DOI:
10.1039/C1LC20626E
(Paper)
Lab Chip, 2012,
12, 139-145
Lateral cavity acoustic transducer as an on-chip cell/particle microfluidic switch†
Received
12th July 2011
, Accepted 8th September 2011
First published on 10th November 2011
Abstract
A novel on-chip microfluidic switch is demonstrated that utilizes the acoustic microstreaming generated by an oscillating air–liquid interface to switch cells/particles into bifurcating microchannels. The air–liquid interface of the Lateral Cavity Acoustic Transducers (LCATs) can be actuated by an external acoustic energy source causing the interface to oscillate. The oscillating interface results in the generation of vortex-like microstreaming flow within a localized region of the surrounding liquid. This streaming was utilized here to deflect cells/particles into a collection outlet. It was demonstrated that the switching zone could be controlled by varying the actuation time of the LCAT. An LCAT based microfluidic switch is capable of achieving theoretical switching rates of 800 cells/particles per second. It was also demonstrated that K562 cells could be switched into a collection channel with cell viability comparable to that of controls as determined by Trypan blue exclusion assay.
Introduction
The rapid growth of microfluidic technologies in the last several decades has led to the development of various degrees of micro total analysis systems. Specifically, the application of microfluidic technologies to the field of cell biology have enabled the development of “lab on a chip” systems that are capable of integrating multiple laboratory steps on to a single device.1 An important cell manipulation process that needs to be integrated into a “lab on a chip” system is the ability to sort cells of interest to multiple downstream processes for further analysis.
Multiple microfluidic switches for particles and cells have been developed including electro-osmotic flow (EOF),2dielectrophoresis,3,4 microfabricated valves,5 external valves6 and optical tweezers.7 These microfluidic switches were later utilized in integrated micro fluorescence activated cell sorting (μFACS) systems.8–13 However, many of the current microfluidic switches have several drawbacks such as low throughput (20 cells s−1,8 44 cells s−1,9 1 cell s−1,10 and 106 cells s−1 11) and low cell recovery (20%,8 50%9 and 73.6%11). Furthermore, some require complex off-chip optical tweezers,11 pneumatic valves12 or high voltage (kV) droplet sorting platforms.13
A sorting platform that utilizes surface acoustic waves (SAW) to generate microstreaming within a microchannel was demonstrated by Franke et al.14 This platform was shown to achieve switching rates of several kHz per second with cell viabilities of 94%. The microfluidic device has a relatively large power requirement of 1 watt and the device must be fabricated using a piezoelectric substrate in order to generate the SAW required to switch cells. An on-chip piezoelectric actuated micro-switch was recently demonstrated and was shown to achieve cell sorting throughput of ∼330–1000 cells s−1.15,16 This platform utilizes the deflection of a lead-zirconate-titanate(PZT)–stainless steel bimorphous structure when a voltage is applied to displace a minute volume of fluid at the sorting junction and the particulates contained within it. This system was able to demonstrate a low-power (<1 mW) on-chip actuator to deflect cells to the collection outlets. The system requires additional fabrication steps to permanently bond the PZT to the device in order to produce leakage-free coupling between the PZT and the microchannel.
Recently, there has been increasing interest in utilizing acoustically excited bubbles within microchannels as simple on-chip actuator systems for applications in pumping, mixing and trapping.17–25 Specifically, Lateral Cavity Acoustic Transducers (LCATs) are versatile, simple on-chip actuators that are easily fabricated and can be actuated using a battery operated portable electronics platform.26–29 LCATs are dead-end side channels that are in the same plane as the microchannels themselves requiring no additional fabrication steps other than those needed to produce the single layer device (Fig. 1A). When the device is filled with liquid, LCATs trap bubbles creating an air–liquid interface that can be excited using an external acoustic source such as a piezoelectric transducer. Previously we had demonstrated that LCATs are capable of deflecting beads into downstream microchannels.29 In this paper, we aim to fully characterize LCATs as an on-chip microfluidic switch that is capable of deflecting cells and particles to downstream collection channels. In addition, the switching zone can be controlled based on the actuation time of the LCATs. Theoretical switching rates that can be achieved for the parameters used within this manuscript are in the range of ∼150–800 particles s−1. An average cell viability of 94% was achieved with K562 cells based on the Trypan blue exclusion assay which is comparable to controls. Furthermore, the power consumption of the LCAT switch is 2.3 mW, making it a low-power on-chip actuator that does not require additional fabrication steps. These features make an LCAT cell/particle switch an ideal candidate for integration into a complete microfluidic sorting platform.
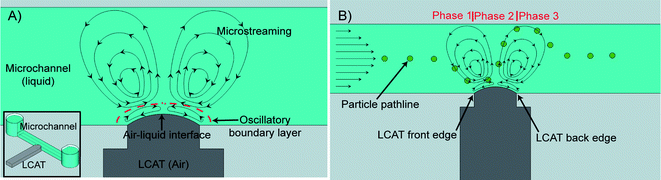 |
| Fig. 1 The inset in (A) shows a graphical depiction of an LCAT. (A) The oscillatory motion of the air–liquid interface generates steady vortex-like streaming. (B) When the LCAT is activated and a bulk flow is present, particles will be transported from the inlet reservoir to the outlet channels. As the particles flow past the activated LCAT, they will be exposed to different phases of the microstreaming flow. The resulting drag force will deflect the particle in the direction perpendicular to the bulk flow as shown. | |
Mechanism of LCAT based cell and particle switching
LCATs exploit the phenomenon of acoustic microstreaming to manipulate fluid flow and suspended cells/particles within a microfluidic environment. Acoustic microstreaming using gaseous bubbles as the actuation source has been utilized in the past to achieve effective fluid transport and mixing.17–26,29 Bubble-induced acoustic microstreaming develops when bubbles trapped within a liquid phase oscillate. The oscillatory motion of the air–liquid interface leads to a first-order periodic flow within the liquid, with the period being the angular frequency of the applied sound field. At the bubble boundary the magnitude of the acoustically excited flow is at a maximum and is given bywhere d is the oscillation amplitude of the interface and ω is the angular frequency of the acoustic field. Within the oscillatory boundary layer, the first-order periodic flow induces a steady second-order streaming due to the balance between the viscous forces and the nonlinear inertial forces (i.e., Reynolds stresses) at the interface (Fig. 1A).19,30,31 The thickness of the oscillatory boundary layer δ, is described bywhere ν is the kinematic viscosity of the fluid.32 The magnitude of the steady microstreaming within the oscillatory boundary layer is: |  | (3) |
where us is the velocity of the streaming within the boundary layer and R is the length scale of the oscillating interface. For the frequencies reported here, the thickness of the boundary layer is on the order of several micrometres. However, the slip-conditions between the edge of the oscillatory boundary and the bulk fluid drives a steady vortex-like streaming well beyond the edge of the boundary layer (Fig. 1A).32 These second-order streaming flows within the bulk fluid are utilized here to switch cells and particles.
When activated by an external acoustic energy source, maximum displacement of the air–liquid interface occurs at the center, while the edges of the interface in contact with the PDMS walls of the device remain relatively fixed. This type of interface motion induces second-order streaming flows that resemble those previously described.33 They are symmetrical to the center of the interface and will generate a vortex type flow as shown in Fig. 1A. The LCAT switch platform manipulates particle paths within a microfluidic switching system by combining the microstreaming flow of an actuated LCAT with the bulk flow as shown in Fig. 1B. When the particles flow through an actuated LCATvia the bulk flow it will experience three different phases of microstreaming flow. In the first phase, the particle will experience a drag force due to the microstreaming that will pull the particle towards the oscillating air–liquid interface. As the particle arrives at the front edge of the LCAT as shown in Fig. 1B, the flow direction in the second phase is pointed away from the interface. This will exert a drag force on the particle in the direction perpendicular to the bulk flow. The highest velocities of the microstreaming occur at the center of the air–liquid interface. This results in the particle having a maximum deflection during this phase. When it reaches the back edge of the LCAT, the microstreaming flow in the third phase will be pulling it towards the center of the microchannel as shown in Fig. 1B. The magnitude of the streaming velocity decreases as 1/r3 where r is the distance from the center of the bubble.18 This will result in a larger drag force being applied to the particle while it is closer to the interface compared to when it is farther away. During Phase 3 the drag force on the particle will be lower than the force that causes the particle to deflect away from the LCAT interface during Phase 2. Therefore, it is expected that there will be a resulting change in particle position away from the interface at the end of Phase 3 compared to the input position. The ability of an actuated LCAT to deflect particles in this manner is utilized for applications in continuous microfluidic cell/particle switching.
Computational fluid dynamic (CFD) simulation
In order to better characterize particle trajectories induced by actuated LCATs, 3-dimensional CFD simulations using the software package CFD-ACE+ v2008 (ESI Group, Inc., France) were performed. The simulation was set up using fluid flow and particle spray modules on a straight microchannel of dimensions 100 μm wide, 50 μm thick and 700 μm long with a structured grid of 10 μm3 throughout (Fig. 2). The oscillations of the interface were modeled as an inlet with a time varying velocity profile as shown in Fig. 2 and described by the following equation: |  | (4) |
This profile was used because it models the first degree motion of a rectangular membrane of dimensions w = 60 μm and l = 50 μm. The frequency of oscillation was 32 kHz and the peak to peak amplitude of the membrane (2d) was set to 12 μm at the center. Membrane amplitude was set to 12 μm because it was determined using ImageJ (NIH Image) that for an applied voltage of 20 Vpp, membrane amplitude varied from ∼10–12 μm. The total bulk flow rate in the microchannel was set to 5 μL min−1 at the inlet and the pressure at the outlet was set to the reference pressure of 100 kPa. A no-slip boundary condition was applied to all the walls within the microchannel. Seven 10 μm polystyrene beads were placed at the center of the channel along the z-axis (height of the channel) approximately 120 μm upstream of the LCAT (Fig. 2).
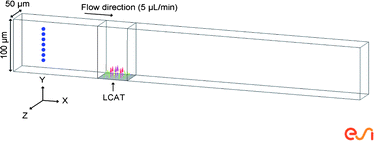 |
| Fig. 2 Straight channel simulation setup. The length of the microchannel is 700 μm. 10 μm polystyrene beads are placed in the center of the channel along the z-axis. The flow induced by the oscillations of an acoustically actuated LCAT is modeled as an inlet with a time varying flow profile with a frequency of 32 kHz and a membrane oscillation amplitude of 12 μm peak to peak that is 60 μm long and 50 μm wide. | |
Simulation parameters
The simulation was solved using constant time steps of 2 μs which results in approximately 16 time steps per period of the air–liquid interface oscillation. Forces due to gravity were considered negligible and the reference pressure and temperature were set at 100 kPa and 300 K respectively. Forces due to drag were included in the spray module with the drag coefficient set to incompressible. The inlets were treated as walls with the coefficient of restitution (COR) set to 1.0 for the normal and parallel components of the particle velocity upon collision with a microchannel wall. Initial velocity of all the fluid in the microchannel was set to 1 × 10−9 m s−1. The maximum iterations per time step were set to 40 with a convergence criterion of 0.0001 and a minimum residual of 1 × 10−18. An upwind spatial differencing method was used for velocity and the inertial relaxation for velocity was set to 0.2, while the linear relaxation was set to 1 for pressure, density and viscosity. The simulation was run for ∼22 ms, in which all particles have flowed past the actuated LCAT and towards the outlet. Properties of water at 300 K were used for the simulations with a dynamic viscosity of μ = 8.55 × 10−4 kg m−1 s−1 and density of ρ = 997 kg m−3.
Simulation results
The 10 μm polystyrene particle pathlines from simulations conducted on a straight microchannel with a continuously activated LCAT are shown in Fig. 3. The particle pathlines observed through simulations resemble those described above in Fig. 1B. Specifically, three distinct phases of particle deflection are observed. Initially as the particles flow towards the LCAT, all are pulled towards the air–liquid interface. In the second phase, as the particles flow between the front and back edge of the interface they are pushed away from the cavity due to the direction of microstreaming flow. As they flow past the back edge of the LCAT they are again pulled towards the center of the microchannel due to the direction of the streaming in that phase. Furthermore, particles closest to the LCAT had the largest deflection in the order of ∼50 μm, while particles furthest from the LCAT had the smallest deflection of ∼10 μm. The simulation demonstrates that an actuated LCAT is capable of deflecting all particles in the direction perpendicular to the flow and that the magnitude of the deflection decreases as the initial distance between the air–liquid interface and the particle increases. This behavior suggests that particles should be focused closer to the interface in order to take advantage of the large particle deflection for optimal switching. As described above, the maximum deflection of all particles occurs at x = 30 μm, the position where the back edge of the air–liquid interface is located along the x-axis (marked by the dashed orange line in Fig. 3). Therefore, a microfluidic device designed to have a symmetric bifurcation at the back edge of the LCAT (shown in gray in Fig. 3) would enable the switching of particles and cells effectively. Symmetrical bifurcating outlet channels with equal channel resistance would require an asymmetrical particle flow focusing scheme. This allows all particles to flow into the outlet channel closest to the interface (waste channel) when the LCAT is not actuated. However, when the LCAT is actuated all particles will flow into the collection channel due to the large deflection of particles away from the interface. Simulation results of a microfluidic device with a symmetric bifurcation at the back edge of the LCAT are shown in ESI Image S1.† The velocity field that is generated due to the interaction between the microstreaming generated by an LCAT and the bulk flow is also simulated in ESI Image S2.†
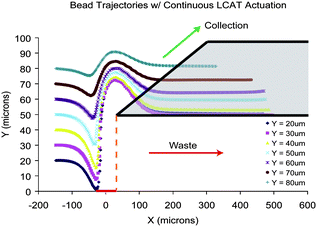 |
| Fig. 3 Simulation results from Fig. 2. Graph showing particle pathlines with the z-axis coming out of the page. Red rectangle on the x-axis indicates the location of the LCAT interface. Particles that start off closer to the interface have the largest deflection. The maximum deflection occurs at x = 30 μm, the back edge of the LCAT (dashed orange line). The gray polygon indicates where the bifurcation should be designed. | |
Materials and methods
Device fabrication
LCAT switch devices were fabricated using standard soft-lithography techniques. The devices consist of polydimethylsiloxane (PDMS) as the top substrate and a 150 μm thick glass coverslip as the bottom substrate. The PDMS top substrate (Sylgard 184, Dow Corning Corp., USA) with the microchannels are molded from an SU-8 master fabricated on a 3′′ silicon wafer. SU-8 50 (Microchem Corp., USA) is spun coated according to manufacturer's recommendations to obtain a photoresist layer thickness of ∼50 μm on the wafer. This is exposed using a UV flood lamp through a transparency mask to produce the desired microchannel patterns after development using SU-8 developer (Microchem Corp., USA). A 10
:
1 ratio of PDMS base to curing agent is completely mixed, degassed and poured onto the SU-8 master and cured at 60 °C for 4 h. The devices are cut and plasma bonded to glass microscope coverslips after a 2 min 30 s air plasma treatment of the surfaces at 250 mtorr. Upon bonding, the devices are allowed to sit at room temperature for 10 min to increase the bond strength between the PDMS and glass substrates. Rain-X (SOPUS Products, USA) is flowed through the device and allowed to incubate at room temperature for 10 min before being vacuumed out to form a hydrophobic coating on the walls of the microchannels. This method is similar to that used by Kim et al.34 These devices are then placed in a 120 °C oven for 10 min and are then allowed to sit at room temperature for at least 24 h before being used for experimentation.
Experimental setup
The manifold that interfaces the piezoelectric transducer to the microfluidic device consists of a 7 cm × 7 cm × 1 cm acrylic piece with an 11 mm hole drilled in its center. A 10 mm piezoelectric transducer (SMATR10H40X80, Steiner and Martins, Inc., USA) is inserted into the center and a thin layer of PDMS (∼350 μm thick) is poured on to the top of the platform and allowed to cure at room temperature for 24 h. The LCAT switch device is placed on the platform (Fig. 4) and couples with the piezoelectric transducer due to the reversible bond between the bottom substrate of the device (glass coverslip) and the thin layer of PDMS on the platform.
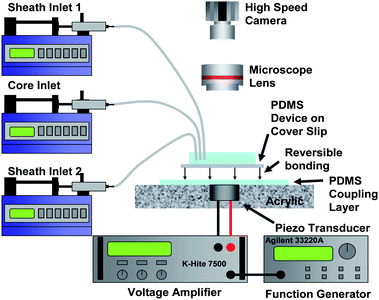 |
| Fig. 4 PDMS/coverslip devices are placed on top of a custom fabricated platform. A thin layer (∼350 μm thick) of PDMS is poured onto the acrylic piece. The reversible bond between the device and platform allows the transducer to be reused. Images and videos are captured using a combination of an upright microscope and a high speed CCD camera. | |
A schematic of the microfluidic device is shown in Fig. 5. Sheath fluid 1 and 2 are used to asymmetrically focus the core fluid (suspension of particles/cells) ensuring that particles/cells flow into the waste outlet channel when the LCAT is not actuated. The flow rates used for all experiments are 3 μL min−1 for sheath fluid 1, 1.2 μL min−1 for sheath fluid 2 and 0.8 μL min−1 for the core fluid and were controlled by syringe pumps (PicoPlus, Harvard Apparatus, USA). The piezoelectric transducer is supplied with a 32 kHz sinusoidal signal from a function generator (Agilent 33220A, Agilent Technologies, USA) and voltage amplifier (Krohn-Hite 7500, Krohn-Hite Corp., USA) with voltages ranging from 20–27 Vpp. The actuated transducer will cause the air–liquid interface of the LCAT to oscillate resulting in acoustic microstreaming within the microchannel. The power consumption of the LCAT was measured using a digital multimeter (HP 34401A, Hewlett Packard Co., USA).
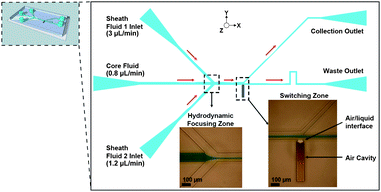 |
| Fig. 5 Image of the complete device geometry. Three inlet channels enable asymmetrical hydrodynamic focusing of cells and particles as they flow through the core inlet. Bifurcating outlet channel geometry at the back edge of the LCAT enables the switching of cells and particles. When the LCAT is in the ON state they are deflected into the collection channel. | |
Switch characterization
The device was characterized in order to determine the region around the LCAT where a particle would be switched to the collection outlet when the LCAT is actuated. This region is referred to as the switching zone of the LCAT. In order to characterize the switching zone, water was used as sheath fluid 1 at 3 μL min−1 and sheath fluid 2 at 1.2 μL min−1, while 10.0 ± 0.7 μm polystyrene beads (SPI Supplies, Inc., USA) suspended in a sucrose/deionized water solution of density 1.052 g mL−1 was flowed through the core inlet at 0.8 μL min−1. The LCAT was actuated with two different sets of actuation times (1) 100 cycles at 32 kHz, ∼3.1 ms of ON time and ∼3.1 ms of OFF time and (2) 50 cycles at 32 kHz, ∼1.6 ms of ON time and ∼4.7 ms of OFF time) with a 32 kHz sine wave and a voltage of 20 Vpp. An LCAT ON time of 3.1 ms was chosen as it allows particles with the largest velocity (twice the average velocity) to be exposed to multiple phases of microstreaming flow. While an LCAT ON time of 1.6 ms was chosen as it allows particles with the largest velocity to be exposed to a single phase of microstreaming flow. A high speed camera (Phantom v310, Vision Research Inc., USA) captured images at 5000 fps with a 19 μs exposure time. Switching zones were determined by analyzing the videos frame by frame. The velocity and position of each particle relative to the LCAT was calculated using ImageJ (NIH Image) particle analyzer function.
Cell viability characterization
In order to determine if the LCAT switch platform is capable of switching cells, K562 leukemia cells were flowed through the device and their viability was quantified using Trypan blue exclusion assay. First, K562 cells were spun down at 1000 rpm for 5 min and resuspended in culture media at a density of ∼9–13 × 106cells mL−1. Phosphate buffered saline (PBS) was used as the sheath fluid at a flow rate of 3 μL min−1 (Sheath fluid 1) and 1.2 μL min−1 (Sheath fluid 2) while the cell suspension flow rate was 0.8 μl min−1 (Core fluid). LCAT was actuated for a 1 s ON time and a 1 s OFF time with a 32 kHz sine wave and a voltage ranging from 23–27 Vpp. The device was allowed to switch cells for at least 1 h while a high speed camera was used to monitor the device to ensure proper function. Short videos were taken every 10 min and were analyzed to quantify the efficiency of the switch. Cells were allowed to accumulate in wells punched out at the collection and waste outlets. Cells from the collection and waste wells along with a control (cells not flowed through the device) were spun down at 1000 rpm for 5 min and resuspended into ∼30 μL of PBS. An equal volume of 0.4% Trypan blue was mixed with the cell suspension and allowed to incubate at room temperature for 5 min. Cells were counted using a hemocytometer to determine cell viability.
Results and discussion
Switch characterization
Experiments were performed on LCAT switch devices in order to determine their switching zone using 10 μm polystyrene beads. The results of the experiments with an ON time of 3.1 ms and an ON time of 1.6 ms are shown in Fig. 6. The position of the particles along the width (y-direction) of the channel was controlled by the hydrodynamic focusing into a narrow band of ∼24 μm due to the flow rates ratios of 3.0
:
0.8
:
1.2 μL min−1 for sheath fluid 1, core fluid and sheath fluid 2 respectively. The x-axis of the plot shows the starting/initial position of the 10 μm polystyrene beads relative to the center of the air–liquid interface prior to LCAT actuation and the y-axis indicates their starting velocities calculated using the particle analyzer function in ImageJ. The points in green and blue represent the particles that were deflected significantly and were switched to the collection channel from two different experimental runs, while all others represent particles that did not experience sufficient force to deflect into the collection channel and instead continued to flow towards the waste channel.
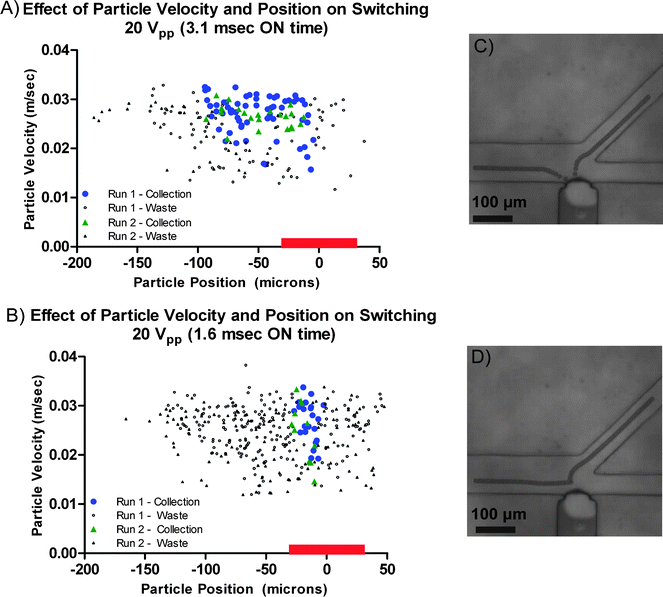 |
| Fig. 6 Scatter plot showing the LCAT switching zone for 10 μm polystyrene particles for an (A) LCAT ON time of 3.1 ms. Red bar on the x-axis of the plot indicates the location of the LCAT. Particles are switched to the collection channel within a narrow region of −15 μm < x < −6 μm independent of their initial velocity. The switching of particles with an initial position of ∼100 μm < x < −15 μm is greatly dependent on velocity. (B) LCAT ON time of 1.6 ms. Switching zone for an LCAT ON time of 1.6 ms was reduced to 20 μm, 20% of that observed for an ON time of 3.1 ms. Micrographs (C) and (D) show typical pathlines observed for an LCAT ON time of 3.1 ms, while (D) shows the typical pathline observed for an LCAT ON time of 1.6 ms. | |
As shown in Fig. 6 there is a finite window in which particles affected by the LCAT will be switched to the collection channel. The switching zone is at its widest (∼100 μm) for particles whose initial velocity is greater than 25 mm s−1. Particles whose position was within the range of −15 μm < x < −6 μm prior to the start of LCAT actuation were deflected to the collection channel independent of their initial velocity. However, for particles whose initial position was to the left of −15 μm, only those with higher velocities were switched. See ESI Video S3† which shows 10 μm polystyrene particles being switched to the top collection channel with an LCAT ON time of 3.1 ms. Comparing the particle pathlines from the videos and the simulations of the velocity field generated (ESI Image S2†) shows that the acoustic microstreaming induced drag force plays the dominant role on the trajectory of the particles. However, it is important to note that the oscillations of the interface will generate a secondary Bjerknes force on the particles suspended within the liquid. The secondary Bjerknes force is the time-average force that is generated between an oscillating bubble and a rigid particle.35,36 When a particle is denser than the fluid in which it is suspended (polystyrene particles in DI water) the particles will be attracted towards the oscillating interface. Experimental results show that particles are not attracted and retained on the air–liquid interface, indicating that the secondary Bjerknes force is negligible compared to the streaming induced drag force.
As the LCAT ON time is decreased to 1.6 ms the switching zone is reduced significantly to approximately 20 μm in width compared to ∼100 μm for an LCAT ON time of 3.1 ms (Fig. 6). With the shorter LCAT ON time of 1.6 ms, the LCAT actuation time is not long enough for those particles further upstream to be pulled towards the interface initially and be pushed away towards the collection channel. As can be seen in the stacked image of a typical particle pathline for an LCAT ON time of 1.6 ms, only those particles that are located closer to the center of the LCAT are deflected away from the interface in accordance with the acoustic microstreaming flow in that region. See ESI Video S4† which shows 10 μm polystyrene particles being switched to the top collection channel with an LCAT ON time of 1.6 ms.
It is important to note that the initial velocity of particle within the 20 μm switching zone does not significantly determine whether it is switched to the collection channel. The different initial velocities indicate that the particle is flowing along different heights within the microchannel. Since particle velocity does not determine whether it is switched to the collection channel, 3-dimensional particle focusing is not required to ensure that the particles are within the center of the microchannel along the z-axis. This will greatly reduce the complexity of a microfluidic sorting platform based on the LCAT switch.
Taking into consideration the average velocity of particles flowing through the microchannel for a 5 μL min−1 flow rate used in the experiments conducted (calculated to be ∼17 mm s−1) and the minimum required interparticle distance of 20 μm (based on the width of the switching zone) the calculated theoretical switching rate of the system should be ∼800 particles s−1. Further increase in the flow rate, optimization of the voltage applied to the PZT as well as the LCAT ON time will allow for faster switching rates resulting in an increased throughput of an LCAT based sorting platform.
Cell viability characterization
Although the LCAT based switch device was characterized using 10 μm polystyrene beads in order to determine its switching zone, it is essential to determine if an LCAT switch would be capable of switching cells. K562 cells were flowed through the device and switched into the collection and waste outlets as described in the previous section. With the LCAT in the ON state for 1 s, cells were observed to flow into the collection outlet, while with the LCAT in the OFF state for 1 s, cells flowed into the waste outlet. Analysis of the videos that were taken every 10 min for all experiments showed that over 99% (123/124) of the K562 cells that flowed passed an LCAT in the ON state were switched to the collection channel. A video of K562 cells being switched is available in ESI Video S5.† Therefore, cells in the collection well were exposed to the microstreaming induced by the LCAT while cells in the waste well were not. The Trypan blue exclusion assay results are shown in Table 1. Multiple switching experiments were conducted (n = 3) with each experiment lasting more than 1 h. Trypan blue exclusion assays were performed using a hemocytometer on cells collected from collection, waste and control (cells not flowed through the device) populations. For each experiment conducted, at least 300 cells were counted from each population.
Table 1
Cell viability was determined by Trypan blue exclusion assay from both collection and waste wells along with controls (cells not flowed through the device). For each experiment (n = 3) conducted, at least 300 cells were counted from each population
|
Cell viability (%) |
Collection |
94.5 ± 4.7 |
Waste |
96.2 ± 0.5 |
Control |
97.8 ± 2.1 |
Although cell viability in both collection and waste channels were slightly lower than those of the control, an average cell viability of 94% was obtained, which is comparable to or exceeds previously reported values for cell viabilities for other microfluidic cell switches.10,11,14,16 This indicates that an LCAT switch does not compromise the membrane of cells and can be utilized as an actuator for an integrated sorting platform.
Conclusion
In the past decade, significant progress has been made on the development of integrated microfluidic platforms for cell biology. However, the cell/particle switching mechanisms utilized have their drawbacks as described previously. In this manuscript we demonstrate the use of LCATs as a microfluidic switch for particles and cells. The air–liquid interface of the LCATs can be actuated by an external acoustic energy source causing the interface to oscillate. The oscillating interface results in the generation of acoustic microstreaming patterns within a localized region of the surrounding liquid. These acoustic microstreaming patterns were utilized here to deflect cells/particles into a collection outlet channel. It was demonstrated that the switching zone of the LCAT could be controlled by varying the actuation time. With a total flow rate of 5 μL min−1 within a 100 μm × 50 μm microchannel and an LCAT ON time of 1.6 ms, the width of the switching zone can be controlled to ∼20 μm in width. Based on these parameters an LCAT switch is capable of achieving theoretical switching rates of 800 cells/particles per second. It was also demonstrated that K562 cells could be switched into a collection channel with an average cell viability of 94% as determined by Trypan blue exclusion assay and is comparable to controls. Furthermore, the low power consumption (2.3 mW) of an LCAT switch makes it possible to develop the electronic driving circuits required into a portable battery driven platform. These features make the LCAT switch an ideal candidate for integration into a complete microfluidics FACS platform.
Acknowledgements
This work was partially funded by the Micro/Nano Fluidics Fundamentals Focus (MF3) Center under the DARPA N/MEMS Science and Technology Fundamentals Program, Grant No. N66001-10-1-4003. The authors acknowledge Dr Randy Wei for providing us with the K562 (leukemia) cell line. The authors would also like to acknowledge Dr Shia-Yen Teh, Dr Robert Lin and Jente Lu for helpful discussions.
References
- J. El-Ali, P. K. Sorger and K. F. Jensen, Nature, 2006, 442, 403–411 CrossRef CAS.
- D. C. Duffy, O. J. A. Schueller, S. T. Brittain and G. M. Whitesides, J. Micromech. Microeng., 1999, 9, 211 CrossRef CAS.
- S. Fiedler, S. G. Shirley, T. Schnelle and G. Fuhr, Anal. Chem., 1998, 70, 1909–1915 CrossRef CAS.
- K. Ahn, C. Kerbage, T. P. Hunt, R. M. Westervelt, D. R. Link and D. A. Weitz, Appl. Phys. Lett., 2006, 88, 024104 CrossRef.
- M. A. Unger, H.-P. Chou, T. Thorsen, A. Scherer and S. R. Quake, Science, 2000, 288, 113–116 CrossRef CAS.
-
P. Telleman, U. D. Larsen, J. Philip, G. Blankenstein and A. Wolff, in Proceedings of the Micro Total Analysis Systems '98 Workshop, Banff, Canada, 1998, pp. 39–44 Search PubMed.
- T. N. Buican, M. J. Smyth, H. A. Crissman, G. C. Salzman, C. C. Stewart and J. C. Martin, Appl. Opt., 1987, 26, 5311–5316 CrossRef CAS.
- A. Y. Fu, C. Spence, A. Scherer, F. H. Arnold and S. R. Quake, Nat. Biotechnol., 1999, 17, 1109–1111 CrossRef CAS.
- A. Y. Fu, H.-P. Chou, C. Spence, F. H. Arnold and S. R. Quake, Anal. Chem., 2002, 74, 2451–2457 CrossRef CAS.
- P. S. Dittrich and P. Schwille, Anal. Chem., 2003, 75, 5767–5774 CrossRef CAS.
- M. M. Wang, E. Tu, D. E. Raymond, J. M. Yang, H. Zhang, N. Hagen, B. Dees, E. M. Mercer, A. H. Forster, I. Kariv, P. J. Marchand and W. F. Butler, Nat. Biotechnol., 2005, 23, 83–87 CrossRef CAS.
- A. Wolff, I. R. Perch-Nielsen, U. D. Larsen, P. Friis, G. Goranovic, C. R. Poulsen, J. P. Kutter and P. Telleman, Lab Chip, 2003, 3, 22–27 RSC.
- J.-C. Baret, O. J. Miller, V. Taly, M. Ryckelynck, A. El-Harrak, L. Frenz, C. Rick, M. L. Samuels, J. B. Hutchison, J. J. Agresti, D. R. Link, D. A. Weitz and A. D. Griffiths, Lab Chip, 2009, 9, 1850–1858 RSC.
- T. Franke, S. Braunmuller, L. Schmid, A. Wixforth and D. A. Weitz, Lab Chip, 2010, 10, 789–794 RSC.
- C. H. Chen, S. H. Cho, F. Tsai, A. Erten and Y.-H. Lo, Biomed. Microdevices, 2009, 11, 1223–1231 CrossRef CAS.
- S. H. Cho, C. H. Chen, F. S. Tsai, J. M. Godin and Y.-H. Lo, Lab Chip, 2010, 10, 1567–1573 RSC.
- R. H. Liu, J. Yang, M. Z. Pindera, M. Athavale and P. Grodzinski, Lab Chip, 2002, 2, 151–157 RSC.
- P. Marmottant and S. Hilgenfeldt, Proc. Natl. Acad. Sci. U. S. A., 2004, 101, 9523–9527 CrossRef CAS.
- P. Marmottant, J. P. Raven, H. Gardeniers, J. G. Bomer and S. Hilgenfeldt, J. Fluid Mech., 2006, 568, 109–118 CrossRef.
- J. Xu and D. Attinger, J. Micromech. Microeng., 2007, 17, 609 CrossRef CAS.
- S. K. Chung and S. K. Cho, J. Micromech. Microeng., 2008, 18, 125024 CrossRef.
- D. Ahmed, X. Mao, J. Shi, B. K. Juluri and T. J. Huang, Lab Chip, 2009, 9, 2738–2741 RSC.
- D. Ahmed, X. Mao, B. K. Juluri and T. J. Huang, Microfluid. Nanofluid., 2009, 7, 727–731 CrossRef CAS.
- S. S. Wang, Z. J. Jiao, X. Y. Huang, C. Yang and N. T. Nguyen, Microfluid. Nanofluid., 2009, 6, 847–852 CrossRef CAS.
- K. Ryu, S. K. Chung and S. K. Cho, J. Assoc. Lab. Autom., 2010, 15, 163–171 CrossRef.
- A. R. Tovar and A. P. Lee, Lab Chip, 2009, 9, 41–43 RSC.
-
J. L. Prieto, R. Lin, M. V. Patel and A. P. Lee, in The 14th International Conference on Miniaturized Systems for Chemistry and Life Sciences (μTAS 2010), Groningen, Netherlands, 2010, pp. 366–368 Search PubMed.
- A. R. Tovar, M. V. Patel and A. P. Lee, Microfluid. Nanofluid., 2011, 10, 1269–1278 CrossRef CAS.
- A. P. Lee, M. V. Patel, A. R. Tovar and Y. Okabe, J. Assoc. Lab. Autom., 2010, 15, 449–454 CrossRef CAS.
- J. Kolb and W. L. Nyborg, J. Acoust. Soc. Am., 1956, 28, 1237–1242 CrossRef.
- W. L. Nyborg, J. Acoust. Soc. Am., 1958, 30, 329–339 CrossRef.
- T. M. Squires and S. R. Quake, Rev. Mod. Phys., 2005, 77, 977 CrossRef CAS.
- P. Marmottant and S. Hilgenfeldt, Nature, 2003, 423, 153–156 CrossRef CAS.
- H. Kim, D. Luo, D. Link, D. A. Weitz, M. Marquez and Z. Cheng, Appl. Phys. Lett., 2007, 91, 133106 CrossRef.
-
T. G. Leighton, The Acoustic Bubble, Academic, San Diego, 1994 Search PubMed.
-
W. T. Coakley and W. L. Nyborg, in Applications in Ultrasound: Its Applications in Medicine and Biology, ed. F. J. Fry, Elsevier, New York, 1978, pp. 77–159 Search PubMed.
Footnote |
† Electronic supplementary information (ESI) available. See DOI: 10.1039/c1lc20626e |
|
This journal is © The Royal Society of Chemistry 2012 |