Metal–organic frameworks for catalysis: the Knoevenagel reaction using zeolite imidazolate framework ZIF-9 as an efficient heterogeneous catalyst
Received
22nd September 2011
, Accepted 15th November 2011
First published on 1st December 2011
Abstract
Crystalline zeolite imidazolate framework ZIF-9 was synthesized from the reaction of cobalt nitrate hexahydrate and benzimidazole by a solvothermal method, and was used as an efficient heterogeneous catalyst for the low temperature liquid phase Knoevenagel reaction. The solid catalyst was characterized by various techniques, including X-ray powder diffraction (XRD), scanning electron microscopy (SEM), transmission electron microscopy (TEM), dynamic laser light scattering (DLS), thermogravimetric analysis (TGA), Fourier transform infrared spectroscopy (FT-IR), atomic absorption spectrophotometry (AAS), and nitrogen physisorption measurements. Excellent conversions were achieved under mild conditions. The ZIF-9 catalyst was easily isolated from the reaction mixture by simple filtration and reused several times without a significant degradation in activity. Moreover, there was no contribution from leached active species and conversion was only being possible in the presence of the solid catalyst.
1. Introduction
Metal–organic frameworks (MOFs) have been identified as potential materials for applications in gas separation and storage, sensors, drug delivery, and catalysis.1–5 Zeolite imidazolate frameworks (ZIFs), being considered as a new subclass of MOFs, have currently attracted significant attention as they combine highly desirable properties from both zeolites and conventional MOFs.6,7 ZIFs are extended three-dimensional structures constructed from tetrahedral metal atoms (e.g., Zn, Co, Cu) bridged by imidazolate linkers,6 producing nanosized pores formed by four, six, eight, and twelve membered ring ZnN4, CoN4, or CuN4 tetrahedral clusters.7,8 Several organic transformations have been carried out in the presence of conventional MOFs as solid catalysts or catalyst supports9 such as cycloaddition of CO2 with epoxides,10 cyanosilylation,11,12 oxidation,13–17 alkene epoxidation,18–20 hydrogenation,21 Suzuki cross-coupling,22 Sonogashira reaction,23 transesterification reaction,24 Friedel–Crafts alkylation,25,26 Knoevenagel condensation,27,28 aldol condensation,29,30 aza-Michael condensation,31 1,3-dipolar cycloaddition reactions,32 and epoxide ring-opening reaction.33–35 However, since the discovery of the first ZIF sample, research works have been mostly focused on preparing new ZIFs and investigating their applications in gas adsorption and separation.15 Indeed, reports on organic transformations using ZIFs as catalysts or catalyst supports have been very limited in the literature.15,36–41
The Knoevenagel reaction of aldehydes with compounds containing activated methylene groups has been widely employed in the synthesis of several fine chemicals42 as well as heterocyclic compounds of biological significance.43 This reaction is conventionally catalyzed by alkali metal hydroxides or by organic bases like primary, secondary and tertiary amines under homogeneous conditions with attendant difficulties in catalyst recovery and recycling.44 Over the last few years, a wide range of solid catalysts have been investigated for this reaction such as amino-functionalized mesoporous silica,45 diamine-functionalized mesopolymers,46 amine-functionalized mesoporous zirconia,47 superparamagnetic mesoporous Mg–Fe bi-metal oxides,48 mesoporous titanosilicate,49 basic MCM-41 silica,50–52 acid–base bifunctional mesoporous MCM-41 silica,53 nanocrystalline ceria–zirconia,54 zeolites exchanged with alkylammonium cations,55 amine-functionalized superparamagnetic nanoparticles,56 chitosan hydrogel,57 acrylic resin immobilized lipase,58 organic–inorganic hybrid silica materials containing imidazolium and dihydroimidazolium salts,59 IRMOF-3,28 and ZIF-8.38 In this work, we wish to report the utilization of zeolite imidazolate framework ZIF-9 as an efficient heterogeneous catalyst for the low temperature liquid phase Knoevenagel reaction. High activity was observed and the ZIF-9 catalyst was easily isolated from the reaction mixture by simple filtration and reused without a significant degradation in activity.
2. Experimental
2.1. Materials and instrumentation
All reagents and starting materials were obtained commercially from Sigma-Aldrich and Merck, and were used as received without any further purification unless otherwise noted. Nitrogen physisorption measurements were conducted using a Quantachrome 2200e system. Samples were pretreated by heating under vacuum at 150 °C for 3 h. A Netzsch Thermoanalyzer STA 409 was used for thermogravimetric analysis (TGA) with a heating rate of 10 °C min−1 from 30 to 900 °C under a nitrogen atmosphere. X-Ray powder diffraction (XRD) patterns were recorded using a Cu Kα radiation source on a D8 Advance Bruker powder diffractometer. Scanning electron microscopy studies were conducted on a JSM 740 Scanning Electron Microscope (SEM). Transmission electron microscopy studies were performed using a JEOL JEM 1400 Transmission Electron Microscope (TEM) at 100 kV. The ZIF-9 samples were dispersed on holey carbon grids for TEM observation. The particle size distribution of the ZIF-9 was determined by a dynamic laser light scattering (DLS) method using a LA 920. Elemental analysis with atomic absorption spectrophotometry (AAS) was performed on an AA-6800 Shimadzu. Fourier transform infrared (FT-IR) spectra were obtained on a Bruker TENSOR37 instrument, with samples being dispersed on potassium bromide pallets.
Gas chromatographic (GC) analyses were performed using a Shimadzu GC 17-A equipped with a flame ionization detector (FID) and a DB-5 column (length = 30 m, inner diameter = 0.25 mm, and film thickness = 0.25 μm). The temperature program for GC analysis heated samples from 60 to 200 °C at 20 °C min−1 and held them at 150 °C for 1 min; then heated them from 150 to 160 °C at 1 °C min−1 and held them at 200 °C for 2 min; then heated them from 200 to 300 °C at 50 °C min−1 and held them at 300 °C for 4 min. Inlet and detector temperatures were set constant at 300 °C. n-Dodecane was used as an internal standard to calculate reaction conversions. GC-MS analyses were performed using a Hewlett Packard GC-MS 5972 with a RTX-5MS column (length = 30 m, inner diameter = 0.25 mm, and film thickness = 0.5 μm). The temperature program for GC-MS analysis heated samples from 60 to 280 °C at 10 °C min−1 and held them at 280 °C for 2 min. Inlet temperature was set constant at 280 °C. MS spectra were compared with the spectra gathered in the NIST library.
2.2. Synthesis of ZIF-9
In a typical preparation,60 a solid mixture of cobalt nitrate hexahydrate (2.10 g, 7.21 × 10−3 mol) and benzimidazole (H-PhIM) (0.600 g, 5.08 × 10−3 mol) was dissolved in 180 ml of N,N′-dimethylformamide (DMF), and then distributed in 20 ml vials. The vials were tightly capped and heated at a rate of 5 °C min−1 to 140 °C in a programmable oven and held at this temperature for 48 h, then cooled at a rate of 0.4 °C min−1 to room temperature. After removal of mother liquor from the mixture, chloroform (20 ml) was added to each of the vials. Purple cubic crystals were collected from the upper chloroform layer, washed with DMF (3 × 15 ml) for 3 days. After that, the DMF was exchanged by dichloromethane (DCM) (3 × 15 ml) for 3 days. The residual solvents were removed under vacuum at 200 °C for 6 h, yielding 0.25 g of purple cubic crystals (25% based on benzimidazole).
2.3. Catalytic studies
The Knoevenagel reaction between benzaldehyde and malononitrile using the ZIF-9 catalyst was carried out in a magnetically stirred round bottom flask. Unless otherwise stated, a mixture of ZIF-9 (0.028 g, 5 mol%), benzaldehyde (0.2 ml, 1.9 mmol), and n-dodecane (0.13 ml, 0.58 mmol) as an internal standard was placed into a 25 ml flask containing 4 ml toluene. The catalyst concentration was calculated with respect to the cobalt/benzaldehyde molar ratio. The reactants in the vessel were stirred for 5 min to disperse the ZIF-9 in the liquid phase. A solution of malononitrile (0.25 g, 3.8 mmol) in toluene (1 ml) was then added, and the resulting mixture was stirred at room temperature for 6 h. Reaction conversion was monitored by withdrawing aliquots from the reaction mixture at different time intervals, quenching with acetone, filtering through a short silica gel pad, analyzing by GC with reference to n-dodecane, and further confirming product identity by GC-MS. The ZIF-9 catalyst was separated from the reaction mixture by simple centrifugation, washed with copious amounts of anhydrous toluene and dichloromethane, dried under vacuum at 200 °C for 6 h, and reused when necessary. For the leaching test, a catalytic reaction was stopped after 2 h, analyzed by GC, and centrifuged to remove the solid catalyst. The reaction solution was then stirred for a further 4 h. Reaction progress, if any, was monitored by GC as previously described.
3. Results and discussion
3.1. Catalyst characterization
In this work, the ZIF-9 was synthesized using cobalt nitrate hexahydrate and benzimidazole by a solvothermal method in DMF, according to a literature procedure.60 The ZIF-9 was then characterized using a variety of different techniques. A cobalt loading of 4.17 mmol g−1 was found for the ZIF-9, as indicated by elemental analysis with AAS. The particle size distribution of the ZIF-9 was observed from the DLS analysis, indicating an average size of 215 μm. As expected, the SEM micrograph showed that a crystalline material was achieved (Fig. 1). Very sharp peaks were observed on the XRD diffractogram of the ZIF-9, confirming the formation of a highly crystalline material using the previously-described synthesis procedure (Fig. 2). The TEM micrograph indicated that the as-synthesized ZIF-9 possessed a porous structure (Fig. 3). However, SEM and TEM results appeared to suggest that the pore structure of the ZIF-9 was complex, containing both microporous and mesoporous pores. FT-IR spectra of the ZIF-9 revealed a significant difference as compared to that of benzimidazole (Fig. 4). A strong and broad band, ranging from 3400 cm−1 to 2200 cm−1 with the maximum at approximately 2800 cm−1 was observed in the FT-IR spectra of benzimidazole, indicating the presence of the N–H⋯N hydrogen bond.61,62 The significant feature observed for the FT-IR spectra of the ZIF-9 as compared to those of benzimidazole was the disappearance of these absorption bands, proving that the benzimidazole linkers were fully deprotonated during the formation of the ZIF-9 structure.
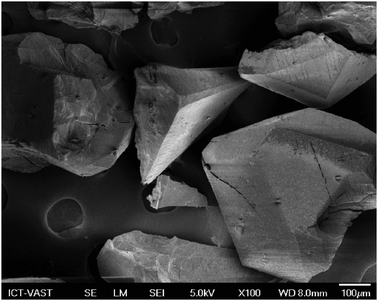 |
| Fig. 1 SEM micrograph of the ZIF-9. | |
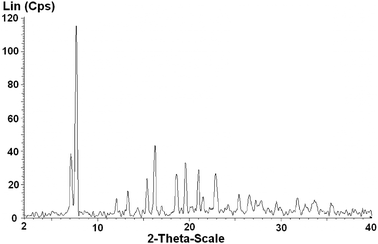 |
| Fig. 2 X-Ray powder diffractogram of the ZIF-9. | |
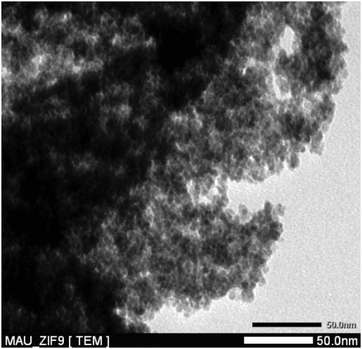 |
| Fig. 3 TEM micrograph of the ZIF-9. | |
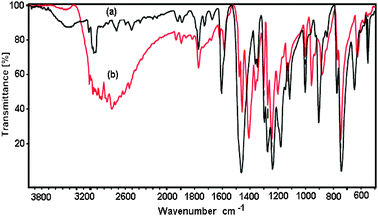 |
| Fig. 4 FT-IR spectra of the ZIF-9 (a) and benzimidazole (b). | |
The most significant feature observed in the TGA result of the ZIF-9 was that little weight loss was found in the temperature range of 200–500 °C (Fig. 5). This result also revealed that the occluded DMF in the as-synthesized ZIF-9 sample was fully exchanged with DCM, which was completely removed under vacuum in the activation step. A sharp weight-loss step of over 40% was observed in the TGA result as the temperature increased to over 500 °C, showing that the ZIF-9 decomposed in that temperature range. It was also observed that approximately 53% of the starting weight remained after the decomposition at 900 °C. This thermal stability behavior showed that the ZIF-9 could be used across a wide temperature range. MOF-based materials have been considered as promising materials for applications in catalysis, separation, and gas storage because of their exceptionally high adsorption surface areas.1–3 In this work, it was found that the ZIF-9 could afford Langmuir surface areas of up to 1144 m2 g−1. It should be noted that the largest surface areas of ordered structures such as zeolites or silicas were observed at around 1000 m2 g−1. This property would make ZIF-9 an attractive candidate for applications in catalysis.
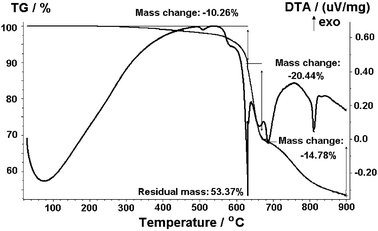 |
| Fig. 5 TGA analysis of the ZIF-9. | |
3.2. Catalytic studies
The ZIF-9 catalyst was assessed for its activity in the Knoevenagel reaction by studying the condensation of benzaldehyde with malononitrile to form benzylidene malononitrile as the principal product (Scheme 1). Chizallet and co-workers previously investigated the presence of acido-basic sites in the ZIF-8 by combining CO adsorption monitored by FT-IR and DFT calculations, and confirmed that some strong Lewis acid sites (in particular ZnII species) and strong Bronsted acid sites (NH groups), together with basic ones, existed on the surface of the catalyst. Furthermore, they demonstrated that all active sites are located at the external surface, but not in the microporosity of the material.37 As the pore diameter as well as the pore windows of the ZIF-9 are even smaller than those of the ZIF-8, it is apparent that reactions using bulky molecules should also occur on the external surface of the ZIF-9 particles. Indeed, Corma, Weckhuysen and their co-workers recently employed the ZIF-9 (a ZIF based on benzimidazole and cobalt) as a catalyst for oxidation reactions, and also reported that the reactions occurred on the exterior surface of the catalyst.14,39 It was therefore inferred that the Knoevenagel reaction of benzaldehyde and malononitrile should also occur on the external surface of the ZIF-9 particles. It should be noted that organic transformations based on the acido-basicity properties of the ZIF-9 were not previously mentioned in the literature.
 |
| Scheme 1 Knoevenagel reaction of benzaldehyde with malononitrile using the ZIF-9 catalyst. | |
The Knoevenagel reaction between benzaldehyde and malononitrile was carried out using 5 mol% ZIF-9 catalyst relative to benzaldehyde in toluene. As required by green chemistry principles, it was decided to carry out the reaction under room temperature conditions. Aliquots were withdrawn from the reaction mixture at different time intervals and analyzed by GC, giving kinetic data during the course of the reaction. The reagent ratio is normally an important factor that should be taken into consideration in the case of the Knoevenagel condensation. The effect of the benzaldehyde
:
malononitrile molar ratio on reaction conversions was therefore investigated, having carried out the reaction at molar ratios of 1
:
1, 1
:
2, 1
:
3, and 1
:
4, respectively. Experimental results showed that 99% conversion was obtained after 4 h for the reaction using the reagent molar ratio of 1
:
3. The reaction at the reagent molar ratio of 1
:
4 occurred more readily. However, it was apparent that increasing the molar ratio from 1
:
3 to 1
:
4 would be unnecessary as the reaction rate was not significantly enhanced. As expected, decreasing the malononitrile
:
benzaldehyde molar ratio to 1
:
2 resulted in a significant drop in the reaction rate, though a conversion of 99% was still achieved after 6 h. The reaction using one equivalent of malononitrile proceeded slowly, with only 49% conversion being observed after 6 h (Fig. 6).
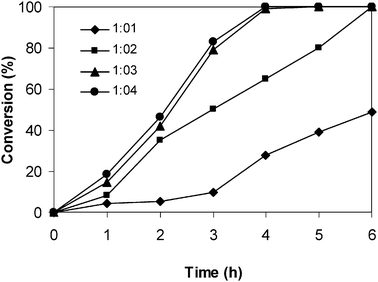 |
| Fig. 6 Effect of benzaldehyde : malononitrile molar ratio on reaction conversion. | |
With this result in mind, we then decided to investigate the effect of catalyst concentration on the reaction conversion, having carried out the reaction at room temperature using the reagent molar ratio of 1
:
3. As demonstrated by Chizallet and co-workers for the case of ZIF-8,37 a variety of acido-basicity active sites should also be present on the surface of the ZIF-9. For the reason of simplicity, cobalt content was used as an elemental tag for the catalyst. However, it should be noted that further investigations would be needed to elucidate the real reactive site on the surface of the ZIF-9 catalyst for the Knoevenagel condensation. The catalyst concentration, with respect to the cobalt content in the ZIF-9, was then studied in the range of 1–5 mol% relative to benzaldehyde. It was observed that quantitative conversion of benzaldehyde was achieved after 4 h at 8 mol% catalyst, though the reaction rate was higher than in the case of 5 mol% catalyst. It was found that decreasing the catalyst concentration to 3 mol% led to a significant drop in the reaction rate, with 81% conversion being achieved after 6 h. As expected, a lower reaction rate was observed for the reaction using 1 mol% catalyst, though the reaction could afford 51% conversion after 6 h (Fig. 7). The results indicated that the ZIF-9 catalyst was quite active in the Knoevenagel reaction. Furthermore, it exhibited higher activity than some previously reported Lewis acid catalysts, where longer reaction time or/and higher catalyst loading were required for the same reaction.63 However, the ZIF-9 was slightly less active for the Knoevenagel condensation as compared to some solid base catalysts such as amine-functionalized superparamagnetic nanoparticles56 and amine-functionalized mesoporous zirconia.47 Indeed, it was previously reported that the catalyst concentration used for the Knoevenagel reaction could vary from less than 1 mol% to more than 10 mol%, depending on the nature of the catalyst.47,49,64
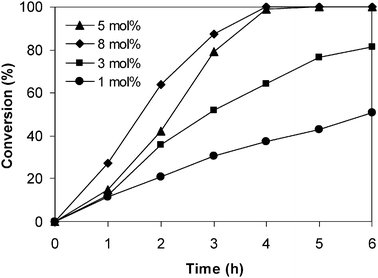 |
| Fig. 7 Effect of catalyst concentration on reaction conversion. | |
When a solid catalyst is employed for a liquid-phase organic transformation, an important problem that should be seriously considered is the possibility that some of the active sites could dissolve into the reaction solution. In some cases, these leached species could contribute significantly to the catalytic activity during the course of the reaction.56 Pande and co-workers previously reported that imidazole, 1-methylimidazole, and 2-methylimidazole could be used as homogenous base catalysts for the Knoevenagel condensation.65 However, it was apparent that these organic bases could not be recycled and reused for the reaction, and therefore they were practically undesirable. In this research, it was found that the homogeneous benzimidazole linker exhibited higher activity in the Knoevenagel reaction than the ZIF-9 catalyst, with quantitative conversion being obtained after 3 h. As expected, cobalt nitrate hexahydrate showed very low activity in the Knoevenagel condensation under similar reaction conditions, with only 11% conversion being observed after 6 h (Fig. 8). In order to determine if the benzimidazole linker leached from the solid ZIF-9 catalyst could play a significant role in the catalytic activity for the Knoevenagel reaction, an experiment was performed using a simple centrifugation during the course of the reaction. After the solid catalyst was removed, if the catalytic reaction continued this would indicate that the active species were from the solution rather than from the solid ZIF-9 catalyst. The organic phase was separated from the solid catalyst after 2 h reaction time by simple centrifugation, having used 5 mol% of fresh ZIF-9 catalyst. The reaction solution was then transferred to a new reactor vessel, and stirred for an additional 4 h at room temperature with aliquots being sampled at different time intervals, and analyzed by GC. The data from GC determinations would show quantitative information about residual, catalytically active species in solution. Indeed, no further reaction was observed after the solid ZIF-9 catalyst was separated from the reaction mixture. This result indicated that the Knoevenagel reaction could only occur in the presence of the solid ZIF-9 catalyst, and there was no contribution from leached active species in the reaction solution (Fig. 8).
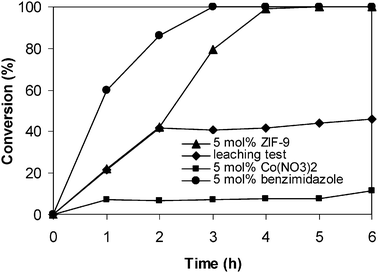 |
| Fig. 8 Leaching test indicated no contribution from homogeneous catalysis of active species leaching into reaction solution. | |
Different reaction rates could normally be observed for the reaction using solid catalysts carried out in different solvents, depending on the nature of the catalyst material.56,66 Macquarrie and Jackson previously reported that the Knoevenagel condensation using silica-based catalysts could proceed well in a very limited range of effective solvents, and the best reaction rate was usually achieved in nonpolar solvents.67 In contrast, Gascon and co-workers demonstrated that nonpolar solvents were not suitable for the Knoevenagel condensation using a MOF-based catalyst, while polar solvents were reported to favor the reaction rate.28,68 Similar results were observed by Corma and co-workers, in which the reaction occurred with difficulty in nonpolar solvents and higher conversions were achieved in more polar solvents.69–71 Moreover, it was also reported that the higher the hydrophobicity of the catalyst, the less the effect of the solvent on the reaction rate.28 It was therefore decided to investigate the solvent effect in the Knoevenagel reaction, using 5 mol% of the ZIF-9 catalyst at room temperature. Experimental results showed that the reaction rate of the Knoevenagel condensation using the ZIF-9 catalyst decreased in the order of solvents: tetrahydrofuran > toluene > ethyl acetate > dichloromethane. The reaction carried out in tetrahydrofuran proceeded readily to over 99% conversion after 2 h with no trace amount of benzaldehyde being detected by GC, while the reaction in toluene required up to 4 h to achieve a similar conversion. It was observed that the reaction carried out in ethyl acetate, a relatively polar solvent, proceeded with difficulty, though 88% conversion was still afforded after 6 h. Dichloromethane was found to be unsuitable for the Knoevenagel reaction using the ZIF-9 catalyst (Fig. 9).
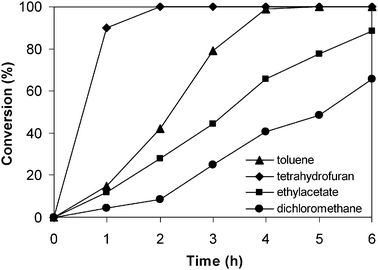 |
| Fig. 9 Effect of solvent on reaction conversion. | |
From an experimental point of view, it should be noted that when tetrahydrofuran was used as the reaction solvent, the ZIF-9 crystals were broken into smaller particles during the course of the reaction. As the Knoevenagel reaction occurred on the exterior surface of the catalyst,14,39 smaller particles would exhibit higher catalytic activity due to the increased external surface of smaller crystals.38,72 This phenomenon should be one of the reasons leading to the high conversion observed for the reaction carried out in tetrahydrofuran. It was previously reported that nonpolar solvents were advantageous for hydrophilic silica-based catalysts in the Knoevenagel condensation.67,73 Although ZIF-9 was hydrophobic like other unmodified ZIF structures,60,74,75 the reaction using this catalyst also afforded high conversions in toluene. Aguado and co-workers recently demonstrated that the hydrophilic–hydrophobic balance of MOF-based materials exhibited a significant effect on the catalytic activity in the Knoevenagel condensation.76
The activity of the ZIF-9 catalyst in the Knoevenagel reaction using different solvents was then compared to those of ZIF-8 (the linker is 2-methylimidazole), and ZIF-10 (the linker is imidazole). The ZIF-8 and the ZIF-10 were synthesized and characterized according to a literature procedure.60 It was observed that a higher reaction rate was achieved for the less hydrophobic catalyst, and the reaction rate decreased in the order: ZIF-10 > ZIF-8 > ZIF-9 (Fig. 10). A higher reaction rate in toluene was obtained in three cases, as compared to that of the reaction carried out in ethylacetate. One of the reasons leading to the difference in the reaction rate in these two solvents might be rationalized based on the fact that the more polar ethylacetate could encourage the polar reactants to leave the more hydrophobic catalyst and move into the bulk reaction media. However, the issue still needs further investigation.
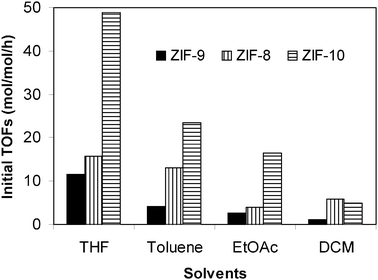 |
| Fig. 10 Initial TOFs of the Knoevenagel reaction in different solvents using ZIF-9, ZIF-8, and ZIF-10 as catalyst, respectively. | |
When using solid catalysts for organic transformations, issues that should be taken into consideration are the ease of separation as well as the deactivation and reusability of the catalyst. The ZIF-9 catalyst was therefore investigated for recoverability and reusability in the Knoevenagel reaction over five successive runs. The reaction was carried out in tetrahydrofuran at room temperature using 5 mol% of ZIF-9 catalyst for 6 h. After the reaction, the catalyst was separated by simple centrifugation, then washed with copious amounts of tetrahydrofuran and dichloromethane to remove any physisorbed reagents, and dried under vacuum at 200 °C for 6 h. The recovered ZIF-9 catalyst was reused in further reaction under identical conditions to those of the first run. It was observed that over 99% conversions were achieved in the 1st, the 2nd and the 3rd runs, with no trace amount of benzaldehyde being detected by GC. However, the reaction conversion decreased to 97% and 93% in the 4th and the 5th run, respectively (Fig. 11a). Although the reaction conversions remained unchanged over the first three runs, kinetic studies indicated that the catalytic activity of the ZIF-9 in the Knoevenagel reaction decreased slightly after one use (Fig. 11b). Conversions of 90%, 86%, and 53% were observed after 1 h for the 1st, the 2nd and the 3rd run, respectively. FT-IR spectra of the ZIF-9 after the 1st run indicated a slight difference as compared to that of the fresh catalyst (Fig. 12). Moreover, XRD results of the reused catalyst revealed that the ZIF-9 could maintain its crystallinity during the course of the reaction. However, a difference in the overall structure was observed for the reused ZIF-9 (Fig. 13). Further studies would be needed to elucidate the reason for catalyst deactivation in the Knoevenagel reaction using the ZIF-9 catalyst.
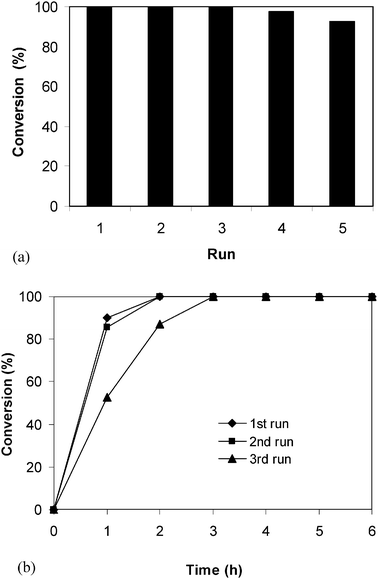 |
| Fig. 11 Catalyst recycling studies. | |
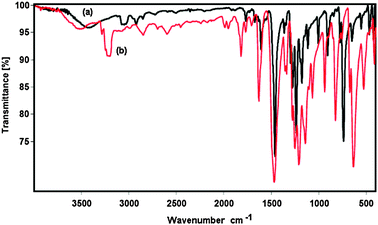 |
| Fig. 12 FT-IR spectra of the reused (a) and fresh (b) ZIF-9. | |
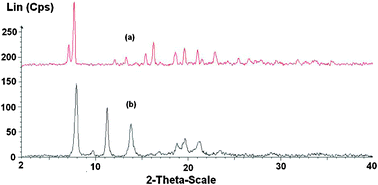 |
| Fig. 13 X-Ray powder diffractogram of the fresh (a) and reused (b) ZIF-9. | |
The study was then extended to the Knoevenagel condensation of several benzaldehyde derivatives having different substituents with malononitrile using the ZIF-9 catalyst. Reactions were carried out in tetrahydrofuran at room temperature using 5 mol% ZIF-9 catalyst loading with aliquots being sampled at different time intervals, and analyzed by GC. Experimental results showed that a clear relationship between the reaction rate and the Hammett constant was not observed for the Knoevenagel condensation using the ZIF-9 catalyst. The reaction of 4-methylbenzaldehyde and 2-methylbenzaldehyde, respectively, with malononitrile proceeded readily in the presence of the ZIF-9 catalyst to give quantitative conversions after 2 h. It should be noted that the reaction rate of benzaldehyde derivatives with malononitrile is normally accelerated in the presence of electron-withdrawing substituents.77 Although methyl is an electron-donating group, both 4-methylbenzaldehyde and 2-methylbenzaldehyde were found to be more reactive than the benzaldehyde. Interestingly, it was observed that 3-methylbenzaldehyde was significantly less reactive than the corresponding 2- and the 4-isomers, and the benzaldehyde. However, the Knoevenagel condensation of 3-methylbenzaldehyde with malononitrile using the ZIF-9 catalyst could still afford more than 99% conversion after 6 h. The Knoevenagel reaction of 4-nitrobenzaldehyde and 4-chlorobenzaldehyde also proceeded facilely, with quantitative conversion being obtained within 1 h (Fig. 14). Indeed, Liu and co-workers recently reported that high conversions were achieved in the Knoevenagel reactions of both benzaldehyde derivatives containing electron-withdrawing and those having electron-donating substituents.78
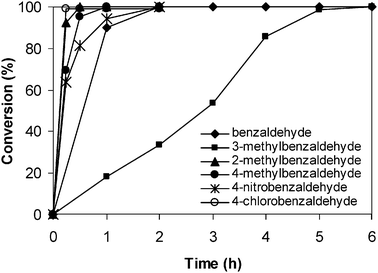 |
| Fig. 14 Effect of different substituents on reaction conversion. | |
4. Conclusions
In conclusion, crystalline zeolite imidazolate framework ZIF-9 was successfully synthesized from the reaction of cobalt nitrate hexahydrate and benzimidazole by a solvothermal method in DMF. The ZIF-9 was characterized using a variety of different techniques, including FT-IR, TEM, SEM, XRD, TGA, AAS, DLS, and nitrogen physisorption measurements. The ZIF-9 was used as an efficient heterogeneous catalyst for the Knoevenagel reaction between benzaldehyde and malononitrile to form benzylidene malononitrile as the principal product. Excellent conversions were achieved under mild conditions without the need for an inert atmosphere. The ZIF-9 catalyst could be reused several times without a significant degradation in catalytic activity. The reaction could only occur in the presence of the solid ZIF-9 catalyst, and there was no contribution from leached active species in the reaction solution. It is apparent that the ZIF-9 catalyst can be an alternative to other solid catalysts for the Knoevenagel reaction. The interesting properties of the ZIF-based material offer potential advantages over conventional catalysts, and would be interesting to the chemical industry. Current research in our laboratory has focused on the design and synthesis of several ZIF-based catalysts for a wide range of organic transformations.
Acknowledgements
The Ho Chi Minh City Department of Science and Technology (Viet Nam) is acknowledged for financial support.
References
- H. K. Chae, D. Y. Siberio-Perez, J. Kim, Y. Go, M. Eddaoudi, A. J. Matzger, M. O'Keeffe and O. M. Yaghi, Nature, 2004, 427, 523–527 CrossRef CAS.
- D. J. Tranchemontagne, M. O. k. Z. Ni and O. M. Yaghi, Angew. Chem., Int. Ed., 2008, 47, 5136–5147 CrossRef CAS.
- S. S. Kaye, A. Dailly, O. M. Yaghi and J. R. Long, J. Am. Chem. Soc., 2007, 129, 14176–14177 CrossRef CAS.
- H. Furukawa, N. Ko, Y. B. Go, N. Aratani, S. B. Choi, E. Choi, A. O. Yazaydin, R. Q. Snurr, M. O'Keeffe, J. Kim and O. M. Yaghi, Science, 2010, 239, 424–428 CrossRef.
- P. Horcajada, T. Chalati, C. Serre, B. Gillet, C. Sebrie, T. Baati, J. F. Eubank, D. Heurtaux, P. Clayette, C. Kreuz, J. S. Chang, Y. K. Hwang, V. Marsaud, P. N. Bories, L. Cynober, S. Gil, G. Fe′rey, P. Couvreur and R. Gref, Nat. Mater., 2010, 9, 172–178 CrossRef CAS.
- A. Phan, C. J. Doonan, F. J. Uribe-Romo, C. B. Knobler, M. O'Keeffe and O. M. Yaghi, Acc. Chem. Res., 2010, 43, 58–67 CrossRef CAS.
- S. R. Venna and M. A. Carreon, J. Am. Chem. Soc., 2010, 132, 76–78 CrossRef CAS.
- R. Banerjee, A. Phan, B. Wang, C. Knobler, H. Furukawa, M. O'Keeffe and O. M. Yaghi, Science, 2008, 319, 939–943 CrossRef CAS.
- A. Dhakshinamoorthy, M. Alvaro, A. Corma and H. Garcia, Dalton Trans., 2011, 40, 6344–6360 RSC.
- J. Song, Z. Zhang, S. Hu, T. Wu, T. Jiang and B. Han, Green Chem., 2009, 11, 1031–1036 RSC.
- K. Schlichte, T. Kratzke and S. Kaskel, Microporous Mesoporous Mater., 2004, 73, 81–88 CrossRef CAS.
- M. Gustafsson, A. Bartoszewicz, B. Martn-Matute, J. Sun, J. Grins, T. Zhao, Z. Li, G. Zhu and X. Zou, Chem. Mater., 2010, 22, 3316–3322 CrossRef CAS.
- A. Dhakshinamoorthy, M. Alvaro and H. Garcia, ACS Catal., 2011, 1, 48–53 CrossRef CAS.
- F. X. L. i. Xamena, O. Casanova, R. G. Tailleur, H. Garcia and A. Corma, J. Catal., 2008, 255, 220–227 CrossRef.
- H. Liu, Y. Liu, Y. Li, Z. Tang and H. Jiang, J. Phys. Chem. C, 2010, 114, 13362–13369 CAS.
- W. Kleist, M. Maciejewski and A. Baiker, Thermochim. Acta, 2010, 499, 71–78 CrossRef CAS.
- A. Dhakshinamoorthy, M. Alvaro and H. Garcia, J. Catal., 2009, 267, 1–4 CrossRef CAS.
- F. Song, C. Wang, J. M. Falkowski, L. Ma and W. Lin, J. Am. Chem. Soc., 2010, 132, 15390–15398 CrossRef CAS.
- S.-H. Cho, B. Ma, S. T. Nguyen, J. T. Hupp and T. E. Albrecht-Schmitt, Chem. Commun., 2006, 2563–2565 RSC.
- K. Brown, S. Zolezzi, P. Aguirre, D. Venegas-Yazigi, V. Paredes-García, R. Baggio, M. A. Novak and E. Spodine, Dalton Trans., 2009, 1422–1427 RSC.
- S. Opelt, S. Turk, E. Dietzsch, A. Henschel, S. Kaskel and E. Klemm, Catal. Commun., 2008, 9, 1286–1290 CrossRef CAS.
- F. X. L. i. Xamena, A. Abad, A. Corma and H. Garcia, J. Catal., 2007, 250, 294–298 CrossRef.
- S. Gao, N. Zhao, M. Shu and S. Che, Appl. Catal., A, 2010, 388, 196–201 CrossRef CAS.
- Y. Zhou, J. Song, S. Liang, S. Hu, H. Liu, T. Jiang and B. Han, J. Mol. Catal. A: Chem., 2009, 308, 68–75 CrossRef CAS.
- N. T. S. Phan, K. K. A. Le and T. D. Phan, Appl. Catal., A, 2010, 382, 246–253 CrossRef CAS.
- U. Ravon, M. Savonnet, S. Aguado, M. E. Domine, E. Janneau and D. Farrusseng, Microporous Mesoporous Mater., 2010, 129, 319–329 CrossRef CAS.
- S. Neogi, M. K. Sharma and P. K. Bharadwaj, J. Mol. Catal. A: Chem., 2009, 299, 1–4 CrossRef CAS.
- J. Gascon, U. Aktay, M. D. Hernandez-Alonso, G. P. M. v. Klink and F. Kapteijn, J. Catal., 2009, 261, 75–87 CrossRef CAS.
- T. Dewa, T. Saiki and Y. Aoyama, J. Am. Chem. Soc., 2001, 123, 502–503 CrossRef CAS.
- F. Vermoortele, R. Ameloot, A. Vimont, C. Serre and D. D. Vos, Chem. Commun., 2011, 47, 1511–1523 RSC.
- M. Savonnet, S. Aguado, U. Ravon, D. Bazer-Bachi, V. Lecocq, N. Bats, C. Pinel and D. Farrusseng, Green Chem., 2009, 11, 1729–1732 RSC.
- I. Luz, F. X. L. i. Xamena and A. Corma, J. Catal., 2010, 276, 134–140 CrossRef CAS.
- K. Tanaka, S. Oda and M. Shiro, Chem. Commun., 2008, 820–822 RSC.
- K. K. Tanabe and S. M. Cohen, Inorg. Chem., 2010, 49, 6766–6774 CrossRef CAS.
- A. Corma, H. García and F. X. L. s. i. Xamena, Chem. Rev., 2010, 110, 4606–4655 CrossRef CAS.
- H.-L. Jiang, B. Liu, T. Akita, M. Haruta, H. Sakurai and Q. Xu, J. Am. Chem. Soc., 2009, 131, 11302–11303 CrossRef CAS.
- C. l. Chizallet, S. Lazare, D. Bazer-Bachi, F. Bonnier, V. Lecocq, E. Soyer, A.-A. Quoineaud and N. Bats, J. Am. Chem. Soc., 2010, 132, 12365–12377 CrossRef CAS.
- U. P. N. Tran, K. K. A. Le and N. T. S. Phan, ACS Catal., 2011, 1, 120–127 CrossRef CAS.
- J. Zakzeski, A. Debczak, P. C. A. Bruijnincx and B. M. Weckhuysen, Appl. Catal., A, 2011, 394, 79–85 CrossRef CAS.
- S. B. Kalidindi, D. Esken and R. A. Fischer, Chem.–Eur. J., 2011, 17, 6594–6597 CrossRef CAS.
- A. Sonia, C. Jerome and F. David, Chem. Commun., 2010, 46, 7999–8001 RSC.
- F. Freeman, Chem. Rev., 1980, 80, 329–350 CrossRef CAS.
- L. F. Tietze, Chem. Rev., 1996, 96, 115–136 CrossRef CAS.
-
D. J. Macquarrie, D. B. Jackson and J. H. Clark, Organic modification of hexagonal mesoporous silicas, in Supported Catalysts and Their Application, ed. D. C. Sherrington and A. P. Kybett, RSC, Cambridge, 2001 Search PubMed.
- J. Mondal, A. Modak and A. Bhaumik, J. Mol. Catal. A: Chem., 2011, 335, 236–241 CrossRef CAS.
- R. Xing, H. Wu, X. Li, Z. Zhao, Y. Liu, L. Chen and P. Wu, J. Mater. Chem., 2009, 19, 4004–4011 RSC.
- K. M. Parida, S. Mallick, P. C. Sahoo and S. K. Rana, Appl. Catal., A, 2010, 381, 226–232 CrossRef CAS.
- Z. Gao, J. Zhou, F. Cui, Z. H. Yan Zhu and J. Shi, Dalton Trans., 2010, 39, 11132–11135 RSC.
- B. Karmakar, B. Chowdhury and J. Banerji, Catal. Commun., 2010, 11, 601–605 CrossRef CAS.
- F. Shang, J. Sun, S. Wu, Y. Yang, Q. Kan and J. Guan, Microporous Mesoporous Mater., 2010, 134, 44–50 CrossRef CAS.
- K. M. Parida and D. Rath, J. Mol. Catal. A: Chem., 2009, 310, 93–100 CrossRef CAS.
- L. Martins, W. Hölderich, P. Hammer and D. Cardoso, J. Catal., 2010, 271, 220–227 CrossRef CAS.
- F. Shang, J. Sun, S. Wu, Y. Yang, Q. Kan and J. Guan, Microporous Mesoporous Mater., 2010, 134, 44–50 CrossRef CAS.
- G. Postole, B. Chowdhury, B. Karmakar, K. Pinki, J. Banerji and A. Auroux, J. Catal., 2010, 269, 110–121 CrossRef CAS.
- L. Martins, K. M. Vieira, L. M. Rios and D. Cardoso, Catal. Today, 2008, 133, 706–710 CrossRef.
- N. T. S. Phan and C. W. Jones, J. Mol. Catal. A: Chem., 2006, 253, 123–131 CrossRef CAS.
- K. R. Reddy, K. Rajgopal, C. U. Maheswari and M. L. Kantam, New J. Chem., 2006, 30, 1549–1552 RSC.
- X.-W. Feng, C. Li, N. Wang, K. Li, W.-W. Zhang, Z. Wang and X.-Q. Yu, Green Chem., 2009, 11, 1933–1936 RSC.
- M. Trilla, R. Pleixats, M. W. C. Man and C. Bied, Green Chem., 2009, 11, 1815–1820 RSC.
- K. S. Park, Z. Ni, A. P. Côté, J. Y. Choi, R. D. Huang, F. J. Uribe-Romo, H. K. Chae, M. O'Keeffe and O. M. Yaghi, Proc. Natl. Acad. Sci. U. S. A., 2006, 103, 10186–10191 CrossRef CAS.
- B. Hachuła, M. Nowak and J. Kusz, J. Chem. Crystallogr., 2010, 40, 201–206 CrossRef.
- S. Mohan and N. Sundaraganesan, Spectrochim. Acta, 1991, 47A, 1111–1115 CrossRef CAS.
- K. Yamashita, T. Tanaka and M. Hayashi, Tetrahedron Lett., 2005, 61, 7981–7985 CAS.
- Y. Kubota, Y. Nishizaki, H. Ikeya, M. Saeki, T. Hida, S. Kawazu, M. Yoshida, H. Fujii and Y. Sugi, Microporous Mesoporous Mater., 2004, 70, 135–149 CrossRef CAS.
- A. Pande, K. Ganesan, A. K. Jain, P. K. Gupta and R. C. Malhotra, Org. Process Res. Dev., 2005, 9, 133–136 CrossRef CAS.
- G. Langhendries, D. E. D. Vos, G. V. Baron and P. A. Jacobs, J. Catal., 1997, 187, 453–463 CrossRef.
- D. J. Macquarrie and D. B. Jackson, Chem. Commun., 1997, 1781–1782 RSC.
- J. Juan-Alcañiz, E. V. Ramos-Fernandez, U. Lafont, J. Gascon and F. Kapteijn, J. Catal., 2010, 269, 229–241 CrossRef.
- I. Rodriguez, G. Sastre, A. Corma and S. Iborra, J. Catal., 1999, 183, 14–23 CrossRef CAS.
- A. Corma, S. Iborra, I. Rodriguez and F. Sanchez, J. Catal., 2002, 211, 208–215 CAS.
- M. J. Climent, A. Corma, I. Domínguez, S. Iborra, M. J. Sabater and G. Sastre, J. Catal., 2007, 246, 136–146 CrossRef CAS.
- R. Selvin, H.-L. Hsu and T.-M. Her, Catal. Commun., 2008, 10, 169–172 CrossRef CAS.
- D. J. Macquarrie, J. H. Clark, A. Lambert, J. E. G. Mdoe and A. Priest, React. Funct. Polym., 1997, 35, 153–158 CrossRef CAS.
- D. Farrusseng, S. A. Dr and C. Pinel, Angew. Chem., Int. Ed., 2009, 48, 7502–7513 CrossRef CAS.
- H. Bux, F. Liang, Y. Li, J. Cravillon, M. Wiebcke and J. Caro, J. Am. Chem. Soc., 2009, 131, 16000–16001 CrossRef CAS.
- S. Aguado, J. Canivet and D. Farrusseng, J. Mater. Chem., 2011, 21, 7582–7588 RSC.
- R. Gupta, M. Gupta, S. Paul and R. Gupta, Bull. Korean Chem. Soc., 2009, 30, 2419–2421 CrossRef CAS.
- Q. Liu, H. Ai and Z. Li, Ultrason. Sonochem., 2011, 18, 477–479 CrossRef CAS.
|
This journal is © The Royal Society of Chemistry 2012 |