DOI:
10.1039/C1FO10129C
(Paper)
Food Funct., 2012,
3, 58-66
Impact of dietary fiber coatings on behavior of protein-stabilized lipid droplets under simulated gastrointestinal conditions
Received
24th June 2011
, Accepted 15th October 2011
First published on 16th November 2011
Abstract
Multilayer emulsions containing lipid droplets coated by lactoferrin (LF) - anionic polysaccharide layers have improved resistance to environmental stresses (such as pH, salt, and temperature), but their behavior within the gastrointestinal tract (GIT) is currently unknown. The objective of this research was therefore to monitor changes in the physicochemical properties and digestibility of these systems under simulated GIT conditions. Primary emulsions (5% corn oil, 0.5% LF) were prepared using a high-pressure homogenizer. Secondary emulsions (5% corn oil, 0.5% LF, 0.5% polysaccharide) were prepared by incorporating alginate, low methoxyl pectin (LMP) or high methoxyl pectin (HMP) into primary emulsions. Emulsions were then subjected to simulated gastric fluid (SGF) and simulated intestinal fluid (SIF) conditions in sequence. LF, LF-LMP and LF-HMP emulsions were stable to droplet aggregation in the stomach but aggregated in the small intestine, whereas LF-alginate emulsions aggregated in both the stomach and small intestine. The presence of a dietary fiber coating around the initial lipid droplets had little influence on the total extent of lipid digestion in SIF, but LF-alginate emulsions had a slower initial digestion rate than the other emulsions. These results suggest that the dietary fiber coatings may become detached in the small intestine, or that they were permeable to digestive enzymes. Pepsin was found to have little influence on the physical stability or digestibility of the emulsions. The knowledge obtained from this study is important for the design of delivery systems for encapsulation and release of lipophilic bioactive ingredients.
1. Introduction
Colloid delivery systems are being used increasingly in the food industry to encapsulate, protect and deliver lipophilic bioactive components, such as ω-3 fatty acids, carotenoids, oil-soluble vitamins, and phytosterols.1–7 An electrostatic layer-by-layer (LbL) deposition methodology has been shown to have particular promise for the development of colloidal delivery systems for this purpose.8–10 This approach relies on electrostatic deposition of ionic polymers onto the surfaces of oppositely charged colloidal particles, leading to the formation of a core-shell structure consisting of a particle core surrounded by a polymer shell.8,9,11,12Food-grade multilayer emulsions can be produced based on this principle using a multiple-step process.8 A “primary” emulsion containing lipid droplets coated with a layer of ionic emulsifier molecules is formed using conventional homogenization procedures. A “secondary” emulsion is then formed by electrostatically depositing an oppositely charged biopolymer onto the charged lipid droplet surfaces. This latter procedure can be repeated a number of times using two or more ionic biopolymers to fabricate droplets with multilayer biopolymer coatings.8,13 The successful utilization of this approach requires careful control of system composition and preparation conditions so as to avoid droplet flocculation due to bridging and depletion mechanisms.8,11,12
Numerous studies have shown that the multilayer approach can be used to improve the stability of oil-in-water emulsions to environmental stresses, such as pH, ionic strength, thermal processing, freezing, and dehydration.8,9,14,15Biopolymer coatings may also be used to improve the chemical stability of encapsulated components so as to extend the shelf life of functional food components, e.g., to retard oxidation of polyunsaturated lipids.16–18 Recent studies suggest that biopolymer coatings may also alter the fate of lipid droplets within the gastrointestinal tract (GIT), e.g.digestion, release, and absorption.17,19–21 The influence of biopolymer coatings on the fate of lipophilic components within the GIT may have either beneficial or detrimental consequences. If a biopolymer coating reduces the release or absorption of a lipophilic component within the GIT, then it may reduce its overall bioactivity. Conversely, the ability to delay the digestion of lipids within the GIT may have advantages for the development of some functional food applications, such as targeted release of bioactive components to specific regions of the GIT (such as the colon) or for inducing satiety through the ileal brake mechanism.22,23
In this study, we focused on the influence of biopolymer coatings comprised of dietary fibers on the fate of multilayer emulsions within a simulated GIT. Bovine lactoferrin (LF) was used as an emulsifier to prepare primary emulsions because it is a surface-active globular protein with a relatively high isoelectric point (pI ≈ 8.7), which means that it has a positive charge over a wide pH range.24,25 Three indigestible anionic polysaccharides were used to form secondary emulsions containing LF-stabilized lipid droplets coated by dietary fibers: alginate; low methoxyl pectin (LMP); high methoxyl pectin (HMP). We selected these polysaccharides because they have different molecular characteristics.26 LMP and HMP have a linear anionic backbone with neutral side branches, whereas alginate has a linear anionic backbone with no branches.26 The linear charge density of the anionic polysaccharides decreases in the following order: alginate > LMP > HMP.27 We hypothesize that the molecular structure of the polysaccharides coating the lipid droplets would influence their stability and digestibility under simulated GIT conditions.
A number of previous studies have shown that the presence of a biopolymer coating may alter the rate and extent of lipid digestion in multilayer emulsions using in vitrodigestion models.17,19–21 However, previous studies have used either a simulated gastric model or a simulated small intestine model. In this study, we subjected the multilayer emulsions to simulated gastric and small intestine models in sequence so as to more closely mimic actual GIT conditions. In addition, the protein used to coat the lipid droplets (lactoferrin) had a considerably higher isoelectric point than those used in most other studies (β-lactoglobulin), which means anionic polysaccharides may associate with lipid droplet surfaces over a wider pH range. In particular, it should be possible for anionic dietary fibers to adhere to lactoferrin-coated lipid droplets under the neutral conditions that occur in the small intestine, where most of the lipid digestion normally occurs. The results from this study should provide useful information for the design of emulsion-based delivery systems to encapsulate lipophilic bioactive ingredients and control lipid digestibility. This may, in turn, be useful for developing functional foods to induce satiety or control the release of bioactive components within the GIT.
2. Materials and methods
2.1. Materials
Corn oil was purchased from a commercial food supplier (Mazola, ACH Food Companies, Inc., Memphis, TN) and stored at 4 °C until use. The manufacturer reported that the corn oil contained approximately 14, 29, and 57 wt% of saturated, monounsaturated, and polyunsaturated fats, respectively. Food grade lactoferrin (Lot 10373317) was supplied by FrieslandCampina Domo (Delhi, NY), and the manufacturer reported that it contained 97.7% protein and 0.12% ash. Food grade alginic acid (sodium salt) was obtained from Sigma-Aldrich (Sigma Chemical Co., St. Louis, MO). Low methoxyl pectin (LMP) [DE<30%] and high methoxyl pectin (HMP) [DE>50%] were obtained from CP Kelco (Atlanta, GA). Pepsin from porcine gastric mucosa, porcine bile extract, type II porcine pancreatic lipase, sodium chloride (NaCl), calcium chloride (CaCl2), monobasic phosphate and dibasic phosphate were purchased from Sigma-Aldrich (Sigma Chemical Co., St. Louis, MO) or Fisher Scientific (Pittsburgh, PA). All solvents and reagents were of analytical grade.
2.2. Emulsion preparation
Primary emulsions.
An aqueous emulsifier solution (1% w/w) was prepared by dissolving powdered LF in 10 mM phosphate buffer solution (pH 7) and stirring for at least 3 h. The LF solution was then filtered to remove any insoluble particles. Corn oil was added to the filtered LF solution so that the final system contained: 10% (w/w) oil; 1% (w/w) LF; 89% (w/w) buffer solution. This mixture was coarsely homogenized for 2 min using a hand blender (Tissue Tearor, model 985379-395, Biospec Products Inc.) and then passed 3 times through a high pressure homogenizer (Microfluidizer M-110 L processor, Microfluidics Inc., Newton, MA) operating at 11,000 psi (75.8 MPa).
Secondary emulsions.
Three types of secondary emulsions were produced by mixing the primary emulsion with alginate, low methoxyl pectin (LMP), or high methoxyl pectin (HMP) solutions. For each polysaccharide type, a 1% (w/w) solution was prepared by dissolving the powdered ingredient into 10 mM of phosphate buffer solution (pH 7), and stirring for 2 to 3 h to ensure dissolution. An equal amount of primary emulsion was then added to each polysaccharide solution, and the mixture was vortexed and stored overnight at 20 °C prior to in vitrodigestion.
2.3. Emulsion characterization
The physical properties and stability of the emulsion and digesta samples were monitored by measuring their particle size distribution, charge and microstructure.
Particle size distributions were measured using static light scattering by injecting diluted emulsion samples into an optical measurement cell and measuring the angular scattering pattern (Malvern Mastersizer 2000, Malvern Instruments, Worcestershire, U.K.). Emulsion samples were diluted in 10 mM phosphate buffer at the appropriate pH prior to analysis to avoid multiple scattering effects. Background corrections and system alignment were performed prior to each measurement when the measurement cell was filled with the appropriate buffer solution. Particle sizes are reported as the volume-averaged mean diameters (d43) calculated from the particle size distribution.
The electrical charge (ζ-potential) of the particles was determined using electrophoretic mobility measurements on diluted emulsion samples (Zetasizer Nano ZS series, Malvern Instruments, Worcestershire, U.K.). Emulsion samples were diluted in 10 mM phosphate buffer at the appropriate pH at a ratio of 1
:
200 (v/v) and then placed in a capillary test tube that was loaded into the instrument. Samples were equilibrated for 1 min inside the instrument before data were collected over at least 10 sequential readings and processed using the Smoluchowski model.
Emulsion microstructures were assessed by optical microscopy using a Nikon D-Eclipse C1 80i microscope (Nikon, Melville, NY). Photographs of the emulsion and digesta samples were taken over a period of 3 days using a digital camera to illustrate macroscopic instability.
2.4. Simulated gastrointestinal tract
Each emulsion sample was passed through a two-step simulated GIT model that consisted of a gastric phase and a small intestine phase.
In vitro gastric phase.
Simulated gastric fluid (SGF) containing 2 g of NaCl and 7 mL of HCl, with or without the addition of 3.2 g of pepsin, diluted to 1 L and with pH adjusted to 1.2 using 1.0 M HCl was prepared (United States Pharmacopeial Convention, 2000). The in vitro gastric model consisted of a conical flask (250 mL) containing SGF maintained at 37 °C with continuous shaking at 100 rev min¬1 in a temperature controlled water bath (Innova Incubator Shaker, Model 4080, New Brunswick Scientific, New Jersey, USA) to mimic the conditions in the stomach.28 The pH and the temperature were continuously monitored and controlled. Each stock emulsion was mixed with SGF (ratio 1
:
1 w/w). The final mixture, which contained 2.5% (w/w) oil, was adjusted to pH 2.5 using 1 M NaOH and then incubated at 37 °C for up to 2 h in the in vitro gastric model. Samples were taken for characterization at the beginning and end of the incubation period. We did not include gastric lipase in the SGF used in the current study, which may have influenced the behavior of the delivery systems in the simulated GI tract used, since some lipid hydrolysis is known to occur within the human stomach. It would be advantageous to systematically examine the influence of gastric lipase in future studies.
In vitro intestinal phase.
The digestion of ingested lipids mainly occurs in the small intestine due to the presence of pancreatic lipases. Thus, the in vitrodigestion of the emulsified lipids from the gastric phase was monitored using a simulated intestinal phase described previously.29–34 Digesta samples (30 mL) obtained from the simulated gastric model were incubated for 2 h at 37 °C in a simulated small intestinal fluid (SIF) containing 2.5 mL pancreatic lipase (4.8 mg mL−1), 4 mL bile extract solution (5 mg mL−1) and 1 mL calcium chloride solution (750 mM), and the free fatty acids (FFA) released were monitored by determining the amount of 0.25 M NaOH needed to maintain a constant pH of 7.0 within the reaction chamber.30 This was achieved using an auto-titration unit (Metrohm USA, Inc.) controlled by dedicated software (Tiamo 1.2.1 software, Metrohm GA, Switzerland). All additives were individually dissolved in phosphate buffer (10 mM, pH 7.0) before use. Lipase addition and initialization of the titration program were done only after the addition of all other predissolved ingredients and careful balancing of the pH to 7.0. Samples were taken for physicochemical and structural characterization after 2 h of digestion. We did not explicitly include co-lipase in the SIF used in this study, but the crude lipase extract used is likely to contain co-lipase (since we found that our system was still able to catalyze the lipid digestion reaction relatively rapidly).
It should be noted that this relatively simple in vitrodigestion model does not accurately simulate the complex physiochemical and physiological conditions that a food would experience within the human gastrointestinal tract. For example, it does not mimic the complex forces and flow profiles that foods experience as they pass through the GI tract, and it does not include all of the biological components that might influence digestion (such as gastric lipase and pancreatic co-lipase). Nevertheless, the relatively simple digestion model used in this study is still useful for rapidly screening different delivery system formulations under standardized conditions.35 Promising candidates can then be investigated using more sophisticated in vitro or in vivodigestion models.
2.5. Experimental design
All experiments were carried out at least in duplicates using freshly prepared samples, and the results are presented as the calculated mean and standard deviation.
3. Results and discussion
3.1. Influence of interfacial properties
Our working hypothesis was that the presence of a dietary fiber coating around lactoferrin-stabilized lipid droplets would influence their behavior within a simulated gastrointestinal tract (GIT). The composition and properties of dietary fiber coatings might be expected to influence the fate of lipid droplets within the GIT for a number of reasons.22,23,36,37 First, the physicochemical characteristics of the coating (e.g. charge, thickness, polarity, and packing) influence the sign, magnitude and range of the colloidal interactions operating between lipid droplets, such as van der Waals, electrostatic, steric, and hydrophobic forces.38 The relative strength of the attractive and repulsive interactions in a particular environment determines the stability of lipid droplets to flocculation and coalescence, and hence to gravitational separation.38Lipid droplet stability within the GIT has important implications for the biological fate of nutrients, as well as the response of the human body to nutrient intake. The ability of digestive enzymes (e.g., lipases and proteases) to access emulsified lipids and proteins adsorbed to their surfaces may be influenced by the droplet aggregation state, e.g., lipid droplets trapped within the interior of a floc may be more difficult to access than free droplets. The stability of emulsified lipids to gravitational separation within the stomach can modulate the satiety response (feeling of fullness), since this influences the rate that calories pass through the pyloric sphincter and into the small intestine, which influences the rate of gastric emptying through a feedback mechanism.39–41 Second, the type, structural organization, and interactions of the molecules present at the oil–water interface will influence how the various molecular species within the GIT responsible for digestion and absorption interact with the emulsified lipid and adsorbed protein, e.g., bile salts, phospholipids, and digestive enzymes.13,36,42 In principle, a dietary fiber coating that is impermeable, remains at the droplet surface, and is resistant to digestion may inhibit the ability of digestive enzymes to reach the proteins or lipids below it.37 Third, the composition of the interface determines the electrical characteristics of the droplet surfaces, which influences how they interact with other charged species in the system, e.g., mineral ions, ionic biopolymers, and biological surfaces.38 This may be important for the design of nutraceutical delivery systems that remain in the GIT for extended periods due to mucoadhesion (adhesion to intestinal walls), thereby providing a longer time to release encapsulated components at the site of action.43
Emulsion samples with different interfacial coatings were prepared at pH 5 and then passed through a simulated GIT consisting of a simulated gastric fluid (SGF, pH 2.5) and a simulated intestinal fluid (SIF, pH 7). The mean particle diameter, particle charge, microstructure, and appearance of the emulsions were measured after each stage (Fig. 1 to 3). The behavior of the lipid droplets within the simulated GIT clearly depended on the nature of the interfacial coating.
3.1.1. Primary emulsions.
The primary emulsion had a monomodal particle size distribution and a relatively small mean particle diameter (d43 = 0.57 μm) after preparation at pH 5 (Fig. 1), indicating that it was stable to particle aggregation, which can be attributed to a combination of electrostatic and steric repulsion. The lipid droplets had a fairly high positive charge (ζ ≈ +17 mV) at pH 5 because the adsorbed LF layer was well below its isoelectric point (pI ≈ 8.7),44 which would generate an electrostatic repulsion between the droplets (Fig. 2).15,45 In addition, LF molecules are relatively large (≈80 kDa) and have some carbohydrate side-groups that protrude into the aqueous phase,44 which would generate a steric repulsion between the droplets.15,45 The mean droplet size remained small after the primary emulsion was incubated in SGF (pH 2.5, 2 h) (Fig. 1a), which can be attributed to the increased positive charge on the droplets (Fig. 2) leading to a stronger electrostatic repulsion. The droplet charge became highly negative after they were incubated in SIF (pH 7, 2 h). One would have expected the electrical charge on LF-coated lipid droplets to be slightly positive at neutral pH due to the relatively high isoelectric point of this globular protein: pI ≈ pH 8.7.44 This result suggests that there was a substantial change in interfacial composition and/or structure in the primary emulsion under simulated digestion conditions. There are a number of potential reasons for this change: adsorption of anionic species (such as free fatty acids, phospholipids, bile salts or lipase) on top of the LF layer; displacement of the LF layer from the lipid droplet surfaces by surface-active anionic species (such as free fatty acids, phospholipids, bile salts or lipase); or adsorption of small negatively charged ions from the surrounding aqueous phase (such as anions from buffers or salts). It is not possible to discriminate between these possibilities using ξ-potential measurements alone, and more detailed analytical techniques would be needed to establish the relative importance of these different physicochemical mechanisms.
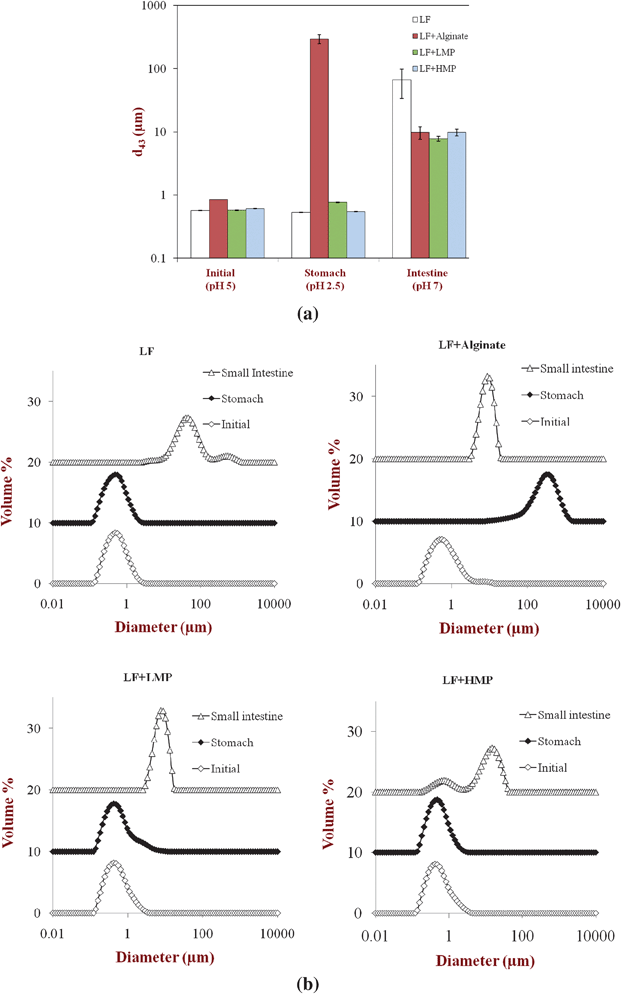 |
| Fig. 1 (a): Effect of simulated gastrointestinal tract conditions on the mean particle diameters (d43) of primary emulsion (LF-coated) and secondary emulsions (LF-alginate, LF-LMP and LF-HMP coated). Note: The SGF contained no pepsin. (b): Effect of simulated gastrointestinal tract conditions on the particle size distribution of the primary emulsion (LF-coated) and the secondary emulsions (LF-alginate, LF-LMP, LF-HMP coated). Note: The SGF contained no pepsin. | |
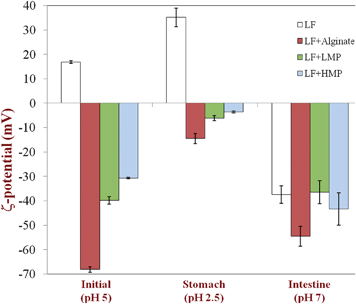 |
| Fig. 2 Effect of simulated gastrointestinal tract conditions on the particle charge (ζ-potential) of primary emulsion (LF-coated) and secondary emulsions (LF-alginate, LF-LMP and LF-HMP coated). Note: The SGF contained no pepsin. | |
3.1.2. Secondary emulsions.
The secondary emulsions containing different kinds of anionic dietary fibers (alginate, HM-pectin, LM-pectin) prepared at pH 5 all had high negative charges (Fig. 2), suggesting that the dietary fibers had adsorbed onto the cationic LF-stabilized lipid droplets. The magnitude of the negative charge on the droplets decreased in the following order: alginate (−68 mV) > LM-pectin (−40 mV) > HM-pectin (−31 mV), which is consistent with the decreasing linear charge density of these polysaccharides.27 All the secondary emulsions had monomodal particle size distributions and a relatively small mean particle diameter (d43 < 1 μm) after preparation at pH 5, indicating that they were relatively stable to particle aggregation (Fig. 1). The good stability of these emulsions can be attributed to a combination of electrostatic repulsion (associated with their high negative charge) and steric repulsion (associated with the thick interfacial layer).8,46 When the secondary emulsions were incubated in simulated gastric fluid (pH 2.5) there was a pronounced decrease in the magnitude of their negative charge (Fig. 2). This result suggests that the anionic polysaccharides remained at least partly bound to the LF-coated lipid droplets under SGF conditions, since the LF-coated droplets alone were highly positive under the same conditions. The charge on the LF-alginate coated droplets was more negative than that on the LF-HMP or LF-LMP coated droplets, which may have been because alginate has a higher linear charge density than either pectin.47 When the secondary emulsions were incubated in small intestinal fluid (pH 7) they all became highly negatively charged and have ζ-potentials that were different from those in the LF-coated droplets alone (Fig. 2), which indicates that there was some change in interfacial composition. As mentioned earlier, it is not possible to ascertain the interfacial composition of the lipid droplets from ζ-potential measurements alone since many anionic components could have contributed to the measured negative charge, e.g., polysaccharides, bile salts, phospholipids, and free fatty acids.
With the exception of the secondary LF-alginate emulsions, all the other emulsions were relatively stable to aggregation in simulated gastric fluid conditions, i.e., no large increase in mean particle diameter (Fig. 1), no evidence of extensive flocculation or coalescence in microscopy images (Fig. 3), and no evidence of visible creaming (Fig. 4). Conversely, the LF-alginate emulsions were highly unstable in SGF conditions, exhibiting a large increase in particle diameter (Fig. 1), extensive flocculation (Fig. 3), and visible evidence of creaming (Fig. 4). The differences in the aggregation stability of the emulsions under acidic conditions can be related to differences in their interfacial characteristics. LF-coated lipid droplets are highly positive at acidic pH (Fig. 2), which will lead to a strong electrostatic repulsion between them.48Pectin molecules have a significant amount of neutral side branches along their anionic backbone,49 which can generate steric repulsion between pectin-protein-coated lipid droplets, thereby improving their stability to aggregation.46 On the other hand, alginate molecules have a linear anionic chain with no neutral side branches,26 and so they may be more prone to droplet aggregation through charge neutralization and bridging effects since there is little steric repulsion contribution.50 The relatively high ionic strength in the SGF will have led to appreciable electrostatic screening, thereby promoting flocculation of predominantly electrostatically-stabilized droplets.23 On exposure to SIF, the primary emulsion and all secondary emulsions exhibited extensive flocculation, coalescence, and creaming. At pH 7, the LF-coated droplets had a negative charge (Fig. 2) and so the anionic polysaccharides may not have been strongly attracted to their surfaces. Consequently, the lipase molecules would be able to access and hydrolyze the emulsified lipids leading to the production of free fatty acids. In addition, the bile salts and phospholipids may have been able to displace at least some of the protein layer from the lipid droplet surface.51 These changes in interfacial composition and droplet-droplet interactions may have made the emulsion less stable to droplet aggregation. In addition, the majority of the triacylglycerols within the lipid phase will have been converted into free fatty acids and monoacylglycerols due to the action of the lipase, which could also lead to emulsion instability.
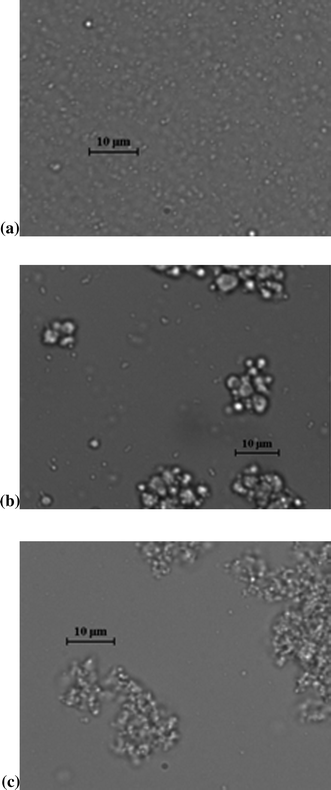 |
| Fig. 3 (a): Microscopic image of a stable primary LF emulsion taken after incubation in SGF (pH 2.5). This kind of image was seen for all of the emulsions after incubation in the stomach step of the simulated GI tract, with the exception of the secondary LF-alginate emulsion (see Fig. 3c). (b): Microscopic image of a coalesced secondary LF-pectin emulsion sample taken after incubation in the SIF (pH 7). This kind of image was seen for all the emulsions after incubation in the intestinal step of the simulated GI tract. (c): Microscopic image of a flocculated LF-alginate emulsion sample taken after incubation in SGF (pH 2.5). This was the only emulsion that aggregated in the gastric phase of the simulated GIT. | |
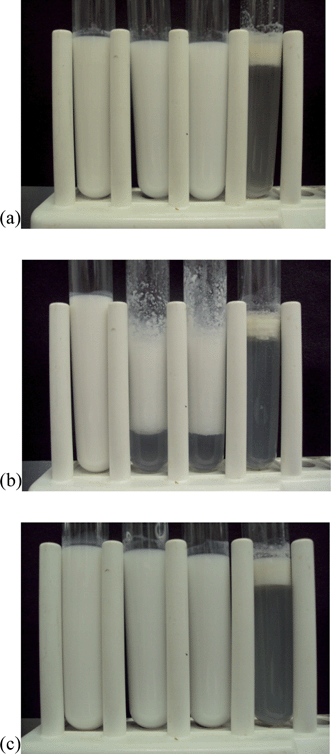 |
| Fig. 4 Photographs of emulsion samples passed throughout a simulated GIT model containing no pepsin. Emulsion type: (a) LF-coated droplets; (b) LF-alginate-coated droplets; (c) LF-pectin-coated droplets (HM-pectin and LM-pectin showed similar behavior). From left-to-right: initial emulsion (pH 5); beginning of stomach phase (pH 2.5); end of stomach phase (pH 2.5); end of small intestine phase (pH 7). | |
3.1.3.
Lipase digestibility.
Finally, we examined the influence of the dietary fiber coating on the lipase digestibility of the lipid droplets under simulated small intestinal conditions using a pH-stat method (Fig. 5). In general, the percentage of free fatty acids released from the emulsions increased steeply during the first 30 min of digestion, and then reached a relatively constant value at longer digestion times. At the end of the digestion period (2 h), the overall extent of lipid digestion was fairly similar for all emulsions, with about 56% of the free fatty acids being released, suggesting that dietary fiber coatings did not prevent lipid digestion. Nevertheless, there were some differences in the initial rate of lipid digestion of the secondary emulsions (Fig. 5), with the alginate-coated droplets being digested at a slower initial rate than the pectin-coated droplets. Previous researchers have also found that alginate may retard lipid digestion in simulated intestinal conditions,52,53 where it was attributed to its ability to either bind calcium ions (which are needed to remove free fatty acids from the droplet surfaces by forming a precipitate) or to form a gel around the lipid droplets (which would slow down the diffusion of reactants, catalysts and products involved in the digestion process). These results suggest that dietary fiber coatings may be able to control the initial rate of lipid digestion within a simulated GIT, but that eventually the encapsulated lipid will be digested and released, which is important information for the design of functional foods.
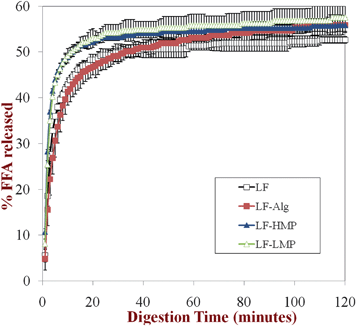 |
| Fig. 5
Lipid digestibility of the primary emulsion (LF-coated) and secondary emulsions (LF-alginate, LF-LMP and LF-HMP coated) in simulated small intestine conditions (SIF) measured using a pH-stat method (pH 7). Prior to testing, the emulsions had been incubated in SGF containing no pepsin. | |
3.2. Influence of pepsin
Gastric pepsin is a protease that can hydrolyze proteins under the highly acidic conditions present within the human stomach.54 In this study, a globular protein (lactoferrin, LF) was used as an emulsifier to form the primary emulsions, and so each lipid droplet was initially covered by a layer of LF molecules prior to introduction into the simulated GIT model. After lipid droplets enter the stomach, pepsin may hydrolyze the adsorbed protein layer, thereby leading to changes in emulsion microstructure and stability.55,56 In the secondary emulsions the protein layer was initially covered by an additional layer of anionic polysaccharide molecules (dietary fibers), which may have altered the ability of pepsin to access the protein molecules adsorbed to the lipid droplet surfaces. We therefore examined the ability of pepsin in the simulated gastric fluid (SGF) to influence the physiochemical properties and digestibility of the emulsified lipids in the primary and secondary emulsions.
We did not find an appreciable effect of pepsin on the electrical characteristics or physicochemical stability of the lipid droplets in the simulated GIT. The physical stability and electrical characteristics of the lipid droplets was similar in the presence (Fig. 6 and 7) and absence (Fig. 1 and 2) of pepsin: emulsions were stable to aggregation in SGF (with the exception of LF-alginate); emulsions were unstable to aggregation and creaming in SIF. We also examined the influence of excluding pepsin from the SGF on the subsequent lipase digestibility of lipid droplets in the SIF (Fig. 8). One might expect pepsin to have an appreciable influence on the digestibility of emulsified lipids because it could hydrolyze and remove the protein coating from the lipid droplets, thereby improving their accessibility to lipase. However, we did not find a major impact of pepsin on the in vitro digestibility of the lipids in the simulated small intestinal conditions using the pH stat method. The rate and extent of lipid digestion was fairly similar in the presence (Fig. 8) and absence (Fig. 5) of pepsin. In both the absence and presence of pepsin in the SGF used to pretreat the emulsions, the overall extent of lipid digestion after 2 h was similar in all of the emulsions, but the initial rate of lipid digestion was somewhat slower in the alginate-coated emulsions than in the other secondary emulsions.
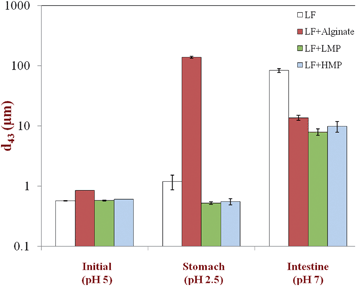 |
| Fig. 6 Effect of simulated gastrointestinal tract conditions on the mean particle diameters (d43) of primary emulsion (LF-coated) and secondary emulsions (LF-alginate, LF-LMP and LF-HMP coated). Note: The SGF contained pepsin. | |
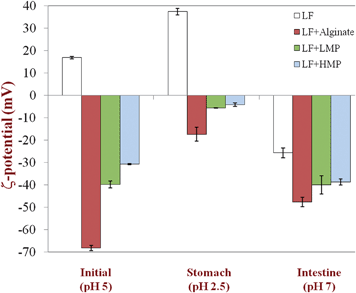 |
| Fig. 7 Effect of simulated gastrointestinal tract conditions on the particle charge (ζ-potential) of primary emulsion (LF-coated) and secondary emulsions (LF-alginate, LF-LMP and LF-HMP coated). Note: SGF contained pepsin. | |
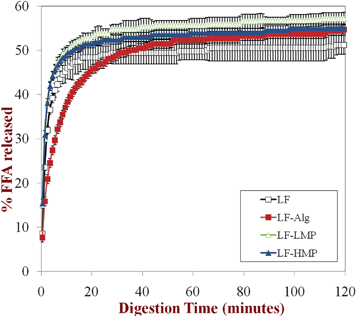 |
| Fig. 8
Lipid digestibility of the primary emulsion (LF-coated) and secondary emulsions (LF-alginate, LF-LMP and LF-HMP coated) in simulated small intestine conditions (SIF) measured using a pH-stat method (pH 7). Prior to testing, the emulsions had been incubated in SGF containing pepsin. | |
These results suggest that pepsin did not play a critical role in the behavior of emulsified lipids within the simulated GIT. The adsorption of globular proteins to lipid droplet surfaces is known to influence their protease digestibility, e.g., adsorbed β-lactoglobulin is digested more rapidly than free β-lactoglobulin.57 This phenomenon was attributed to the conformational change that globular proteins undergo when they adsorb to oil–water interfaces, which leads to alterations in the susceptibility of the peptide chain to cleavage by digestive enzymes. Previous studies with β-lactoglobulin-coated lipid droplets have shown that they are less stable to aggregation under simulated gastric conditions in the presence of pepsin than in its absence, which was attributed to an increased amount of droplet flocculation and coalescence when the protein coating was hydrolyzed to smaller peptides.42 Our results suggest that LF-coated lipid droplets were less prone to pepsin-induced aggregation in the stomach than β-lactoglobulin-coated ones. A possible explanation of this phenomenon is that either the adsorbed LF was not digested by pepsin or that the peptide fragments formed by LF hydrolysis were still surface active and remained at the oil–water interface. The lipid droplets coated by LF hydrolysis products may therefore have acted similarly to lipid droplets coated by non-digested LF.
4. Conclusions
We have studied the influence of dietary fiber coatings on the physicochemical properties and digestibility of protein-coated lipid droplets under simulated GIT conditions. The nature of the coating surrounding the emulsified lipids had an appreciable impact on their physicochemical stability within the simulated GIT. LF, LF-LMP and LF-HMP emulsions were stable to droplet aggregation in the stomach but aggregated in the small intestine, whereas LF-alginate emulsions aggregated in both the stomach and small intestine. The difference in fate of the lipid droplets was interpreted in terms of the influence of the various dietary fibers on the colloidal interactions between the droplets. The presence of the dietary fiber coatings around the lipid droplets had little influence on the rate or extent of lipid digestion, in both the absence and presence of pepsin. This knowledge is important for the design of delivery systems for encapsulation of lipophilic bioactive ingredients. For example, multilayer emulsion-based delivery systems can be designed to improve the physicochemical stability of lipids in food products, while still enabling the encapsulated components to be released in the GIT.
Acknowledgements
This material is based upon work supported by the Cooperative State Research, Extension, Education Service, United State Department of Agriculture, Massachusetts Agricultural Experiment Station and a United States Department of Agriculture, CREES, NRI and AFRI Grants.
References
- L. Chen, G. E. Remondetto and M. Subirade, Trends Food Sci. Technol., 2006, 17, 272–283 CrossRef CAS.
- F. Kesisoglou, S. Panmai and Y. H. Wu, Curr. Nanosci., 2007, 3, 183–190 CrossRef CAS.
- S. L. Kosaraju, Crit. Rev. Food Sci. Nutr., 2005, 45, 251–258 CrossRef CAS.
- L. Sagalowicz, M. Leser, H. J. Watzke and M. Michel, Trends Food Sci. Technol., 2006, 17, 204–214 CrossRef CAS.
- J. Flangan and H. Singh, Crit. Rev. Food Sci. Nutr., 2006, 46, 221–237 CrossRef.
- A. Spernath and A. Aserin, Adv. Colloid Interface Sci., 2006, 128, 47–64 CrossRef.
- T. M. Taylor, P. M. Davidson, B. D. Bruce and J. Weiss, Crit. Rev. Food Sci. Nutr., 2005, 45, 587–605 CrossRef CAS.
- D. Guzey and D. J. McClements, Adv. Colloid Interface Sci., 2006, 128, 227–248 CrossRef.
- D. Guzey and D. J. McClements, J. Agric. Food Chem., 2007, 55, 475–485 CrossRef CAS.
- F. Caruso and H. Mohwald, J. Am. Chem. Soc., 1999, 121, 6039–6046 CrossRef CAS.
- Y. H. Cho and D. J. McClements, Langmuir, 2009, 25, 6649–6657 CrossRef CAS.
- P. Thanasukarn, R. Pongsawatmanit and D. J. McClements, Food Res. Int., 2006, 39, 721–729 CrossRef CAS.
- D. J. McClements, J. Food Sci., 2010, 75, R30–R42 CrossRef CAS.
- T. Harnsilawat, R. Pongsawatmanit and D. J. McClements, Food Hydrocolloids, 2006, 20, 577–585 CrossRef CAS.
- T. Tokle, U. Lesmes and D. J. McClements, J. Agric. Food Chem., 2010, 58, 9825–9832 CrossRef CAS.
- M. V. Lomova, G. B. Sukhorukov and M. N. Antipina, ACS Appl. Mater. Interfaces, 2010, 2, 3669–3676 CAS.
- V. Gudipati, S. Sandra, D. J. McClements and E. A. Decker, J. Agric. Food Chem., 2010, 58, 8093–8099 CrossRef CAS.
- U. Lesmes, S. Sandra, E. A. Decker and D. J. McClements, Food Chem., 2010, 123, 99–106 CrossRef CAS.
- U. Klinkesorn and D. J. McClements, Food Biophys., 2010, 5, 73–81 CrossRef.
- D. J. McClements and Y. Li, Adv. Colloid Interface Sci., 2010, 159, 213–228 CrossRef CAS.
- M. Hu, Y. Li, E. A. Decker, H. Xiao and D. J. McClements, Food Biophys., 2010, 6, 37–48 CrossRef.
- L. Lundin, M. Golding and T. J. Wooster, Nutr. Diet., 2008, 65, S79–S85 CrossRef.
- H. Singh, A. Q. Ye and D. Horne, Prog. Lipid Res., 2009, 48, 92–100 CrossRef CAS.
- J. H. Brock, Biochem. Cell Biol., 2002, 80, 1–6 CrossRef CAS.
- B. Lonnerdal and S. Iyer, Annu. Rev. Nutr., 1995, 15, 93–110 CrossRef CAS.
-
S. W. Cui, Food Carbohydrates: Chemistry, Physical Properties and Applications, Taylor and Francis, Boca Raton, FL, 2005 Search PubMed.
- C. K. Siew, P. A. Williams and N. W. G. Young, Biomacromolecules, 2005, 6, 963–969 CrossRef CAS.
- M. Beysseriat, E. A. Decker and D. J. McClements, Food Hydrocolloids, 2006, 20, 800–809 CrossRef CAS.
- S. Mun, E. A. Decker, Y. Park, J. Weiss and D. J. McClements, Food Biophys., 2006, 1, 21–29 CrossRef.
- M. Hu, Y. Li, E. A. Decker, H. Xiao and D. J. McClements, Journal of Agricultural and Food Chemistry, 2010, 58, 1283–1289 CrossRef CAS.
- A. Sarkar, K. K. T. Goh and H. Singh, Food Hydrocolloids, 2010, 24, 534–541 CrossRef CAS.
- A. Sarkar, K. K. T. Goh, R. P. Singh and H. Singh, Food Hydrocolloids, 2009, 23, 1563–1569 CrossRef CAS.
- A. Sarkar, D. S. Horne and H. Singh, Food Hydrocolloids, 2010, 24, 142–151 CrossRef CAS.
- U. Lesmes, P. Baudot and D. J. McClements, J. Agric. Food Chem., 2010, 58, 7962–7969 CrossRef CAS.
- D. J. McClements and Y. Li, Food Funct., 2010, 1, 32–59 CAS.
- D. J. McClements, E. A. Decker and Y. Park, Crit. Rev. Food Sci. Nutr., 2009, 49, 48–67 CrossRef.
- D. J. McClements and Y. Li, Adv. Colloid Interface Sci., 2010, 159, 213–228 CrossRef CAS.
-
D. J. McClements, Food Emulsions: Principles, Practice, and Techniques, CRC Press, Boca Raton, 2005 Search PubMed.
- M. Golding and T. J. Wooster, Curr. Opin. Colloid Interface Sci., 2010, 15, 90–101 CrossRef CAS.
- L. Marciani, R. Faulks, M. S. J. Wickham, D. Bush, B. Pick, J. Wright, E. F. Cox, A. Fillery-Travis, P. A. Gowland and R. C. Spiller, Br. J. Nutr., 2009, 101, 919–928 CrossRef CAS.
- L. Marciani, M. Wickham, G. Singh, D. Bush, B. Pick, E. Cox, A. Fillery-Travis, R. Faulks, C. Marsden, P. A. Gowland and R. C. Spiller, Gastroenterology, 2006, 130, A227–A227 Search PubMed.
- A. Sarkar, K. K. T. Goh and H. Singh, Food Hydrocolloids, 2010, 24, 534–541 CrossRef CAS.
- N. Thirawong, J. Nunthanid, S. Puttipipatkhachorn and P. Sriamornsak, Eur. J. Pharm. Biopharm., 2007, 67, 132–140 CrossRef CAS.
- E. N. Baker and H. M. Baker, Cell. Mol. Life Sci., 2005, 62, 2531–2539 CrossRef CAS.
- T. Tokle and D. J. McClements, Food Hydrocolloids, 2011, 25, 976–982 CrossRef CAS.
- D. Guzey and D. J. McClements, J. Agric. Food Chem., 2007, 55, 475–485 CrossRef CAS.
-
Food Carbohydrates: Chemistry, Physical Properties and Applications, ed. S. W. Cui, Taylor & Francis, Boca Raton, FL, 2005 Search PubMed.
- T. Tokle and D. J. McClements, Food Hydrocolloids, 2010, 25, 976–982 CrossRef.
- W. G. T. Willats, P. Knox and J. D. Mikkelsen, Trends Food Sci. Technol., 2006, 17, 97–104 CrossRef CAS.
- T. Harnsilawat, R. Pongsawatmanit and D. McClements, J. Agric. Food Chem., 2006, 54, 5540–5547 CrossRef CAS.
- J. Maldonado-Valderrama, A. P. Gunning, M. J. Ridout, P. J. Wilde and V. J. Morris, Eur. Phys. J. E, 2009, 30, 165–174 CrossRef CAS.
- M. Hu, Y. Li, E. A. Decker and D. J. McClements, Food Hydrocolloids, 2010, 24, 719–725 CrossRef CAS.
- Y. Li, M. Hu, Y. M. Du and D. J. McClements, J. Microencapsulation, 2011, 28, 166–175 CrossRef CAS.
-
L. R. Johnson, in Gastrointestinal Physiology, 6th Edition, ed. L. R. Johnson, Mosby, St. Louis, MI, 2001, pp. 75–94 Search PubMed.
- A. Sarkar, K. K. T. Goh and H. Singh, Food Hydrocolloids, 2009, 23, 1270–1278 CrossRef CAS.
- A. Sarkar, D. S. Horne and H. Singh, Int. Dairy J., 2010, 20, 589–597 CrossRef CAS.
- A. Mackie and A. Macierzanka, Curr. Opin. Colloid Interface Sci., 2010, 15, 102–108 CrossRef CAS.
|
This journal is © The Royal Society of Chemistry 2012 |
Click here to see how this site uses Cookies. View our privacy policy here.