Oxidation of xylitol by a silver(III) periodate complex in the presence of osmium(VIII) as a homogeneous catalyst
Received
21st May 2012
, Accepted 11th August 2012
First published on 14th August 2012
1. Introduction
Carbohydrates are a major source of energy for living organisms and understanding the mechanism of oxidation of carbohydrates is therefore of immense importance. Xylitol (XYL) is an organic compound with the formula (CHOH)3(CH2OH)2. Xylitol is produced via the hydrogenation of xylose, which converts the sugar (an aldehyde) into a primary alcohol. Xylitol has virtually no aftertaste and is advertised as “safe for diabetics and individuals with hyperglycemia”. This tolerance is attributed to the lower impact of xylitol on a person's blood sugar, compared to that of regular sugars, and also has a very low glycemic index of 13 (glucose has a GI of 100). Xylitol is a “tooth-friendly”, nonfermentable sugar alcohol. It is specific in its inhibition of the mutans streptococci group, bacteria that are significant contributors to tooth decay. It does not contribute to high blood sugar levels or the resulting hyperglycemia caused by an insufficient insulin response. This characteristic has also proven beneficial for people suffering from metabolic syndrome. Xylitol also has potential as a treatment for osteoporosis. A recent report suggests that the consumption of xylitol may help to control oral infections of Candida yeast.1 Since xylitol contains two –CH2OH and three –OH groups, it necessitates the need to know at which position of the groups the oxidation takes place.
The silver(III) periodate complex (DPA) is a powerful oxidizing agent in an alkaline medium with a reduction potential2 of 1.74 V. It is widely used as a volumetric reagent for the determination of various organic and inorganic species.3,4 Jayaprakash Rao et al.5,6 have used DPA as an oxidizing agent to study the kinetics of oxidation of various organic substrates. They generally found that order with respect to both the oxidant and substrate was unity and [OH−] was found to enhance the rate of reaction. It was also observed that they did not derive the possible active species of DPA in alkali and therefore, they proposed mechanisms by generalizing the DPA as [Ag(HL)L](X+1)−. However, Kumar et al.7–9 made an effort to give evidence for the reactive form of DPA at an alkaline pH, on a large scale. In the present investigation, we have obtained evidence for the reactive species for DPA in an alkaline medium.
In recent years, the use of transition metal ions, such as osmium, ruthenium and iridium, either alone or as binary mixtures, as a catalysts in various redox processes has attracted considerable interest.10 The role of osmium(VIII) as a catalyst in some redox reactions has been reviewed.11 The mechanism of catalysis depends on the nature of the substrate, the oxidant and the experimental conditions. It has been shown12 that metal ions act as a catalyst by one of several different paths, such as the formation of complexes with the reactants, the oxidation of the substrate itself or other formation of free radicals. Osmium(VIII) catalysis in redox reactions involves several complexities, different oxidation states of osmium, etc. The uncatalyzed oxidation reaction of XYL by DPA has been studied.13 We have observed that osmium(VIII) catalyzes the oxidation of XYL by DPA in an alkaline medium in micro amounts.
A literature survey reveals that there are no reports on the mechanism of the osmium catalyzed oxidation of xylitol by DPA in an alkaline medium. Hence, in view of the pharmaceutical importance of xylitol and in order to understand the active species of the oxidant and catalyst and to identify a suitable mechanism, the title reaction was investigated. An understanding of the mechanism allows the chemistry to be interpreted, understood and predicted.
2. Experimental and methods
2.1. Materials and reagents
All the chemicals used were of reagent grade and double distilled water was used throughout the work. A solution of xylitol (HIMEDIA) was prepared by dissolving an appropriate amount of the recrystallized sample in double distilled water. The purity of the xylitol sample was checked by comparing its IR spectrum with the literature data and with its m.p. 93 °C (literature value is 94 °C). The osmium(VIII) solution was prepared by dissolving OsO4(VIII) oxide (Johnson Matthey) in 0.50 mol dm−3 NaOH. The concentration was ascertained14 by determining the unreacted [Fe(CN)6]4− with a standard Ce(IV) solution in an acidic medium. The silver(III) periodate complex was prepared15,16 and standardized by a standard procedure.17 The UV-vis spectrum, with a maximum absorption at 362 nm, verified the existence of the silver(III) complex. A periodate solution was prepared by weighing the required amount of the sample in hot water and was used after 24 hours to complete the equilibrium. Its concentration was ascertained iodometrically18 at neutral pH and maintained using a phosphate buffer. KOH (BDH) and KNO3 (BDH) were employed to maintain the required alkalinity and ionic strength, respectively. The temperature was maintained to within ±0.1 °C.
2.2. Instrumentation and kinetic measurements
All kinetic measurements were performed on a Varian CARY 50 Bio UV-vis Spectrophotometer. The kinetics were followed under pseudo first-order conditions where [XYL] > [DPA] at 25.0 ± 0.1 °C, unless specified. The reaction was initiated by mixing DPA with the xylitol solution, which also contained the required concentrations of KNO3, KOH, KIO4 and the Os(VIII) catalyst. The progress of the reaction was monitored spectrophotometrically at 360 nm (i.e. a decrease in the absorbance due to DPA with the molar absorbancy index, ‘ε’ to be 13
900 ± 100 dm3 mol−1 cm−1), which is the maximum absorption wavelength of DPA. The spectral changes during the chemical reaction for the standard conditions at 298 K are given in Fig. 1. It was verified that there was almost no interference from other species in the reaction mixture at this wavelength.
![UV-vis spectroscopic changes occurring in the Os(viii) catalysed oxidation of xylitol by alkaline diperiodatoargentate(iii) at 298 K, [DPA] = 5.0 × 10−5, [XYL] = 5.0 × 10−4, [Os(viii)] = 8.0 × 10−6, [OH−] = 0.04 and I = 0.06 mol dm−3, with a scanning time of (1) 0.2 min, (2) 0.4 min, (3) 0.6 min, (4) 0.8 min and (5) 1.0 min.](/image/article/2012/CY/c2cy20336g/c2cy20336g-f1.gif) |
| Fig. 1 UV-vis spectroscopic changes occurring in the Os(VIII) catalysed oxidation of xylitol by alkaline diperiodatoargentate(III) at 298 K, [DPA] = 5.0 × 10−5, [XYL] = 5.0 × 10−4, [Os(VIII)] = 8.0 × 10−6, [OH−] = 0.04 and I = 0.06 mol dm−3, with a scanning time of (1) 0.2 min, (2) 0.4 min, (3) 0.6 min, (4) 0.8 min and (5) 1.0 min. | |
The pseudo-first order rate constants, (kC), were determined from the log (absorbance) vs. time plots and were the average of two independent values. The plots were linear up to 85% completion of the reaction under the range of [OH−] used. The orders for the various species were determined from the slopes of plots of log (kC) versus the respective concentration of the species except for [DPA], in which a non-variation of ‘kC’ was observed, as expected for the reaction condition. The rate constants were reproducible to within ±5%. A regression analysis of the experimental data to obtain the regression coefficient ‘r’ and the standard deviation, S, of the points from the regression line was performed with Microsoft office Excel 2003.
3. Results
3.1. Stoichiometry and product analysis
Different sets of reaction mixtures containing varying ratios of DPA to XYL, in the presence of a constant amount of OH−, KIO4, KNO3 and Os(VIII), were kept for 3 hours in a closed vessel under a nitrogen atmosphere. The remaining concentration of DPA was assayed by measuring the absorbance at 360 nm. The results indicated a 1
:
2 stoichiometry, as given in eqn (1). |  | (1) |
The main oxidation product was identified as 2,3,4,5-tetrahydroxypentanoic acid, which was confirmed by IR and LC-MS spectra. The nature of the carboxylic acid was confirmed by the IR spectrum, which showed a carbonyl (>C
O) stretch at 1722 cm−1 and O–H stretching of the acid at 3362 cm−1. The product was also confirmed by the LC-MS analysis data. The mass spectrum showed a molecular ion peak at 166 amu, confirming the presence of the product, 2,3,4,5-tetrahydroxypentanoic acid (Fig. 2). The formation of free Ag(I) in solution was detected by adding a KCl solution to the reaction mixture, which produced white turbidity due to the formation of AgCl. It was observed that 2,3,4,5-tetrahydroxypentanoic acid did not undergo further oxidation under the present kinetic conditions.
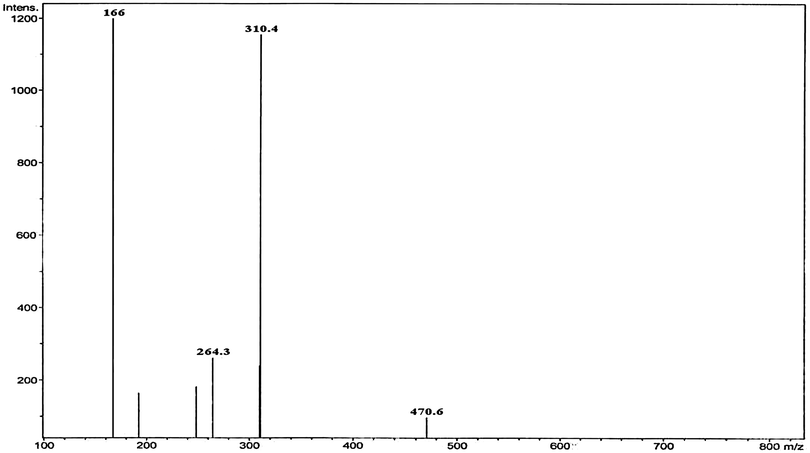 |
| Fig. 2 LC-mass spectrum of 2,3,4,5-tetrahydoxypentanoic acid with its molecular ion peak at 166 m/z. | |
3.2. Reaction order
As the diperiodatoargentate(III) oxidation of XYL in an alkaline medium proceeds with a measurable rate in the absence of Os(VIII), the catalyzed reaction is understood to occur via parallel paths with contributions from both the catalyzed and unanalyzed paths. Thus, the total rate constant (kT) is equal to the sum of the rate constants of the catalyzed (kC) and unanalyzed (kU) reactions, so kC = kT − kU. Hence, the reaction orders have been determined from the slopes of log kCvs. log (concentration) plots by varying the concentrations of XYL, IO4−, OH− and the Os(VIII) catalyst in turn, while keeping the other concentrations and conditions constant.
3.3. Effect of [diperiodatoargentate(III)]
In the presence of the Os(VIII) catalyst, the DPA concentration was varied in the range of 1.0 × 10−5 to 1.0 × 10−4 mol dm−3 and the linearity of the plots of log (absorbance) versus time up to 85% completion of the reaction indicates a reaction order of unity in [DPA] (r ≥ 0.9986, S ≤ 0.013). This is also confirmed by varying the [DPA], which did not result in any change in the pseudo-first order rate constant, kC (Table 1).
Table 1 Effect of varying the [DPA], [XYL], [OH−], [IO4−] and [Os(VIII)] on the oxidation of XYL by diperiodatoargentate(III) in an aqueous alkaline medium at 25 °C and I = 0.06 mol dm−3
[DPA] × 105 (mol dm−3) |
[XYL] × 104 (mol dm−3) |
[OH−] (mol dm−3) |
[IO4−] × 104 (mol dm−3) |
[Os(VIII)] × 106 (mol dm−3) |
k
T (× 102 s−1) |
k
U (× 103 s−1) |
k
C × 102 (s−1) |
Found |
Calculated |
1.0 |
5.0 |
0.04 |
1.0 |
8.0 |
2.22 |
5.90 |
1.62 |
1.99 |
3.0 |
5.0 |
0.04 |
1.0 |
8.0 |
2.38 |
5.96 |
1.78 |
1.99 |
5.0 |
5.0 |
0.04 |
1.0 |
8.0 |
2.46 |
5.30 |
1.93 |
1.99 |
8.0 |
5.0 |
0.04 |
1.0 |
8.0 |
2.56 |
5.55 |
2.01 |
1.99 |
10 |
5.0 |
0.04 |
1.0 |
8.0 |
2.62 |
5.94 |
2.02 |
1.99 |
5.0 |
1.0 |
0.04 |
1.0 |
8.0 |
0.55 |
1.16 |
0.44 |
0.45 |
5.0 |
3.0 |
0.04 |
1.0 |
8.0 |
1.52 |
2.89 |
1.23 |
1.28 |
5.0 |
5.0 |
0.04 |
1.0 |
8.0 |
2.46 |
5.30 |
1.93 |
1.99 |
5.0 |
10.0 |
0.04 |
1.0 |
8.0 |
4.32 |
8.55 |
3.46 |
3.43 |
5.0 |
20.0 |
0.04 |
1.0 |
8.0 |
6.18 |
10.9 |
5.09 |
5.50 |
5.0 |
5.0 |
0.005 |
1.0 |
8.0 |
1.03 |
0.90 |
0.94 |
0.96 |
5.0 |
5.0 |
0.01 |
1.0 |
8.0 |
1.39 |
1.40 |
1.25 |
1.36 |
5.0 |
5.0 |
0.02 |
1.0 |
8.0 |
1.82 |
2.60 |
1.56 |
1.73 |
5.0 |
5.0 |
0.04 |
1.0 |
8.0 |
2.46 |
5.30 |
1.93 |
1.99 |
5.0 |
5.0 |
0.06 |
1.0 |
8.0 |
2.90 |
7.79 |
2.12 |
2.10 |
5.0 |
5.0 |
0.04 |
0.5 |
8.0 |
3.11 |
5.70 |
2.54 |
2.75 |
5.0 |
5.0 |
0.04 |
1.0 |
8.0 |
2.46 |
5.30 |
1.93 |
1.99 |
5.0 |
5.0 |
0.04 |
2.0 |
8.0 |
1.99 |
5.21 |
1.46 |
1.28 |
5.0 |
5.0 |
0.04 |
3.0 |
8.0 |
1.41 |
4.80 |
0.93 |
0.94 |
5.0 |
5.0 |
0.04 |
5.0 |
8.0 |
1.02 |
3.99 |
0.62 |
0.62 |
5.0 |
5.0 |
0.04 |
1.0 |
2.0 |
1.08 |
5.30 |
0.55 |
0.50 |
5.0 |
5.0 |
0.04 |
1.0 |
3.0 |
1.28 |
5.30 |
0.75 |
0.74 |
5.0 |
5.0 |
0.04 |
1.0 |
5.0 |
2.21 |
5.30 |
1.68 |
1.24 |
5.0 |
5.0 |
0.04 |
1.0 |
8.0 |
2.46 |
5.30 |
1.93 |
1.99 |
5.0 |
5.0 |
0.04 |
1.0 |
20.0 |
5.74 |
5.30 |
5.21 |
4.98 |
3.4. Effect of [XYL]
The effect of XYL was studied in the range of 1.0 × 10−4 to 2.0 × 10−3 mol dm−3 at 25 °C while keeping the other reactant concentrations and conditions constant. The kC values increased with the increase in concentration of xylitol. The order with respect to [XYL] was found to be less than unity, 0.82, (Table 1) (r ≥ 0.9995, S ≤ 0.007) under the experimental conditions. This less than unit order in the [XYL] was also confirmed by the linear plot of kCversus [XYL]0.82 and the nonlinearity of the plot of kCversus [XYL] (Fig. 3).
![Plot of kCvs. [XYL]0.82 and kCvs. [XYL].](/image/article/2012/CY/c2cy20336g/c2cy20336g-f3.gif) |
| Fig. 3 Plot of kCvs. [XYL]0.82 and kCvs. [XYL]. | |
3.5. Effect of [Os(VIII)]
The osmium(VIII) concentration was varied from 1.0 × 10−6 to 1.0 × 10−5 mol dm−3 range, at constant concentrations of DPA, XYL, alkali and at a constant ionic strength. The order in [Os(VIII)] was found to be unity (Table 1). (kCvs. [Os(VIII)] was linear).
3.6. Effect of [alkali] and [periodate]
The effect of the alkali on the reaction was studied in the range of 0.005 to 0.06 mol dm−3 at constant concentrations of XYL, DPA, IO4−, Os(VIII) and at a constant ionic strength of 0.06 mol dm−3. The rate constant increased with an increase in [alkali] and the order was found to be a positive fraction i.e. 0.32.
The effect of periodate was observed by varying the concentration from 5.0 × 10−5 to 5.0 × 10−4 mol dm−3 while keeping all other reactants concentrations constant. It was observed that the rate constant decreased with an increase in [periodate] and the order was found to be a negative fraction i.e. −0.60.
3.7. Effect of the initially added products
The initially added products, Ag(I) and 2,3,4,5-tetrahydroxypentanoic acid, did not have any significant effect on the rate of the reaction.
3.8. Effect of the ionic strength and dielectric constant of the medium (D)
The effect of varying [KNO3] at constant [DPA], [XYL], [OH−], [Os(VIII)] and [IO4−] was found that an increase in the ionic strength had no significant effect on the rate of the reaction.
The dielectric constant of the medium, ‘D’ was varied by varying the t-butyl alcohol and water percentage. The D values were calculated from the equation D = DwVw + DBVB, where Dw and DB are the dielectric constants of pure water and t-butyl alcohol, respectively. Vw and VB are the volume fractions of water and t-butyl alcohol components respectively, in the total mixture. It was observed that the rate constant increased with an decrease in the dielectric constant of the reaction medium and the plot of log kCvs. 1/D was linear with a positive slope. It was also tested for any possible oxidation by DPA of the solvent used under the experimental conditions and it was found that no oxidation of the solvent by DPA occured.
Thus, from the observed experimental results, the rate law for the Os(VIII) catalyzed reaction is given as:
Rate = kC [DPA]1.0 [XYL]0.82 [OH−]0.32 [IO4−]−0.60 [Os(VIII)]1.0 |
3.9. Effect of temperature (T)
The influence of temperature on the kC values was studied at 15, 20, 25, 30 and 35 °C under varying concentrations of XYL, alkali, osmium(VIII) and periodate, while keeping the other conditions constant. The rate constant was found to increase with an increase in temperature. The rate constants, k, of the slow step of Scheme 1 were obtained from the intercepts of the Os(VIII)/kCvs. 1/[XYL] plots at five different temperatures and used to calculate the activation parameters. The energy of activation corresponding to these constants was evaluated from the Arrhenius plot of log kCvs. 1/T (r ≥ 0.9955, S ≤ 0.005) and the other activation parameters which were obtained are tabulated in (Table 2).
 |
| Scheme 1 Detailed mechanism of the osmium(VIII) catalyzed oxidation of xylitol by alkaline diperiodatoargentate(III). | |
Table 2 Activation parameters and thermodynamic quantities for the osmium(VIII) catalyzed oxidation of XYL by diperiodatoargentate(III) in an aqueous alkaline medium with respect to the slow step of Scheme 1
(A) Effect of temperature |
Temperature (K) |
k × 10−4 dm3 mol−1 s−1 |
288 |
0.77 |
293 |
1.15 |
298 |
1.52 |
303 |
2.35 |
308 |
3.18 |
(B) Activation parameters |
Parameters |
Values |
E
a (kJ mol−1) |
52.2 ± 2.6 |
ΔH# (kJ mol−1) |
49.8 ± 2.4 |
ΔS# (JK−1 mol−1) |
2.6 ± 0.1 |
ΔG#298 (kJ mol−1) |
49.0 ± 2.5 |
log A |
13.3 ± 0.7 |
(C) Effect of temperature to calculate K1, K2 and K3 |
Temperature (K) |
K
1 × 10−1 (dm3 mol−1) |
K
2 × 105 (mol dm−3) |
K
3 × 10−3 (dm3 mol−1) |
288 |
8.49 |
4.18 |
1.50 |
293 |
7.47 |
5.67 |
1.32 |
298 |
6.55 |
7.15 |
1.14 |
303 |
4.58 |
9.18 |
1.03 |
308 |
2.60 |
11.2 |
0.92 |
(D) Thermodynamic quantities using K1, K2 and K3 |
Thermodynamic quantities |
Values from K1 |
Values from K2 |
Values from K3 |
ΔH (kJ mol−1) |
−41.4 ± 2.0 |
−109.0 ± 5.4 |
−18.1 ± 0.9 |
ΔS (JK−1 mol−1) |
−105.7 ± 5.2 |
42.2 ± 2.1 |
−2.0 ± 0.1 |
ΔG298 (kJ mol−1) |
−9.9 ± 0.5 |
23.7 ± 1.2 |
−17.5 ± 0.9 |
3.10. Test for free radicals
The intervention of free radicals was examined as follows. The reaction mixture, to which a known quantity of acrylonitrile monomer had been added initially, was kept in an inert atmosphere for 2 hours. On diluting the reaction mixture with methanol, a white precipitate was formed, indicating the intervention of free radicals in the reaction. The blank experiments of either DPA or XYL alone with acrylonitrile did not induce any polymerization under the same conditions as those induced for the reaction mixture. Initially, the addition of acrylonitrile decreases the rate of reaction, indicating free radical intervention, which is the case in earlier work.19
3.11. Catalytic activity
It has been pointed out by Moelwyn-Hughes20 that, in the presence of the catalyst, the uncatalyzed and catalyzed reactions proceed simultaneously, so that | kT = kU + KC [catalyst]x | (2) |
Here, kT is the observed pseudo first-order rate constant in the presence of the Os(VIII) catalyst, kU is the pseudo first-order rate constant for the uncatalyzed reaction, KC is the catalytic constant and ‘x’ is the order of the reaction with respect to [Os(VIII)]. In the present investigations, the x values for the standard run were found to be unity for Os(VIII). Consequently, the value of KC can be calculated using the equation:
|  | (3) |
The values of KC were evaluated at different temperatures and found to vary. Furthermore, the plot of log KCversus 1/T was linear and the values of the energy of activation and other activation parameters with reference to the catalyst were computed. These results are summarized in (Table 3).
Table 3 Values of the catalytic constant (KC) at different temperatures and the activation parameters calculated using the KC values
Temperature (K) |
K
c × 10−3 |
288 |
1.2 |
293 |
1.8 |
298 |
2.4 |
303 |
3.6 |
308 |
4.8 |
E
a (kJ mol−1) |
51.2 ± 2.5 |
ΔH# (kJ mol−1) |
48.7 ± 2.4 |
ΔS# (JK−1 mol−1) |
−15.9 ± 0.8 |
ΔG# (kJ mol−1) |
53.4 ± 2.7 |
log A |
12.4 ± 0.6 |
4. Discussion
In the later period of the 20th century, the kinetics of the oxidation of some organic and inorganic substrates have been studied by Ag(III) species, which may be due to its strong versatile nature as a two electron oxidant. Among the various species of Ag(III), Ag(OH)4−, diperiodatoargentate(III) (DPA) and ethylenebis (biguanide) silver(III) (EBS) are of maximum interest to researchers due to their relative stability.21 The stability of Ag(OH)4− is sensitive towards traces of dissolved oxygen and other impurities in the reaction medium and therefore, it has not received much attention. However, the other two forms of Ag(III)21 are considerably more stable and the DPA is used in highly alkaline media and EBS is used in highly acidic media.
According to the literature,15 water soluble DPA has the formula [Ag(IO6)2]7− with a dsp2 configuration and a square planar structure, similar to diperiodatocopper(III), with two bidentate periodate ligands forming a planar molecule. In alkaline media, it is unlikely to exist as [Ag(IO6)2]7− since periodate is known to exist in various protonated forms22 depending on the pH of the solution, as given in the following multiple equilibria in eqn (4)–(6).
| H3IO62− ⇌ H4IO63− + H+ | (6) |
Periodic acid (H5IO6) exists in acid media and also as H4IO6− at pH 7. Thus, under alkaline conditions, the main species is expected to be H3IO62−. At higher concentrations, periodate also tends to dimerise. However, the formation of this species is negligible under the conditions used in these kinetic studies. On the contrary, authors5,6 in recent studies have proposed the DPA as [Ag(HL)2]x−, in which L is a periodate with an uncertain number of protons and HL is a protonated periodate of an uncertain number of protons. This can be ruled out by considering the alternative forms22 of IO4− at pH > 7, which is in the form of H3IO62− or H2IO63−. Hence, DPA could be as [Ag(H3IO6)2]− or [Ag(H2IO6)2]3− in alkaline media. Therefore, under the present conditions, diperiodatoargentate(III), may be depicted as [Ag(H3IO6)2]−. A similar speciation of periodate in alkali was proposed23 for diperiodatonickelate(IV).
Osmium(VIII) is known to form different complexes as [OsO4(OH)2]2− and [OsO5(OH)]3− at different OH− concentrations24. At higher concentrations of OH−, [OsO5(OH)]3− is significant. At lower concentrations of OH−, as employed in the present study and since the rate of oxidation increased with an increase in [OH−], it is reasonable that [OsO4(OH)2]2− was operative and its formation is important in the reaction.
4.1. Mechanism for the reaction
The reaction between DPA and XYL in the presence of the Os(VIII) catalyst in an alkaline medium has a 1
:
2 stoichiometry (xylitol
:
DPA) with a first order dependence on [DPA] and Os(VIII) and an apparent order of less than unit order in [substrate], a positive fractional order dependence on [alkali] and a negative fractional order dependence on [periodate]. No effect from the added products was observed. Based on the order with respect to the reactants, such as [DPA], [OH−], [periodate], [Os(VIII)] and [XYL], the following mechanism has been proposed by considering XYL in its neutral form, which explains all the other experimental observations in Scheme 1.
In the prior equilibrium, during step 1 the [OH−] deprotonates the DPA to give a deprotonated diperiodatoargentate(III) and in the second step, the displacement of a ligand on periodate takes place to give a free periodate, which is evidenced by a decrease in the rate with an increase in the [periodate] (Table 1). It may be expected that a lower Ag(III) periodate species, such as MPA, is more important in the reaction than DPA. The inverse fractional order in [H3IO62−] might also be due to this reason. In the pre-rate determining stage, the Os(VIII) species combines with a neutral molecule of XYL to give an intermediate complex (C), which further reacts with one mole of monoperiodatoargentate(III) (MPA) in a rate-determining step to give a free radical derived from XYL with the regeneration of the catalyst, osmium(VIII). This free radical of XYL combines with the Ag(II) species in a fast step to give an aldehyde, which reacts with another molecule of MPA in a fast step to give the products. Thus, all these results indicate a mechanism of the type shown in Scheme 1.
Spectroscopic evidence for the complex formation between Os(VIII) and XYL was obtained from the UV-vis spectra of XYL (5.0 × 10−4 mol dm−3), Os(VIII) (8 × 10−6 mol dm−3), ([OH−] = 0.04 mol dm−3) and a mixture of both. A hypsochromic shift of 6 nm from 317 nm to 311 nm in the spectra of Os(VIII) to the mixture of Os(VIII) and XYL was observed (Fig. 4). Attempts to separate and isolate the complex were not successful. The Michaelis–Menten plot proved the complex formation between the catalyst and substrate, which explains the less than unit order in [XYL]. Such a complex between a catalyst and substrate has also been observed in other studies.15 The rate law for Scheme 1 could be derived as:
|  | (7) |
In view of the low concentrations of [H3IO62−] and [XYL] used, the last two terms of the denominator may be neglected when compared to the concentration of H3IO62−
| 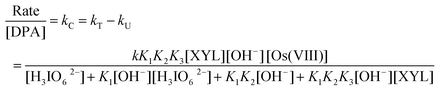 | (8) |
The rate law in eqn (8) can be rearranged into the following form, which is suitable for verification.
| 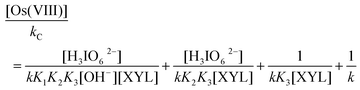 | (9) |
According to eqn (9), with the other conditions remaining constant, the plots of [Os(VIII)]/kCvs. [H3IO62−] (r ≥ 0.9798, S ≤ 0.011), [Os(VIII)]/kCvs. 1/[OH−] (r ≥ 0.9904, S ≤ 0.016) and [Os(VIII)]/kCvs. 1/[XYL] (r ≥ 0.9964, S ≤ 0.023) should be linear, as verified in Fig. 5. From the intercepts and slopes of such plots, the reaction constants K1, K2, K3 and k were calculated as 6.5 × 101 dm3 mol−1, 7.1 × 10−5 mol dm−3, 1.14 × 103 dm3 mol−1, 1.52 × 104 dm3 mol−1 s−1 respectively. The equilibrium constant K1 is far greater than K2. This may be attributed to the greater tendency of DPA to undergo de-protonation compared to the formation of the hydrolyzed species in alkaline media. The negligible effect of the ionic strength on the rate of reaction qualitatively explains the involvement of the neutral molecule, as seen in Scheme 1. The effect of the solvent on the reaction rate has been described in detail in the literature.20 In the present study, the plot of log kCvs. 1/D is linear with a positive slope, which seems to be contrary to the expected reaction between the neutral and cationic species in media with a lower dielectric constant. However, an increase in the rate of the reaction with a decreasing dielectric constant may be due to the stabilization of the complex at low dielectric constants.
![Verification of the rate law in (9) of the Os(viii) catalyzed oxidation of XYL by DPA at five different temperatures. Plots of (A) [Os(viii)]/kCvs. 1/[XYL], (B) [Os(viii)]/kCvs. 1/[OH−], (C) [Os(viii)]/kCvs. [H3IO62−].](/image/article/2012/CY/c2cy20336g/c2cy20336g-f5.gif) |
| Fig. 5 Verification of the rate law in (9) of the Os(VIII) catalyzed oxidation of XYL by DPA at five different temperatures. Plots of (A) [Os(VIII)]/kCvs. 1/[XYL], (B) [Os(VIII)]/kCvs. 1/[OH−], (C) [Os(VIII)]/kCvs. [H3IO62−]. | |
The thermodynamic quantities for the different equilibrium steps in Scheme 1 can be evaluated as follows. The [XYL], [OH−] and [H3IO62−] (Table 1) were varied at five different temperatures. The plots of [Os(VIII)]/kCvs. 1/[XYL], [Os(VIII)]/kCvs. 1/[OH−] and [Os(VIII)]/kCvs. [H3IO62−] should be linear (Fig. 5). From the slopes and intercepts, the values of K1 were calculated at different temperatures. A vant Hoff's plot was made for the variation of K1 with temperature [i.e. log K1vs. 1/T (r ≥ 0.9851, S ≤ 0.1121)] and the thermodynamic parameters were calculated. These values are given in Table 2. A comparison of the latter values with those obtained for the slow step of the reaction shows that these values mainly refer to the rate-limiting step, supporting the fact that the reaction before the rate-determining step is fairly fast and involves a high activation energy.25 In the same manner, K2 and K3 were calculated at different temperatures and the corresponding thermodynamic quantities are given in Table 2.
The values of ΔS# and ΔH# were both favorable for the electron transfer processes. The favorable enthalpy was due to the release of energy on the solution changes in the transition state. The low value of the enthalpy of activation obtained might be due to the involvement of prior equilibrium steps, as given in Scheme 1.26 The positive value of ΔS# is normal in the case of fast reactions27 involving a distribution of charges, as given in Scheme 1. The observed modest enthalpy of activation and higher rate constant for the slow step suggest that the oxidation presumably occurs via an inner-sphere mechanism. This conclusion is supported by earlier observations.28,29
5. Conclusion
The Os(VIII) catalyzed oxidation of xylitol by diperiodatoargentate was studied. The oxidation products were identified. Among the various species of Ag(III) in the alkaline medium, MPA, i.e., [Ag(H2IO6)(H2O)2] is consideredto be the active species for the title reaction. The active species of Os(VIII) is [OsO4(OH)2]2−. The thermodynamic activation parameters of individual steps in the mechanism were evaluated for the Os(VIII) catalyzed reaction at different temperatures. The catalytic constant and the activation parameters with reference to the catalyst were also computed.
Appendix
According to Scheme 1. | 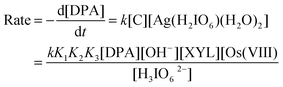 | (A.1) |
The total concentration of DPA is given by (where T and f stands for total and free)
Therefore
|  | (A.2) |
Similarly,
In view of the low concentration of Os(
VIII) used
Similarly,
| 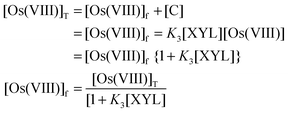 | (A.4) |
Similarly,
In view of low concentration of [DPA] and [H3IO62−]
Substituting the
eqn (A.2)–(A.5) in
eqn (A.1) and omitting the T and f subscripts, we get
| 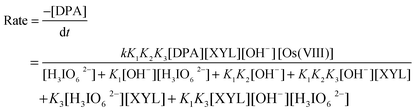 | (A.6) |
| 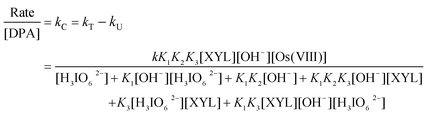 | (A.7) |
Acknowledgements
The authors thank the UGC, New Delhi for the sanction of a major research project, F No. 36-136/2008 (SR), dated 27/03/2009. J. I. Gowda thanks UGC, New Delhi, for the award of a Research Fellowship in Science for Meritorious Students (RFSMS).
References
- K. H. Abu-Elteen, Microb. Ecol. Health Dis., 2005, 17, 156–162 CrossRef CAS.
-
B. Sethuram, Some aspects of electron transfer reactions involving organic molecules,Allied Publishers (P) Ltd., New Delhi, 2003, p. 151 Search PubMed.
- P. K. Jaiswal and K. L Yadava, Talanta, 1970, 17, 236–238 CrossRef CAS.
- P. K. Jaiswal, Analyst, 1972, 1, 503–506 CAS.
- P. Jaya Prakash Rao, B. Sethuram and T. Navaneeth Rao, React. Kinet. Catal. Lett., 1985, 29, 289–296 CrossRef.
- K. Venkata Krishna and P. Jaya Prakash Rao, Indian J. Chem., 1998, 37A, 1106–1110 Search PubMed.
- A. Kumar, P. Kumar and P. Ramamurthy, Polyhedron, 1999, 18, 773–780 CrossRef CAS.
- A. Kumar and P. Kumar, J. Phys. Org. Chem., 1999, 12, 79–85 CrossRef CAS.
- A. Kumar, P. Vaishali and P. Ramamurthy, Int. J. Chem. Kinet., 2000, 32, 286–293 CrossRef CAS.
- A. K. Das, Coord. Chem. Rev., 2001, 213, 307–325 CrossRef CAS.
- M. C. Agrawal and S. K. Upadhyay, J. Sci. Ind. Res., 1983, 42, 508–517 CAS.
- P. Veerasomaiah, K. B. Reddy, B. Sethuram and T. Navaneeth Rao, Indian J. Chem., 1987, 26A, 402–406 Search PubMed.
- J. I. Gowda and S. T. Nandibewoor, Synth. React. Inorg. Met.–Org. Chem. DOI:10.1080/1553-3174.2012.684261.
- O. C. Saxena, Microchem. J., 1967, 12, 609–611 CrossRef CAS.
- G. L. Cohen and G. Atkinson, Inorg. Chem., 1964, 3, 1741–1743 CrossRef CAS.
- R. R. Hosamani, N. P. Shetti and S. T. Nandibewoor, J. Phys. Org. Chem., 2009, 22, 234–240 CrossRef CAS.
-
G. H. Jeffery, J. Bassett, J. Mendham and R. C. Denney, Vogel's textbook of quantitative chemical analysis, ELBS, New York, 6th edn, 1996, p. 679 Search PubMed.
- G. P. Panigrahi and P. K. Misro, Indian J. Chem., 1977, 15A, 1066–1069 CAS.
- R. V. Jagadeesh and Puttaswamy, J. Phys. Org. Chem., 2008, 21, 844–858 CrossRef CAS.
-
E. A. Moelwyn-Hughes, Kinetics of Reactions in Solutions, Physical Chemistry, Pergamon Press, New York, 2nd edn, 1961, pp. 297–299 Search PubMed.
- L. J. Krishenbaum and L. Mrozowski, Inorg. Chem., 1978, 17, 3718–3719 CrossRef.
- C. E. Crouthamel, A. M. Hayes and D. S. Martin, J. Am. Chem. Soc., 1951, 73, 82–87 CrossRef CAS.
- D. C. Bilehal, R. M. Kulkarni and S. T. Nandibewoor, Inorg. React. Mech., 2002, 4, 103–109 CrossRef CAS.
- D. L. Kamble and S. T. Nandibewoor, J. Phys. Org. Chem., 1998, 11, 171–176 CrossRef CAS.
- K. S. Rangappa, M. P. Raghavendra, D. S. Mahadevappa and D. Channegouda, J. Org. Chem., 1998, 63, 531–536 CrossRef CAS.
-
A. Weissberger, Techniques of Chemistry, ed. E. S. Lewis, Investigation of Rates and Mechanism of Reactions in Techniques of Chemistry, Wiley, New York, 1974, vol. 6, pp. 421–427 Search PubMed.
-
K. J. Laidler, Chemical Kinetics, McGraw-Hill Inc., New York, 2nd edn, 1991, p. 237 Search PubMed.
- S. A. Farokhi and S. T. Nandibewoor, Tetrahedron, 2003, 59, 7595–7602 CrossRef CAS.
- M. Martinez, M. A. Pitarque and R. V. Eldik, J. Chem. Soc., Dalton Trans., 1996, 2665–2671 RSC.
|
This journal is © The Royal Society of Chemistry 2012 |
Click here to see how this site uses Cookies. View our privacy policy here.