DOI:
10.1039/C1LC20720B
(Paper)
Lab Chip, 2012,
12, 133-138
Enhancement of DNA hybridization under acoustic streaming with three-piezoelectric-transducer system
Received
4th August 2011
, Accepted 30th September 2011
First published on 10th November 2011
Abstract
Recently, we have demonstrated that DNA hybridization using acoustic streaming induced by two piezoelectric transducers provides higher DNA hybridization efficiency than the conventional method. In this work, we refine acoustic streaming system for DNA hybridization by inserting an additional piezoelectric transducer and redesigning the locations of the transducers. The Comsol® Multiphysics was used to design and simulate the velocity field generated by the piezoelectric agitation. The simulated velocity vector followed a spiral vortex flow field with an average direction outward from the center of the transducers. These vortices caused the lower signal intensity in the middle of the microarray for the two-piezoelectric disk design. On the contrary, the problem almost disappeared in the three-piezoelectric-disk system. The optimum condition for controlling the piezoelectric was obtained from the dye experiments with different activation settings for the transducers. The best setting was to activate the side disks and middle disk alternatively with 1 second activating time and 3 second non-activating time for both sets of transducers. DNA hybridization using microarrays for the malaria parasite Plasmodium falciparum from the optimized process yielded a three-fold enhancement of the signal compared to the conventional method. Moreover, a greater number of spots passed quality control in the optimized device, which could greatly improve biological interpretation of DNA hybridization data.
Introduction
The DNA microarray is one of the most important analysis techniques in molecular biology. This technology is used to measure changes in gene expression levels and to identify patterns of genetic variation i.e.single nucleotide polymorphisms (SNPs).1 In a standard microarray experiment, the sample solution is confined in a microarray hybridization chamber by a cover slip. Inside the chamber, the target DNA diffuses all around the hybridization chamber to hybridize with complementary DNA probes fixed on a solid surface. Without active agitation, the diffusion of macromolecules such as DNA is an extremely slow process owing to the low diffusion constant (D) of DNA, which in water is typically 10−6 to 10−7 cm2 s−1, depending on DNA size, concentration and hybridization conditions.2,3 According to the equation of diffusion length (L): L = (Dt)0.5, the diffusion time (t) for DNA to travel over a distance of a few mm is more than 24 h.4 Practically, diffusion-limited DNA hybridization takes 6–24 h to achieve sufficient hybridization signals and uniformity of signals across an array.5
Accordingly, a large number of researchers have been seeking alternative methods to enhance the hybridization, for example surface acoustic wave (SAW) based microagitation,6 cavitational microstreaming,7 fluid circulation and mixing,1,2,8 rotating the hybridization chamber9,10 and other forms of electrical enhancement.11,12 All of these methods are based on the use of mechanical or electrical force to increase the transportation rate of the DNA molecules. Some of these methods require complicated fabrication processes, expensive devices, or modification of the DNA microarray. Acoustic streaming is another effective mixing technique applicable to microarrays. In this technique, an ultrasound wave passes through a liquid medium and transfers the momentum to the test fluid, generating a steady circular flow occurring in a high-intensity non-linear acoustic field.13 The spatial attenuation of a wave in free space and the friction between a medium and a vibrating object are the two key mechanisms for acoustic streaming induction.14 Another non-linear phenomenon always considered along with acoustic streaming, is the acoustic radiation force. Acoustic radiation force moves a suspending particle toward or away from acoustic pressure nodes while acoustic streaming induces the flow of the entire fluid.13 The extent to which a particle is affected by acoustic radiation force or acoustic streaming depends on the size of the particle. Larger particles will experience a higher degree of acoustic radiation force and a lower level of acoustic streaming, respectively.15 Acoustic streaming has been used in many applications, for example clinical analysis,16 enhancement of heat and mass transfer17 and fluid mixing.3,18 It is one of the most practical fluid mixing techniques for DNA hybridization since there is no need for an external pumping unit or complicated mechanical parts for moving the microarray slide and coverslip. However, fabrication and integration of the acoustic streaming source for microarrays typically requires modification to the microarrays and coverslip,19–21 which is impractical since DNA microarrays are typically obtained from commercial sources available in standard formats only.
Recently, we proposed a simple and low cost device for DNA hybridization based on acoustic streaming induced by two piezoelectric transducers with a coupling fluid.22 Each piezoelectric disk, placed directly beneath each hybridization chamber, generates acoustic waves that are effectively transferred to microarrays via a coupling fluid. The DNA targets were observed to move in both vertical and horizontal directions under the influence of acoustic streaming facilitating hybridization with their complementary DNA probes. This scheme requires no modification of the microarray or coverslip and provides better DNA hybridization efficiency and speed compared to the conventional method of static hybridization. However, the efficiency of DNA hybridization over the whole array area is still sub-optimal. In this work, we refine the acoustic streaming system for DNA hybridization by inserting an additional piezoelectric transducer (PZT) and redesigning the locations of the transducers. The Comsol® Multiphysics software is used to design and simulate the velocity field generated by the piezoelectric agitation. DNA microarray hybridization experiments were performed using Plasmodium falciparum microarrays to verify the performance of the new device.
Experimental section
Design and fabrication
The schematic of the DNA hybridization device is shown in Fig.1. The device consists of three PZTs attached in aluminium chambers. Another aluminium plate, which could be screwed to the chamber, was used as a lid. An o-ring was used to provide a tight seal between chambers and the cover plate. PZTs with a diameter of 1.75 mm were activated at a resonant frequency of 1.67 MHz and operating voltage of 24 Vrms. The 0.8 mm deep circular wells located on top of the PZTs and two rectangular wells at both ends of the chamber were used for holding a coupling fluid and a humidity control solution, respectively. 3xSSC solution was used as a coupling fluid as well as a humidity control solution. The reasons for choosing 3xSSC solution as a coupling fluid were that it is closely matched in acoustic impedance (z) with the silica glass, it is low in heat transfer and it is non-contaminant to the hybridization process.22 The 3-PZT design was proposed in order to improve the non-uniformity problem found from our previous design, in which microarrays were placed on top of two PZTs. It was found that signal intensity in the middle of the hybridization chamber tended to be low compared with other regions, which may have been a result of an outward flow of acoustic wave from the center of the microarray. The new device was designed to have PZTs interposed between microarrays to provide alternate agitations between both sides of the hybridization chamber and thus significantly enhance the movement of target DNA. Moreover, the new design would allow us to operate with different microarray shapes i.e., the short microarray (17.5 × 19.5 mm2) and a long microarray (17.5 × 45 mm2). The optimum signal for each microarray could be obtained by controlling each PZT independently. It should also be noted that no more than three-piezoelectric-transducers can be used in this system because of physical constraints including the sizes of the piezoelectric disk, microarray, glass slide and chamber.
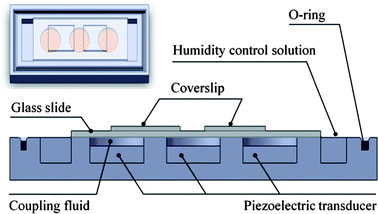 |
| Fig. 1 Schematic showing a side view and top view of dynamic hybridization device in which DNA microarrays are placed inside the hybridization chamber above the piezoelectric ultra sonic transducers and coupling liquid. An O-ring rubber is used to provide a tight seal. | |
Finite element simulation
The microagitation system was designed and simulated using Comsol® Multiphysics (COMSOL Inc., Burlington, MA), a finite element method (FEM) simulation software. The 3D model for numerical simulations was constructed according to the real device dimensions. The finite elements of the structure were then built using a tetragonal mesh with ultra fine precision. The generation and propagation of acoustic waves in the structure were simulated using piezoelectric and pressure acoustic modules in Comsol® Multiphysics. The first set of governing equations used in the piezoelectric module are electromechanical equations of motion with stress-induced piezoelectric effect23: |  | (1) |
where M, Cm, Km, Kme, Ke, u, v, F and L were mass matrix, damping matrix, dielectric conductively matrix, piezoelectric coupling matrix, stiffness matrix, spatial displacement vector, electric displacement vector, structural force vector and nodal charge vector, respectively. In eqn (2) and (3)T, S and E are stress, strain and electric field vectors respectively, while e, cE and εs are anisotropic elastic modulus, piezoelectric constant and dielectric constant matrices, respectively. The electric potential boundary conditions applied between piezoelectric electrodes are 1.67 MHz 130 Vrms sinusoidal waves. The Comsol® program solves the equations in three dimensions and produces the solution of acoustic wave from displacement fields, which is coupled to the pressure acoustic modules where the propagation of acoustic wave is determined by solving Helmholtz's acoustic wave equation15,24: | ∇·(−(1/ρ0)(∇p − q)) − (ω2/c2s + λ2)p/ρ0 = Q, λ = −ikN | (4) |
where p, ρ0, ω, cs, Q, q, λ and kN are acoustic pressure wave, density of acoustic medium, angular frequency, acoustic velocity, monopole source, dipole source, eigenfrequency and normal wave number, respectively. Monopole and dipole source are absent in this case. The mechanical boundary conditions are that all surface of the device are free boundary while the side and top walls of coupling fluid chambers are hard-sound boundary where normal velocity component vanished. The COMSOL eigenvalue solver is used to determine the acoustic frequency response and acoustic wave velocity field. The acoustic simulation of the device with three-PZT agitation was made and compared to that from the previous design that contained only two PZTs.
Optimization of controlling signal
Since dramatic heat flux was generated and could denature DNA during PZT activation, transducers could not operate continuously. Thus, PZTs must be controlled to minimize heat as well as bubble generation, but maximize overall fluidic movement. For the control experiment, the transducers were turned on and off using a square wave signal and tested in three different modes. First, the side disks and the middle disk were activated alternately. Secondly, all disks were activated simultaneously and lastly each disk was activated alternately. Fluidic dye was added into hybridization chambers to observe lateral fluidic movement as a result of agitation. The on and off times for activating the disks were also varied to find an optimum condition. The optimum controlling signal that allowed the fluidic dye to flow all over the hybridization chamber rapidly, homogeneously and coldly are used in the hybridization experiment.
DNA hybridization experiment
The hybridization experiments were then performed using two- and three-piezo hybridization devices and compared with the conventional static method. The DNA microarrays were fabricated on polylysine-coated glass slides using a new generation ultrafast, linear servo driven DeRisi microarrayer, controlled by ArrayMaker software (http://derisilab.ucsf.edu/microarray/software.%20html). The DNA microarray was printed on a glass substrate with a total area of 17.5 × 19.5 mm2. The microarray contained 8088 features with a printed long oligonucleotide probe (70 bases in length). The probes covered the malaria parasite Plasmodium falciparumgenome. The target DNAs were cDNAs, which were transcribed from total RNA.
There were two different cDNA samples, one labeled with Cy3 dye and the other labeled with Cy5 dye (Amersham Biosciences) as described previously.25 The experiments were conducted with the same cDNA quantity (5 pmol) and hybridized for 16 h at 42 °C. After hybridization, the arrays were washed, dried and stored in a cool dark place before analysis. All scanning was done on the same day and all scans were done using the same laser power and PMT gain settings (100%, Cy5 PMT = 560 V, and Cy3 PMT = 490 V respectively). Microarray scanning and image analysis was done on a GenePix400B scanner and spot intensities were quantified using GenePix Pro 6.0 Software (Axon Instrument, Inc). Filtering to distinguish good/effective spots was done using the signal-to-noise ratio (SNR) ≥ 3 reported by GenePixPro, which follows the recommendation made by Yatskou et al.26 for DNA microarray data. Kernel density plots and subarray boxplots were carried out using the R interpreter software.
Results and discussion
Flow field in hybridization chamber
The velocity vector fields from acoustic simulation of the device with 2-PZT and 3-PZT agitation systems are illustrated in Fig. 2. The results of the 2-PZT agitation system are demonstrated when only the left PZT is activated while the results of the 3-PZT agitation system are demonstrated in the mode in which two side PZTs are activated and the center PZT is off. These settings represent typical conditions used in experiments. Comparing the top view of the velocity vector field of 2-PZT and 3-PZT systems (Fig. 2A–B), it is evident that the velocity vector field on the square hybridization area of the 2-PZT system is considerably weaker than that of the 3-PZT system. Thus, agitation by the 3-PZT system is much more effective. From the enlarged 3D view of the velocity vector field of the 2-PZT and 3-PZT systems (Fig. 2C–D), a spiraling vortex 3D streaming flow with a source located approximately in the middle of the PZT is formed in both cases. The spiral flow is in the direction outward from the center of the PZT. For the 2-PZT case, each PZT is concentric with the microarray. Thus, DNA particles will move very slowly (almost stationary) around the center of microarray, however there is much more movement toward the edges. The results can explain our previous experimental observation that the hybridization intensity on the edge of microarrays was often observed to be much greater than on the central region. In the 3-PZT design, each microarray is interposed between two PZTs. Hence, each edge of a microarray can receive the outward spiral flow with strong velocity field from the PZT on each side as shown in Fig. 2D. However, it should be noted that if the spiral fields from PZTs on both sides appear simultaneously, undesirable standing wave will occur from the simulation of two opposing waves. Thus, PZTs on either side should not be activated concurrently. Therefore, the 3-PZT design with alternate PZT activation should be able to circumvent the problem of low hybridization intensity at the microarray center in 2-PZT design.
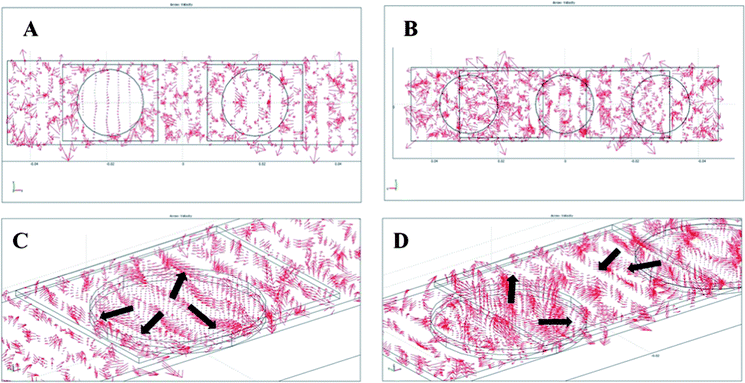 |
| Fig. 2 Top view of velocity vector field of (A) the 2-PZT system when the left PZT is activated and (B) the 3-PZT system when two side PZTs are activated. The corresponding 3D views of the velocity vector field of the (C) 2-PZT and (D) 3-PZT systems. | |
Operating signal optimization
From the control experiments, three PZTs were initially tested in three different modes without non-activating time between consecutive transducer activations. It was found that the fluidic dye could cover the entire hybridization chamber within 5 min when the side disks and the middle disk were alternately activated. However, similar coverage would take 10 min and 14 min when operating all three PZTs simultaneously and activating each PZT alternately, respectively. Thus, the results agree with the prediction from the simulation that a standing wave would occur and deter fluid mixing when operating all PZTs simultaneously. Therefore, the best activation mode is confirmed to be operating the middle and side PZTs alternately. However, it was found that excessive heat was generated due to the lack of non-activating time between consecutive transducer activations. Thus, non-activating time was added and on-off activating signals were varied to optimize fluidic movement, total operating time and heat generation. Lower heat generation could be observed with less bubble generation in the fluid after adding non-activating time. The optimum activating and non-activating times were found to be 1 and 3 s, respectively. The condition was proven to be suitable in terms of heat reduction and overall fluid movement. The optimum agitation by 3-PZT system allowed the fluidic dye to fill the entire chamber as illustrated in Fig. 3B within 15 min, while it took more than 30 min for the dye to cover half of the chamber as shown in Fig. 3A for the static case (all PZTs off).
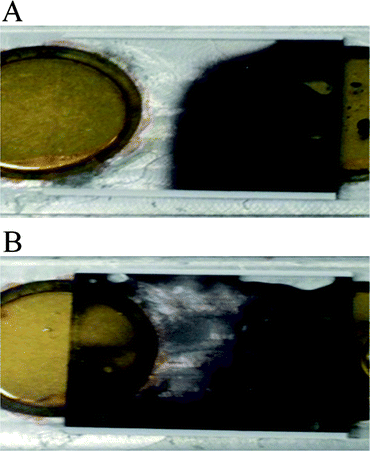 |
| Fig. 3 Photographs of dye solution mixing in the hybridization microchamber (A) without acoustic streaming (PZT off) and (B) with the PZT agitation at 1.67 MHz and 24 Vrms. | |
Enhancement of microarray hybridization efficiency
The hybridization efficiency of our device was compared with the conventional static hybridization method. The hybridization experiments were performed on a microarray with corresponding biological cDNA samples. The fluorescent scanned images of microarrays hybridized with or without piezoelectric agitation, are shown in Fig. 4. The signals are consistent across both arrays, although the signal overall for the piezoelectric hybridization appears stronger relative to conventional hybridization. For microarray data analysis, spot quality control is a crucial part to distinguish between good/effective spots from bad ones that may represent biologically irrelevant noise. We found that more spots pass filtering for agitated 2-PZT and 3-PZT relative to conventional hybridization (2720, 5560, and 2414 spots respectively). Thus, our 3-PZT system can significantly increase the number of effective spots over the previous 2-PZT system and static hybridization. As a result, our new agitation system could increase the detection sensitivity for DNA targets that may have produced low hybridization signals (due to their low expression level) but were biologically relevant. The power of discovering more effective spots would be extremely beneficial in many kinds of transcriptomic profiling studies because it would produce better microarray interpretation. The greater number of good spots for agitated hybridization cover a broader range of signal intensities, especially for the 3-PZT hybridization, as shown by the kernel density distribution plots for the Cy3 and Cy5 channels (Fig. 5A).
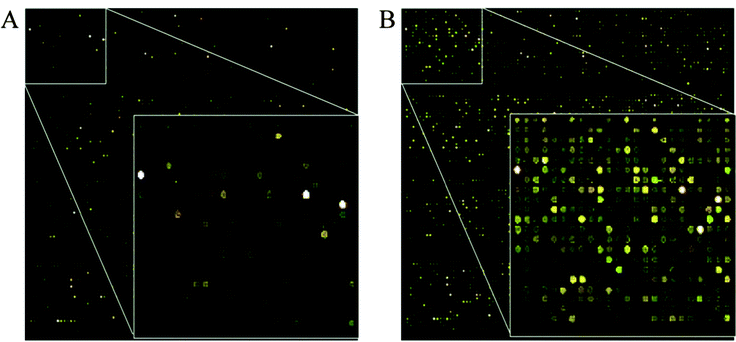 |
| Fig. 4 Microarray scan pictures of P. falciparumDNA microarrays with (A) 16 h static hybridization, and (B) 16 h 3-PZT dynamic hybridization. | |
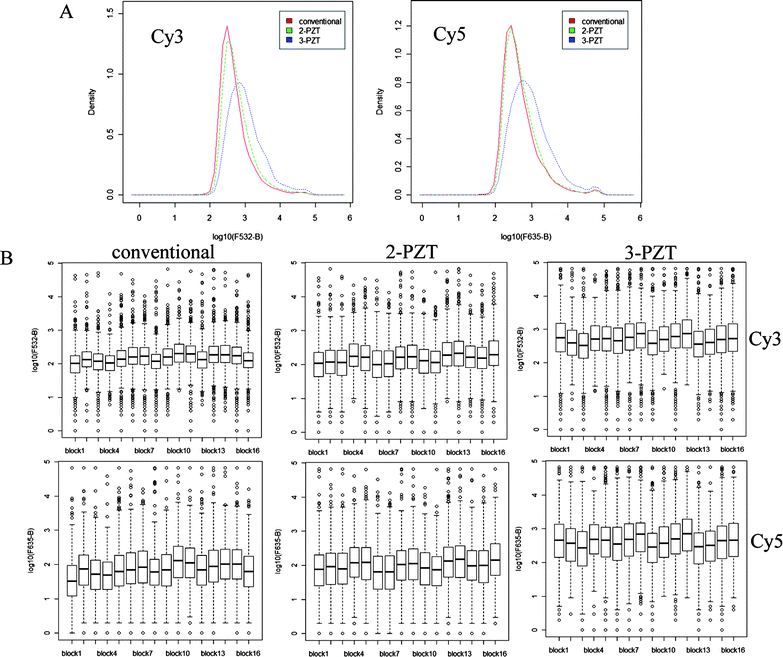 |
| Fig. 5 (A) Kernel density plots (normalized density vs. background-corrected fluorescence intensity (log scale) for the spots in Cy3 (532 nm) and Cy5 (635 nm) channels) obtained from hybridization experiments using conventional, 2-PZT and 3-PZT systems and (B) corresponding subarray box plots (background-corrected fluorescence intensity (log scale) distribution for 16 subarrays). Thick line in each box represents median intensity. | |
In order to demonstrate that the hydridization efficiency of the 3-PZT is consistent across the array, the fluorescent signals within each of the 16 subarrays were compared. The boxplots of fluorescent intensity shown in Fig. 5B indicate that the ranges of intensities within each subarray are comparable, with no noticeable bias in different regions of the array. A rigorous statistical analysis was not performed though, since the subarrays contain different probes corresponding to genes expressed at different levels. Moreover, the printing of probes on the array is not random e.g. the bottom row of each subarray is mostly empty. Consistent hybridization across the array also requires careful application of the labeled DNA solution and coverslip, which can be a major source of operator error. To overcome this problem, semi-automated delivery systems could be incorporated into the 3-PZT device, such as used in the MAUI commercial system (BioMicro systems USA).
Conclusions
In conclusion, we have developed a new three-PZT design for a low-cost dynamic acoustic streaming-based DNA hybridization system. The new acoustic streaming design is modified from the previously developed 2-PZT system by inserting an additional piezoelectric transducer and redesigning the locations of the transducers. The design is assisted by acoustic simulations using Comsol® Multiphysics. Our static versus dynamic hybridization comparison under ‘real’ microarray experimental parameters demonstrated that the newly designed dynamic hybridization system provides significantly higher average fluorescent signal intensities in both Cy3 and Cy5 channels than the conventional static hybridization and the previous 2-PZT design. This allows more effective spots to be included in the subsequent microarray data analysis, and potentially greater power to detect biologically significant signals, especially those in the lower range.
Acknowledgements
We would like to thank Drs Joseph Derisi and Jennifer Shock at the University of San Francisco for providing the oligo-set, training and facilities for the production of DNA microarray slides, through generous support from the Howard Hughes Medical Institute (HHMI, USA). S.K. is an international research scholar of HHMI, USA. A.T. expresses his great gratitude to the Thailand Research Fund (RSA5380005) for a research career development grant.
Notes and references
- H. H. Lee, J. Smoot, Z. McMurray, D. A. Stahl and P. Yager, Lab Chip, 2006, 6, 1163–1170 RSC.
- M. K. McQuain, K. Seale, J. Peek, T. S. Fisher, S. Levy, M. A. Stremler and F. R. Haselton, Anal. Biochem., 2004, 325, 215–226 CrossRef CAS.
- R. H. Liu, J. Yang, M. Z. Pindera, M. Athavale and P. Grodzinski, Lab Chip, 2002, 2, 151–157 RSC.
- J.-M. Hertzsch, R. Sturman and S. Wiggins, Small, 2007, 3, 202–218 CrossRef CAS.
-
M. Schena, Microarray Biochip Technology, Eaton Publishing Company, California, 2000 Search PubMed.
- A. Toegl, R. Kirchner, C. Gauer and A. Wixforth, J Biomol Tech., 2003, 14, 197–204 Search PubMed.
- R. H. Liu, R. Lenigk, R. L. Druyor-Sanchez, J. Yang and P. Grodzinski, Anal. Chem., 2003, 75, 1911–1917 CrossRef CAS.
- P. K. Yuen, G. Li, Y. Bao and U. R. Muller, Lab Chip, 2003, 3, 46–50 RSC.
- J. Vanderhoeven, K. Pappaert, B. Dutta, P. Van Hummelen and G. Desmet, Anal. Chem., 2005, 77, 4474–4480 CrossRef CAS.
- M. A. Bynum and G. B. Gordon, Anal. Chem., 2004, 76, 7039–7044 CrossRef CAS.
- C. Gurtner, E. Tu, N. Jamshidi, R. W. Haigis, T. J. Onofrey, C. F. Edman, R. Sosnowski, B. Wallace and M. J. Heller, Electrophoresis, 2002, 23, 1543–1550 CrossRef CAS.
-
C. Chii-Chang, K. Wei-Chu, C. Sung-Kay and T. Chi-Meng, Enhancement of DNA hybridization efficiency using photovoltaic effect, 2003 Search PubMed.
-
T. Uchida, T. Suzuki and S. Shiokawa, Investigation of acoustic streaming excited by surface acoustic waves, 1995 Search PubMed.
-
C. P. Lee and T. G. Wang, Outer acoustic streaming, ASA, 1990 Search PubMed.
- S. M. Hagsater, T. G. Jensen, H. Bruus and J. P. Kutter, Lab Chip, 2007, 7, 1336–1344 RSC.
- X. Shi, R. W. Martin, S. Vaezy and L. A. Crum, J. Acoust. Soc. Am., 2002, 111, 1110–1121 CrossRef.
-
E. H. Trinh and J. L. Robey, Experimental study of streaming flows associated with ultrasonic levitators, AIP, 1994 Search PubMed.
- C. Suri, K. Takenaka, H. Yanagida, Y. Kojima and K. Koyama, Ultrasonics, 2002, 40, 393–396 CrossRef CAS.
- Z. Guttenberg, H. Muller, H. Habermuller, A. Geisbauer, J. Pipper, J. Felbel, M. Kielpinski, J. Scriba and A. Wixforth, Lab Chip, 2005, 5, 308–317 RSC.
- A. Wixforth, C. Strobl, C. Gauer, A. Toegl, J. Scriba and Z.v. Guttenberg, Anal. Bioanal. Chem., 2004, 379, 982–991 CrossRef CAS.
- Z. Pan, Y. Li, D. Zhou, J. Tang, M. Zhang, P. Xiao and Z. Lu, Anal. Biochem., 2008, 376, 280–282 CrossRef CAS.
- K. Rodaree, T. Maturos, S. Chaotheing, T. Pogfay, N. Suwanakitti, C. Wongsombat, K. Jaruwongrungsee, A. Wisitsoraat, S. Kamchonwongpaisan, T. Lomas and A. Tuantranont, Lab Chip, 2011, 11, 1059–1064 RSC.
- F. Lu, H. P. Lee, P. Lu and S. P. Lim, Sens. Actuators, A, 2005, 119, 90–99 CrossRef.
- S. Mohamady, R. K. Raja Ahmad, A. Montazeri, R. Zahari and N. A. Abdul Jalil, Advances in Acoustics and Vibration, 2009, 2009, 1–10 CrossRef.
- Z. Bozdech, J. Zhu, M. Joachimiak, F. Cohen, B. Pulliam and J. DeRisi, GenomeBiology, 2003, 4, R9 CrossRef.
- M. Yatskou, E. Novikov, G. Vetter, A. Muller, E. Barillot, L. Vallar and E. Friederich, BMC Res. Notes, 2008, 1, 80 CrossRef.
|
This journal is © The Royal Society of Chemistry 2012 |
Click here to see how this site uses Cookies. View our privacy policy here.