permeation parameters were obtained from the cumulative amounts of curcumin permeated per cm2versus incubation time plots. The steady state flux (J) – representing the absorption rate per unit area – was determined from the slope of the linear portion of the plots.9 The permeability constant was calculated according to Fick's first law of diffusion.29–31 Based on the steady state flux and the applied drug concentration (C1) of the donor compartment (eqn (1)).
The enhancement ratio (Er) was calculated from the steady state flux values as shown below (eqn (2)).32
| Er = J of formulation/J of control | (2) |
2.11.6 Fluorescent imaging.
As curcumin has an innate fluorescent property, its localization at different depths of skin can be observed by fluorescent imaging. The skin samples from the permeation experiments were collected after the exposure period. Skin samples treated with PBS were used as a control. The skin samples were washed with PBS and dried with an absorbent towel and samples of 1 cm2 areas were cut and placed on a cork disc. The freezing liquid was added to the skin sample and allowed to freeze at −20 °C. The frozen samples were sectioned using a cryotome (Leica CM 1505 S) into 5 μm sections.28 One section from each control group as well as sample-treated ones were repeatedly treated with xylene to remove water and mounted on to glass slides for fluorescent imaging using DPX. The slides were then viewed under the fluorescent microscope (Olympus-BX-51) to understand the localization of permeated curcumin at different depth of dermal penetration.
2.11.7. Histological examinations.
Porcine skin samples were treated with PBS (control), control curcumin solution, control chitin nanogels and CCNGs respectively in FD cell exactly as in permeation experiments for an exposure period of 10 h. Then, the skin samples were washed properly with PBS and fixed in 10% formaldehyde in PBS. Samples were then processed in a tissue processor to remove formaldehyde, embedded in paraffin for fixing and vertical sections were taken using a microtome. The sections after removing traces of paraffin were stained with hematoxylin and eosin (H & E). The paraffin embedding and sectioning of skin samples was done by a tissue embedding center and a semi automatic microtome (Microtomes India).
2.12. Statistics
Statistical analysis of the data was performed using Students T-test analysis. A value of p < 0.05 was considered significant. (n = 3).
3. Results and discussion
3.1 Preparation of control and curcumin loaded chitin nanogels
The preparation of CCNGs basically involves regeneration of chitin along with entrapped curcumin and further wet milling to reduce the size to the nanoscale by probe sonication. The term regeneration here implies the restoration of chitin as well as curcumin from their solutions but then as a conjugate not as individual components. The interesting factor here is that the regeneration is brought about by addition of excess methanol, which itself in the presence of calcium chloride is solvent for the chitin. Here the important parameters involved are the time and amplitude of probing which bring about the regeneration as nanogels. The interplay of interactions, such as hydrophobic association of major functionalities also contributes towards the stability of the nanogels. The plausible interaction chemistry between curcumin with chitin nanogels has been shown in Fig. 1. The hydrogen bonding interaction between curcumin with chitin nanogels could be via the end–end mode. The –OH and –NHCOCH3 functionalities in chitin nanogels are more prone to hydrogen bonding with curcumin as they have –OH as their terminal groups.
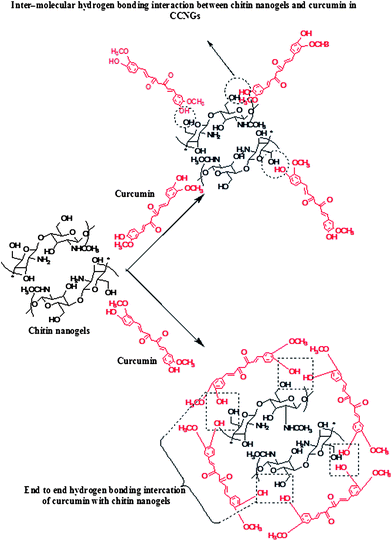 |
| Fig. 1 Representation for the plausible interaction mechanism between curcumin and chitin nanogels in CCNGs. | |
3.2 Size and morphology of the nanogels
Fig. 2A showing control chitin nanogel and 2B the stable CCNGs dispersion in water. Fig. 2C & 2D shows the SEM image and DLS spectra of CCNGs, respectively. SEM analysis confirms that CCNGs are nearly spherical in shape and the size range is around 70–80 nm (c1 shows the magnified image for the CCNGs), which facilitates skin penetration since permeability of a substance through skin is inversely proportional to its size under certain conditions.10 It was found that as the amplitude and time of probing increases the particle size decreases (Table 2). The zeta potential value shown in Table 3 indicates that the system forms a dispersion with good stability, as indicated by the value higher than 40, and the positive value is largely due to the positive charge of chitin. The entrapment efficiency of curcumin in CCNGs was found to be 95%.
Table 2 Showing the particle size parameters for the CCNGs with respect to time and amplitude of probe sonication
Samples |
Time/min |
Amplitude (%) |
Particle size measured in DLS/nm |
CCNGs (a) |
1 |
25 |
200 ± 2 |
CCNGs (b) |
2 |
45 |
150 ± 7 |
CCNGs (c) |
3 |
60 |
100 ± 5 |
CCNGs (c) |
5 |
75 |
60 ± 15 |
Table 3 Zeta potential of control chitin nanogels and CCNGs
Samples |
Zeta potential in water at pH 5/mV |
CCNGs |
+ 49.34 |
Control chitin nanogels |
+ 44.25 |
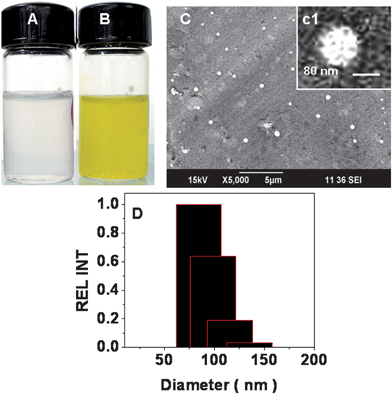 |
| Fig. 2 A & B: The stable control chitin nanogel and CCNGs at pH 5.0. C, c1 & D: the size distribution of CCNGs by SEM and DLS. | |
3.3
FTIR analysis of CCNGs
Fig. 3A shows the FTIR spectrum of CCNGs, control nanogels, chitin (control) and the control curcumin. In the spectrum of control nanogels, characteristic bands were observed at 1640 (amide I region) and 1070 cm−1 (–C–O– stretching). The IR spectrum of curcumin exhibited characteristic absorption bands at 2954, 1430 and 1376 cm−1, attributed to the C–H stretching modes and deformation of methyl groups.33 Additionally, sharp absorption bands at 1627 (enol carbonyl stretching vibration), 964 (plane bending of aromatic C–H bond), 1155 (C–O stretching), 1605 (stretching vibrations of benzene ring), and 1435 cm−1 (olefinic C–H bending vibrations).34,35 were also observed. The complexation of curcumin with chitin caused a shift in the characteristic peaks of both the control nanogels and curcumin. Comparing the control drug with the CCNGs, a peak shift was observed from 1627 to 1637 cm−1, 964 to 952 cm−1 and a small shift from 1155 to 1156 cm−1. These peak shifts can be attributed to the intermolecular hydrogen bonding between the curcumin and chitin. Also the curcumin peak at 1376 cm−1 appears in the CCNGs confirming the association of chitin and curcumin. On comparing the CCNGs and control chitin nanogels a shift was seen in the peak at 1070 to 1075 cm−1. The data obtained confirms the association between the curcumin and chitin in CCNGs.
3.4. Thermal analysis
The thermal stability and thermal decomposition of prepared systems were investigated by TG and are given in Fig. 3B. It shows that the initial degradation temperature of the control chitin nanogel is very close to 100 °C; a slow weight loss starting from 70 to 100 °C due to the decomposition of polymer with low molecular weight is followed by more obvious loss of weight starting from 250 to 350 °C, which could be attributed to a complex process including dehydration of the saccharine rings.19 The degradation profile of CCNGs is not similar to the control chitin nanogel, but more stable which proves that the system has an amorphous nature.
3.5 Swelling studies
Fig. 4A shows the swelling ratio for control chitin nanogels and CCNGs, calculated at three different pHs. The swelling was highest at acidic pH compared to neutral, acidic and alkaline conditions. This is because a polyelectrolyte nanogel, like the chitin nanogel, is believed to swell at a pH below its pKa of 6.135–37 and fixed charges are developed on the nanogel network when it is exposed to an acidic pH. This swelling forms the basis of the pH responsivity of the nanogel. The swelling ratios of the control nanogels were found to be greater than the CCNGs at corresponding pH values. This is due to the fact that the drug conjugation reduces the number of free reactive functional groups present on the chitin nanogels.
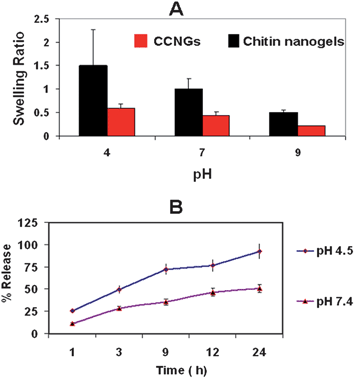 |
| Fig. 4 A: Swelling ratios of CCNGs in comparison to control CNG. B: Curcumin release from the nanogels at different pH. | |
3.6
Curcumin release studies from CCNGs
The drug release studies were carried out at acidic and neutral pH. The release profile is shown in Fig. 4B. It is evident from the Figure that the drug release is more prominent at acidic pH than at neutral pH. This again shows the pH-responsive behavior of the nanogels. The enhanced release is due to the swelling of CCNGs in acidic pH, as seen in swelling studies, due to the protonation of the free amine groups. The release of curcumin from CCNGS is actually a combination of simple diffusion, nanogel degradation, change in pH and the displacement by counterions present in the environment.38 This is because the ionization of the pendant groups causes electrostatic repulsion between the like charges in the nanogel network, thereby resulting in the enlargement of the pores and allowing excess solvent influx leading to release of the encapsulated payload.3 From the release data, it is seen that almost 80% of the drug is released within 24 h when the nanogel is exposed to pH 4.5, allowing a better release in the acidic tumor environment. Only less than 30% of curcumin is released at pH 7.4 for the same time interval.
The observation of the higher release at acidic pH is significant because of two reasons. One is that curcumin under in vitro conditions shows rapid degradation at and above neutral pH39 and secondly the pH at the tumor site is acidic, which favors site-specific release of the drug.
3.7
In vitro hemolysis assay of the nanogels
Blood compatibility is an important measure of biological safety for biomaterials. Here, the material might be exposed to blood circulation when systemic delivery is achieved through transdermal route. Hemolysis assay was carried out as an indicator of blood compatibility analysis of nanogels. The results in Fig. 7D shows that there is negligible hemolysis produced by sample at different concentrations compared to the positive control. The hemolytic ratio of the sample was found to be 2.45% which is far below 5%, the critical safe hemolytic ratio for bio-materials according to ISO/TR 7406. This indicates that the risk of hemolysis by CCNGs as well as control chitin nanogels is negligible.
3.8 Cytotoxicity studies
The MTT assay showed that CCNGs has specific toxicity towards A375 compared to its low toxicity towards human dermal fibroblast cells as shown in Fig. 5A and 5B, respectively. There are many reasons suggested for the selective toxicity of curcumin towards cancer cells and not towards normal cells. One major reason is the lower glutathione levels in tumor cells compared to normal cells, thus enhancing the sensitivity of tumor cells to curcumin.39 Secondly, most tumor cells and not normal cells express constitutively active NF-KB and mediate their survival.40Curcumin can suppress the survival and proliferation of tumor cells by suppressing NF-KB regulated gene products. However, few other studies showed that curcumin induces apoptosis in primary cultures of human dermal fibroblasts at higher concentrations and this could be reduced by coadministration of some antioxidants41,42 Chitin and its derivatives are reported to have an antioxidant effect43 and this may be the reason why conjugation of curcumin with chitin in CCNGs reduced the toxic effect on fibroblast cells. Our group has already reported the non-toxicity of control chitin nanogels on a number of normal cells.35 The MTT results throws light on the fact that CCNGs also retain the specificity of curcumin towards cancer cells without affecting the normal cells, which is a major requisite for the cancer treatment modality.
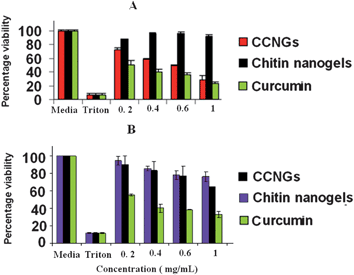 |
| Fig. 5 The results of MTT assay on A375 (A) and human dermal fibroblast cells (B). | |
3.9
Cell uptake studies
Fig. 6A shows the internalization of CCNGs by A375 cells after an incubation time of 6 h as observed by confocal microscopy. It was clear from the images that the fluorescence intensity increases as we go deeper into the cell indicating the internalization of particles well within the cell. Fig. 6B shows the same with human dermal fibroblast cells and as observed in the images these cells also show internalization but to a lesser extent. The images, as shown in Fig. 6A and B showed a distinguishable difference in fluorescence intensity, which correlates to the specific uptake of curcumin by the cancer cells as already reported by the scientific community and due to reasons mentioned above.
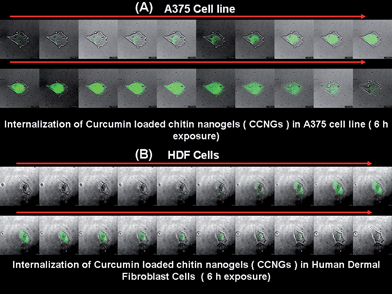 |
| Fig. 6 A & B: Cellular localization of CCNGs on A375 and human dermal firoblast cells, respectively, after a 6 h incubation period by confocal microscopy. | |
3.10.
Apoptosis assay by flowcytometry
Annexin V-FITC/PI staining.
Fig. 7A–C shows apoptotic profile of control curcumin solution, control chitin nanogels, and CCNGs. It is evident from the Figure that there is no significant apoptosis induced by the control chitin nanogels whereas the CCNGs at the higher concentration of the cytotoxic range showed comparable apoptosis to that of control curcumin. It shows that even after nanogel loading and conjugation with chitin, the cytotoxic efficacy of the drug was retained.
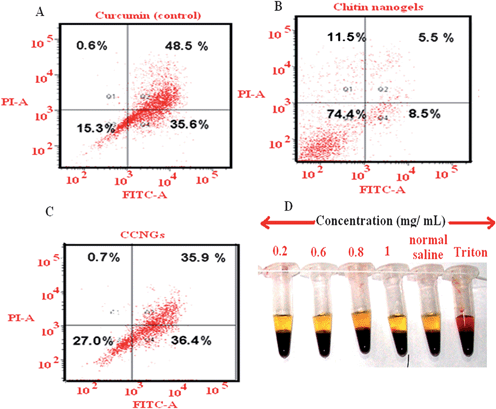 |
| Fig. 7 A, B & C: FACS based apoptosis analysis for the control curcumin, chitin nanogel and CCNGs on A375 cells after 24 h exposure; 7D: In vitro hemolysis studies for the control chitin nanogels and CCNGs at different concentrations. | |
It is a well known fact that curcumin induce apoptosis of cancer cells by different mechanisms. Curcumin affects both the main apoptotic pathways: the intrinsic (mitochondrial) pathway and the extrinsic (death receptor) pathway. It induces apoptosis in tumor cells at the G2 phasevia upregulation of p53 expression and initiation of the mitochondrial apoptotic pathway via increased Bax expression and cytochrome c release.44–48Curcumin also has a stimulatory effect on the extrinsic apoptotic pathway, which is triggered by the binding of “death activators” such as TNF-α, and Fas ligand to their corresponding cell surface receptors. Activation of these receptors results in activation of caspase-8via the receptor-attached FADD adapter molecule and initiation of the Caspase cascade.49,50 A study on melanoma demonstrated that curcumin led to apoptosis by promoting aggregation of Fas receptors and by increasing levels of caspase-8 and -3 without altering the level of caspase-9 (unique to the intrinsic pathway).51 In addition, upregulation of p53 activation of BID and subsequent down regulation of Bcl-2 are also specific for melanoma. The possible mechanism of action of CCNGs against melanoma could be as shown below in Fig. 8, where the cationic charged CCNGs are able to permeate through the stratum corneum and attach to the negatively charged melanoma cells,52–54 eventually leading to their uptake viaendocytosis and to apoptosis, which could be due to the mechanism44–50 depicted in Fig. 8.
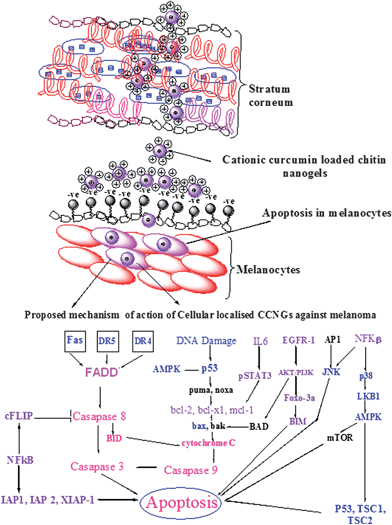 |
| Fig. 8 Proposed mechanism for the modulation of various cell death pathways by CCNGs. Permeation of CCNGs through the stratum corneum and interaction with melanocytes are illustrated in the Figure. Targets which could be up-regulated by CCNGs are in blue letters; those which could be down regulated are in violet letters; the signaling cascade is in pink letters; and those which could be unaffected are in black letters. AP-1 activator protein-1, AMPK 5′ adenosine monophosphateactivated protein kinase, BID BH3 interacting domain death agonist, BIM BCL2-like 11 (apoptosis facilitator), cFLIP cellular FLICE-like inhibitory protein, FADD Fas-associated protein with Death Domain, DR4 death receptor 4, DR5 death receptor 5, EGFR epithelial growth factor receptor, IAP inhibitor of apoptosis protein, IL-6 interleukin-6, JNK c-Jun N-terminal kinase, mTOR mammalian target of rapamycin, NF-kB nuclear factor-kB, PI3K phosphoinositide 3-kinase, STAT3 signal transducer and activator of transcription 3, XIAP X-linked IAP. | |
3.11.
Ex
vivo skin permeation studies
Ex-vivo skin permeation was studied by using vertical Franz diffusion cell shown in Fig. 9A. Fig. 9B shows that the skin penetration of curcumin from CCNGs is more than that from control curcumin solution. The penetration from the control curcumin solution was also influenced by the small amount of ethanol used as a co-solvent and not that of the pure drug as such, which is completely insoluble in water. The flux determined from Fig. 9B was found to be 4 fold greater for CCNGs compared to the control curcumin solution (Table 4), which means the enhancement ratio (Er) for CCNGs was 4. The nanosize as well as the influence of chitin are the major factors contributing to better penetration. The cationic charges, as well as the hydrophobicity of the polymer, are two important parameters affecting skin penetration.52 The more cationic the polymer is, the greater will be the interaction with the negatively charged skin lipids and thus the systemic delivery via this route is found to be greater in the case of cationically charged polymers. Similarly, the hydrophobic moieties on the chitin nanogels could favor van der Vaals interactions with the hydrophobic areas of the keratin,53 and this is an advantage since melanomas may contain keratin.54 There are four accessible routes for transdermal penetration: namely trancellular; intercellular; through the hair follicles; and via the sweat glands. The first two are through the intact horny layer whereas the other two by-pass the horny layer and so are known as shunt pathways. Among these four, the potential pathways are transcellular and intercellular, and the principal pathway taken by a penetrant depends largely on its partition coefficient. Anyway the tortuous intercellular pathway is considered as the major pathway as well as the major barrier for transdermal permeation. Even though the route for CCNGs is not understood, it is believed to pass through both the intercellular and transcellular pathways because of the above mentioned properties of the polymer (Fig. 8) we have used. Fig. 10 shows the fluorescent images of skin samples at different depth and it is clear from the images as well as the concentration depth profile shown in Fig. 9C that the retention of curcumin at the deeper layers of the epidermis and dermis is greater in the case of CCNGs as compared to the control curcumin solution. This also can be explained by the cationic nature of chitin35 facilitating interaction with skin lipids. An effective percutaneous drug delivery should be able to transport the drug across the entire thickness of skin rather than retention at any depth of skin, but here, this increased retention by deep skin layers may be useful to treat diseases like skin cancers.
Table 4 Permeability data (mean ± S.D, n = 3.) calculated for control chitin nanogels and CCNGs (concentration taken is 5 × 105 ng ml−1)
Samples |
Steady state flux, J/ng cm−2 h−1 |
Permeability constant, P/cm h−1 × 10−3 |
CCNGs |
605.21 ± 1.512 |
1.210 ± 1.512 |
Control curcumin solution |
151.49 ± 0.813 |
0.30298 ± 0.813 |
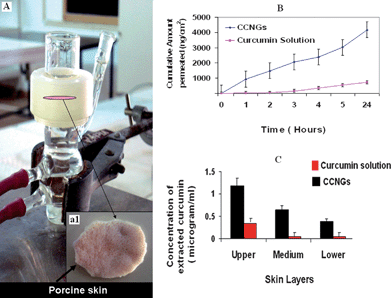 |
| Fig. 9 A: Showing picture of a vertical Franz diffusion cell used for skin permeation studies and inset (a1) porcine skin sample sandwiched between the two compartments; B: plot of cumulative amount of curcumin permeated vs. time for control curcumin and CCNGs; C: Penetration experiments showing concentration – depth profile after 24 h exposure (values reported are mean ± S.D, n = 3). | |
The histopathological evaluations shown in Fig. 11 show the micrographs of porcine skin treated with PBS (control), control chitin nanogel, control curcumin solution and CCNGs. Normal epidermis and dermis morphology was observed in all the samples. In case of control chitin nanogels and CCNGs, the keratin was found to be comparatively thin. and ablation and fragmentation of the stratum corneum was also found.55 These changes were not prominent for the control curcumin solution. Loosening of an otherwise organized multiple lipid bilayer is also visible in the case of skin treated with control nanogels and CCNGs. These are the facilitating factors for better skin penetration of curcumin from CCNGs. No other changes indicating skin irritation or toxicity in terms of edema and erythema were observed in any of the cases.
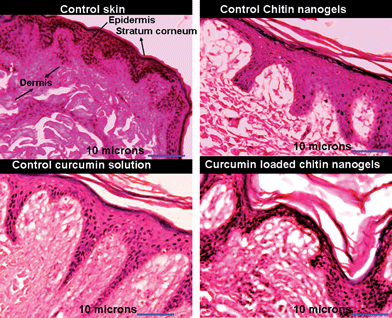 |
| Fig. 11 Images showing results of histopathology studies for control curcumin solution, control chitin nanogels and CCNGs. | |
4. Conclusions
Curcumin loaded chitin nanogels (CCNGs) were developed and evaluated, which can be used for treatment of melanoma through a transdermal route. These CCNGs have desirable size and surface properties, excellent capacity for drug loading and release and good skin penetration and retention properties. These nanogels due to their efficiency of skin penetration would be a novel approach for trafficking of curcumin through the different layers of skin. The CCNGs showed selective toxicity towards melanoma cells and were free from hemolysis and toxicity on normal cells. These will find excellent application for melanoma or other skin cancers where a high selective uptake of curcumin through the transdermal route will make the treatment effective.
Acknowledgements
The Department of Biotechnology, Government of India supported this work, under a centre grant from the Nanoscience and Nanotechnology Initiative program (Ref. No. BT/PR10850/NNT/28/127/2008). This work is also partly supported by the Department of Science and Technology under the Nanoinitiative program. One of the authors, N. Sanoj Rejinold would like to acknowledge the Council of Scientific and Industrial Research (CSIR) for financial support through the Senior Research Fellowship (SRF; award No: 9/963 (0017)2K11-EMR). The authors are also thankful to Mr Sajin P. Ravi, Mr Sarath Sreenivasan, Ms Sreerekha. P. R. and Mr Sudheesh Kumar P. T. for their help in SEM, confocal, FACS, and TG/DTA studies.
References
-
S. P. Vyas and R. K. Khar, Transdermal drug delivery in Controlled Drug Delivery: Concepts and Advances 2002, Vallabh Prakashan, pp. 411–447 Search PubMed.
- K. Sungwon, M. P. Kyong, Y. K. Jin, C. K. Ick, G. C. Hyeon, K. Dongmin, T. Y. In, K. Kwangmeyung and N. Kun, Minimalism in fabrication of self-organized nanogels holding both anti-cancer drug and targeting moiety, Colloids Surf., B, 2008, 63, 55–63 CrossRef.
- K. Raemdonck, J. Demeester and S. De Smedt, Advanced nanogel engineering for drug delivery, Soft Matter, 2009, 5, 707–715 RSC.
- M. Hamidi, A. Azadi and P. Rafiei, Hydrogel nanoparticles in drug delivery, Adv. Drug Delivery Rev., 2008, 60, 1638–1649 CrossRef CAS.
- J. Zhang, S. Xu and E. Kumacheva, Polymer microgels: reactors for semiconductor, metal, and magnetic nanoparticles, J. Am. Chem. Soc., 2004, 126, 7908–7914 CrossRef CAS.
- R. M. Sawant, J. P. Hurley, S. Salmaso, A. Kale, E. Tolcheva and T. S. Levchenko, “Smart” drug delivery systems: Double-targeted pH-responsive pharmaceutical nanocarriers, Bioconjugate Chem., 2006, 17, 943–949 CrossRef CAS.
- W. Wu, T. Zhou and S. Zhou, Tunable photoluminescence of Ag nanocrystals in a p21-independent manner, Exp. Mol. Pathol., 2009, 84, 230–243 Search PubMed.
- M. A. C. Stuart, W. T. S. Huck, J. Genzer, M. Müller, C. Ober, M. Stamm, G. B. Sukhorukov, I. Szleifer, V. V. Tsukruk, M. Urban, F. Winnik, S. Zauscher, I. Luzinov and S. Minko, Emerging applications of stimuli-responsive polymer materials, Nat. Mater., 2010, 9, 101–103 CrossRef.
- W. Heike, H. K. Karl, M. L. Claus and F. S. Ulrich, Interrelation of permeation and penetration parameters obtained from in vitro experiments with human skin and skin equivalents, J. Controlled Release, 2001, 75, 283–295 CrossRef.
- H. Ehrlich, Chitin and collagen as universal and alternative templates in biomineralization, Int. Geol. Rev., 2010, 52, 661–699 CrossRef.
-
H. Ehrlich, Biological Materials of Marine Origin: Invertebrates, Springer Verlag, 2010, p. 569 Search PubMed.
- H. Ehrlich, D. Janussen, P. Simon, V. V. Bazhenov, N. P. Shapkin and C. Erler, Nanostructural organisation of naturally occurring composites: Part II. Silica-chitin-based biocomposite, J. Nanomater., 1, 670235 Search PubMed.
- H. Ehrlich, P. Simon, W. Carrillo-Cabrera, V. V. Bazhenov, J. P. Botting and M. Ilan, Insights into chemistry of biological materials: Newly discovered silica–aragonite–chitin biocomposites in demosponges, Chem. Mater., 2010, 22, 1462–1471 CrossRef CAS.
- M. Kong., X. G. Chen, D. K. Kweon and H. J. Park, Investigations on skin permeation of hyaluronic acid based nanoemulsion as transdermal carrier, Carbohydr. Polym., 2011, 86, 837–843 CrossRef CAS.
- C. Vauthier, C. Dubernet, E. Fattal, H. Pinto-Alphandary and P. Couvreur, Poly(alkylcyanoacrylates) as biodegradable materials for biomedical applications, Adv. Drug Delivery Rev., 2003, 55, 519–548 CrossRef CAS.
- B. B. Aggarwal, A. Kumar and A. C. Bharati, Anticancer potential of curcumin: Preclinical and clinical studies, Anticancer Res., 2003, 23, 363–398 CAS.
- J. Ravindran, S. Prasad and B. B. Aggarwal, Curcumin and cancer cells: How many ways can curry kill tumor cells selectively?, AAPS J., 2009, 11, 495, DOI:10.1208/s12248-009-9128-x.
- N. Sanoj Rejinold, Amrita Nair, M. Sabitha, K. P. Chennazhi, H. Tamura, S. V. Nair and R. Jayakumar, Synthesis, characterization and in vitro cytocompatibility studies of chitin nanogels for biomedical applications, Carbohydr. Polym, 2012, 87, 936–942 Search PubMed.
- N. Sanoj Rejinold, K. P. Chennazhi, H. Tamura, S. V. Nair and R. Jayakumar, Multifunctional chitin nanogels for simultaneous drug delivery, bioimaging and biosensing, ACS Appl. Mater. Interfaces, 2011, 3, 3654–3665 Search PubMed.
- M. H. Kyaw, C. Chun-Chieh and S. Lie-Fen, Current research and development of chemotherapeutic agents for melanoma, Cancers, 2010, 2, 397–419 Search PubMed.
- V. K. Lakshmanan, K. S. Snima, Joel D. Bumgardner, S. V. Nair and R. Jayakumar, Chitosan-based nanoparticles in cancer therapy, Adv. Polym. Sci., 2011, 243, 55–92 CrossRef.
- H. Tamura, H. Nagahama and S. Tokura, Preparation of hydrogels under mild conditions, Cellulose, 2006, 13, 357 CrossRef CAS.
- L. Ji, J. Yunyun, W. Jun, F. Guorong, W. Yutian and Z. Chuan, A rapid and simple HPLC method for the determination of curcumin in rat plasma: assay development, validation and application to a pharmacokinetic study of curcumin liposome, Biomed. Chromatogr., 2009, 23, 1201–1207 CrossRef.
-
U. F. Schaefer, S. Hansen, M. Schneider, J. Luengo, C. Claus and M. Lehr, Models for skin absorption and skin toxicity testing, in Drug Absorption Studies In Situ, In Vitro and In Silico Models, ed. C. Ehrhardt and K.-J. Kim, 2010, Springer International Edition, pp. 3–24 Search PubMed.
- R. L. Bronaugh, R. F. Stewart and I. R. Congdon, Methods for in vitro percutaneous absorption studies II. Animal models for human skin, Toxicol. Appl. Pharmacol., 1982, 62, 481–488 CrossRef CAS.
-
H. Raabe, R. Curren, S. Ward and J. Harbell, Report from an in vitro dermal absorption assay workshop in In Vitro Percutaneous Absorption Expert-Users Workshop, Gaithersburg, USA, 2005 Search PubMed.
- P. S. Fritz, G. M. Josef and B. Andreas, Comparison of human skin or epidermis models with human and animal skin in in vitro percutaneous absorption, Int. J. Pharm., 2001, 215, 51–56 CrossRef.
- T. J. Karpanen, T. Worthington, B. R. Conway, A. C. Hilton, T. S. J. Elliott and P. A. Lambert, Penetration of chlorhexidine into human skin, Antimicrob. Agents Chemother., 2008, 52, 3633–3636 CrossRef CAS.
- W. A. Ritschel, A. Sabouni and A. S. Hussain, Percutaneous absorption of coumarin, griseofulvin and propranalol across human scalp and abdominal skin; Methods Find, Exp. Clin. Pharmacol., 1989, 11, 643–646 CAS.
- K. Kubota, M. Sznitowska and H. I. Maibach, Percutaneous absorption: a single-layer model, J. Pharm. Sci., 1993, 82, 450–456 CrossRef CAS.
- M. Foldvari, C. J. Oguejiofor, T. W. Wilson, S. K. Afridi and T. A. Kudel, Transcutaneous delivery of prostaglandin E1: in vitro and laser doppler flowmetry study, J. Pharm. Sci., 1998, 87, 721–725 CrossRef CAS.
- R. Thakur, M. K. Anwer, M. S. Shams, A. Ali, R. K. Khar, F. Shakeel and E. I. Taha, Proniosomal transdermal therapeutic system of losartan potassium: development and pharmacokinetic evaluation, J. Drug Targeting, 2009, 17, 442–449 CrossRef CAS.
- Salaün Fabien and Vroman Isabelle, Curcumin loaded nanocapsules: formulation and influence of the nanoencapsulation processes variables on the physico-chemical characteristics of the particles, Int. J. Chem. React. Eng, 2009, 7, A55 Search PubMed.
- A. Anitha, V. G. Deepagan, V. V. Divya Rani, M. Deepthy, S. V. Nair and R. Jayakumar, Preparation, characterization, in vitro drug release and biological studies of curcumin loaded dextran sulphate–chitosan nanoparticles, Carbohydr. Polym., 2011, 84, 1158–1164 CrossRef CAS.
- N. Sanoj Rejinold, M. Muthunarayanan, V. V. Divyarani, P. R. Sreerekha, K. P. Chennazhi, S. V. Nair, H. Tamura and R. Jayakumar, Curcumin-loaded biocompatible thermo responsive polymeric nanoparticles for cancer drug delivery, J. Colloid Interface Sci., 2011, 360, 39–51 CrossRef CAS.
- A. V. Kabanov and S. Vinogradov, Nanogels as pharmaceutical carriers: finite networks of infinite capabilities, Angew. Chem., Int. Ed., 2009, 48, 5418–5429 CrossRef CAS.
- S. B. Murray and A. C. Neville, The role of pH, temperature and nucleation in the formation of cholesteric liquid crystal spherulites from chitin and chitosan, Int. J. Biol. Macromol., 1998, 22, 137–144 CrossRef CAS.
- Y. J. Wang, M. H. Pan, A. L. Cheng, L. I. Lin, Y. S. Ho, C. Y. Hsieh and J. K. Lin, Stability of curcumin in buffer solutions and characterization of its degradation products, J. Pharm. Biomed. Anal., 1997, 15, 1867–1876 CrossRef CAS.
- C. Syng-Ai, A. L. Kumari and A. Khar, Effect of curcumin on normal and tumor cells: role of glutathione and bcl-2, Mol. Cancer Ther., 2004, 3, 1101–1108 CAS.
- S. Shishodia, H. M. Amin, R. Lai and B. B. Aggarwal, Curcumin (diferuloylmethane) inhibits constitutive NF-kappaB activation, induces G1/S arrest, suppresses proliferation, and apoptosis in mantle cell lymphoma, Biochem. Pharmacol., 2005, 70, 700–713 CrossRef CAS.
- J. L. Watson, R. Hill, P. W. Lee, C. A. Giacomantonio and D. W. Hoskin, Curcumin induces apoptosis in HCT-116 human colon cancer cells, Chem. Mater., 2008, 21, 2851–2861 Search PubMed.
- A. Scharstuhl, H. A. Mutsaers, S. W. Pennings, W. A. Szarek, F. G. Russel and F. A. Wagener, Curcumin-Induced fibroblast apoptosis and in vitro wound contraction are regulated by antioxidants and heme oxygenase: implications for scar formation, J. Cell. Mol. Med., 2009, 13, 712–725 CrossRef CAS.
- D. N. Ngo, S. H. Lee, M. M. Kim and S. K. Kim, Production of chitin oligosaccharides with different molecular weights and their antioxidant effect in RAW 264.7 cells, J. Funct. Foods, 2009, 1, 188–198 CrossRef CAS.
- Y. Wang, I. Okan, L. Szekely, G. Klein and K. G. Wiman, Bcl-2 inhibits wild-type p53-triggered apoptosis but not G1 cell cycle arrest and transactivation of WAF1 and Bax, Cell Growth Differentiation, 1995, 6, 1071–1075 CAS.
- X. Liu, C. N. Kim, J. Yang, R. Jemmerson and X. Wang, Induction of apoptotic program in cell-free extracts: requirement for dATP and cytochrome C, Cell, 1996, 86, 147–157 CrossRef CAS.
- T. Choudhuri, S. Pal, T. Das and G. Sa, Curcumin selectively induces apoptosis in deregulated cyclin D1-expressed cells at G2 phase of cell cycle in a p53-dependant manner, J. Biol. Chem., 2005, 280, 20059–20068 CrossRef CAS.
- N. M. Weir, K. Selvendiran, V. K. Kutala, L. Tong, S. Vishwanath, M. Rajaram, S. Tridandapani, S. Anant and P. Kuppusamy, Curcumin induces G2/M arrest and apoptosis in cisplatin-resistant human ovarian cancer cells by modulating Akt and p38 MAPK, Cancer Biol. Ther., 2007, 6, 178–184 CrossRef CAS.
- E. Liu, J. Wu, W. Cao, J. Zhang, W. Liu, X. Jiang and X. Zhang, Curcumin induces G2/M cell cycle arrest in a p53-dependent manner and upregulates ING4 expression in human glioma, J. Neuro-Oncol., 2007, 85, 263–270 CrossRef CAS.
- C. Gajate and F. Mollinedo, Cytoskeleton-mediated death receptor and ligand concentration in lipid rafts forms apoptosis-promoting clusters in cancer chemotherapy, J. Biol. Chem., 2005, 280, 11641–11647 CrossRef CAS.
- H. F. Lu, K. C. Lai, S. C. Hsu, H. J. Lin, M. D. Yang, Y. L. Chen, M. J. Fan, J. S. Yang, P. Y. Cheng, C. L. Kuo and J. G. Chung, Curcumin induces apoptosis through FAS and FADD, in caspase-3-dependent and -independent pathways in the N18 mouse-rat hybrid retina ganglion cells, Oncol. Rep., 2009, 22, 97–104 CAS.
- J. A. Bush, K. J. Cheung and G. Li, Curcumin induces apoptosis in human melanoma cells through a Fas receptor/caspase-8 pathway independent of p53, Exp. Cell Res., 2001, 271, 305–314 CrossRef CAS.
- B. Ballarin, S. Galli, F. Mogavero and M. Morigi, Effect of cationic charge and hydrophobic index of cellulose based polymers on the semipermanent dyestuff process for hair, Int. J. Cosmet. Sci., 2011, 33, 228–233 CrossRef CAS.
-
A. O. Barell, M. Paye and H. I. Maibach, Handbook of Cosmetic Science and Technology, Marcel Dekker, 2001, p. 407 Search PubMed.
- M. Miettinen and K. Franssila, Immunohistochemical spectrum of malignant melanoma, the common presence of keratins, Lab. Invest., 1989, 61, 623–628 CAS.
- H. S. Liu, F. U. Chang and D. K. Hung, Terpene microemulsions for transdermal curcumin delivery: effect of terpenes and cosurfactants, Colloids Surf., B, 2011, 82, 63–70 CrossRef.
|
This journal is © The Royal Society of Chemistry 2012 |