Pattern formation in the iodate–sulfite–thiosulfate reaction–diffusion system†
Received
13th July 2011
, Accepted 11th October 2011
First published on 8th November 2011
Abstract
Sodium polyacrylate-induced pH pattern formation and starch-induced iodine pattern formation were investigated in the iodate–sulfite–thiosulfate (IST) reaction in a one-side fed disc gel reactor (OSFR). As binding agents of the autocatalyst of hydrogen ions or iodide ions, different content of sodium polyacrylate or starch has induced various types of pattern formation. We observed pH pulses, striped patterns, mixed spots and stripes, and hexagonal spots upon increasing the content of sodium polyacrylate and observed iodine pulses, branched patterns, and labyrinthine patterns upon increasing the starch content in the system. Coexistence of a pH front and an iodine front was also studied in a batch IST reaction–diffusion system. Both pH and iodine front instabilities were observed in the presence of sodium polyacrylate, i.e., cellular fronts and transient Turing structures resulting from the decrease in diffusion coefficients of activators. The mechanism of multiple feedback may explain the different patterns in the IST reaction–diffusion system.
Introduction
Spatiotemporal patterns arising from the interaction of reaction and transport processes abound in nature.1,2 Explanation of the important characteristics of pattern formation in solution chemistry paves the way for better understanding the formation of complex biological analogues.1–3 Over the past two decades, much attention has been devoted to spatiotemporal pattern formation in chemical reaction–diffusion (RD) systems. Remarkable developments have been achieved since the first experimental discovery of Turing patterns in the chlorite–iodide–malonic acid (CIMA) system in 1990.4 Studies of sustained spiral waves have focused on the Belousov–Zhabotinsky (BZ) reaction both in batch and in spatial open reactors.5–7 The study of spatial stationary patterns, such as Turing patterns, has mostly focused on the CIMA reaction7,8 or the Belousov–Zhabotinsky reaction in AOT microemulsions.9 A variety of spatial patterns such as stationary labyrinthine patterns, self-replicating spots arising from front interactions and front instabilities were observed in the ferrocyanide–iodate–sulfite (FIS) reaction.10,11 In the CIMA reaction, starch or polyvinyl alcohol is introduced to reversibly form an unreactive, immobile complex with the activator of iodide ions, which reduces the effective diffusion coefficient of the activator, in order to fulfill the necessary conditions for development of the stationary patterns.12,13 A rich variety of spatial structures, such as hexagonal, striped, and mixed Turing patterns,8 has been observed in this system.
Even though a large number of chemically different bistable and oscillating reactions have been found, only a few have been shown to produce spatiotemporal waves and patterns. In the past years, De Kepper's group was dedicated to designing new chemical systems for pattern formation. For a comprehensive understanding of chemical patterns, they have made fundamental research on spatial bistability in several chemical systems such as the chlorine dioxide–iodide (CDI) reaction,14 the tetrathionate–chlorite (CT) reaction,15–17 the iodate–sulfite (Landolt) reaction,18 the bromate–sulfite (BS) reaction,19 the FIS reaction,20,21 and the thiourea–iodate–sulfite (TuIS) reaction.22,23 Spatial bistability arises from diverse reaction–diffusion instabilities24 and is a source of complex dynamics and a common phenomenon when an autocatalytic reaction is conducted in an OSFR. They also found spatiotemporal oscillations in some of the above reactions,18,20,23 which possess either pH-driven or iodide ion-driven autocatalysis.
Although a large variety of spatial patterns,20,21,25,26 such as self-replicating spots, bouncing waves, and stationary labyrinthine patterns, was found in the FIS system, it is not easy to observe these patterns in many other systems. Recently, an effective experimental method was designed to produce Turing patterns in the TuIS RD system22,23 in an OSFR by coupling self-activatory and inhibitory reactions along with controlling of the effective diffusion coefficient of the activator.
For an analogous Landolt-type reaction, the iodate–sulfite–thiosulfate (IST) reaction, which is autocatalytic with respect to both protons and iodide ions, shows complex pH oscillations and temperature oscillations in a continuous flow stirred tank reactor (CSTR)27–29 and produces pH waves and iodine waves in a Petri dish.30 In this paper, we focus on the pH and triiodide–starch complex pattern formation obtained by using sodium-polyacrylate as the hydrogen ion binding agent, bromocresol green as the pH indicator, and starch as the simultaneous binding agent and indicator, respectively. An experimental investigation of the individual structures, coexistence and instabilities of pH patterns and iodine patterns is presented.
Experimental section
1. CSTR experiments
Reagent grade chemicals were used without further purification, and all the stock solutions were prepared with deionized water (ultrafiltered from a Milli-Q system). The flow experiments were performed in a water-jacketed cylindrical-shaped glass CSTR with a liquid volume of 21 cm3. The pH signal in the CSTR was followed with a glass electrode coupled to an Hg|Hg2SO4|K2SO4 reference electrode and collected with a PC computer through a Powerlab/4sp system. The chemical solutions were stored in four separate reservoirs and injected by a high-precision peristaltic pump (ISMATIC IPC4) to the bottom of the CSTR, in close vicinity to a magnetic stirrer rotating at 800 rpm to ensure uniform mixing. The four reservoirs contained: (1) potassium iodate, (2) sodium thiosulfate, (3) sodium sulfite, (4) sulfuric acid. Solutions (1) and (2) entered the CSTR through independent ports, respectively. Feed streams (3) and (4) were premixed in a premixing head, just before their entry into the reactor, which effectively avoided local acidification.29
2. OSFR experiment
The experiments for pattern formation far from the equilibrium were performed in a one-side-fed reactor (OSFR) consisting of a gel part and a CSTR. The gel part consisted of a transparent thin gel disc made of 2 wt% agarose gel with 20 mm diameter and 0.5 mm thickness. One face of the disc was in contact with the contents of the CSTR through a cellulose nitrate membrane for providing a white background for visualization and continuously transferring refreshed reactants from the CSTR into the gel by diffusion. The opposite one of the gel disc was tightly pressed against a flat observation window. A detailed description and sketch of the disk-shaped OSFR is given in ref. 13 and 14. Patterns developed in the disc were monitored by a CCD video camera (Pixera, 49900-30, Cole-Parmer Co.), connected to a computer running Pixera motion software. For pH pattern formation, sodium polyacrylate (Aldrich, MW: 15
000 Daltons) with different concentrations was added to reservoir 2 and the gels. The immobilized carboxylate groups reversibly bind hydrogen ions resulting in an effective diffusion coefficient for hydrogen ions being much smaller than that of the reactants. A pH color indicator bromocresol green, which switches from blue to yellow in the 5.4–3.8 pH range, was added (0.2 g L−1) to reservoir 4. For iodine pattern formation, starch was added to the gel and reservoir 4. Starch acted as a color indicator and reduced the effective diffusion coefficient of the activator (iodide) as well. Bracketed terms [
]0 denote the concentration of the input species in question in the reactor after mixing prior to any reaction. [PA]0 and [starch]0 stand for the concentration of sodium polyacrylate and starch in gel and in the CSTR, respectively.
3. Batch experiment for pattern formation
The batch pattern formation was investigated in a thin layer of agarose gel at room temperature (25 ± 2 °C). Agarose stock solution was prepared by dissolving 0.1205 g of agarose in 30 mL of water at 100 °C and then the solution was cooled down to 40 °C. The thin gel was prepared by mixing the 0.4% agarose stock solution with other reactants according to desired concentrations and then the mixture solution was poured into a mold having a size of 9.5 cm × 8 cm × 1 mm. The gelation process typically was complete within several minutes by natural cooling. To visualize the pH or/and iodine wave formation, a few drops of a pH indicator (methyl red, which switched from red to yellow between 4.2–6.2 pH) or/and 0.025 g starch was/were added to the reaction mixture. Appropriate amounts of PA were also dissolved in the solution. The RD front was initiated electrochemically in the middle of the rectangular gel layer by applying a 65 V potential difference between a pair of Pt electrodes for about 5 s. Planar initiations were achieved along the edge of the rectangular gel by bringing a strip of filter paper, impregnated in sulfuric acid solution with the concentration of 0.17 mM, into contact with the reactant medium. The propagating front was monitored using a CCD video camera. During the pattern evolution, the gel was covered with a glass plate and sealed to a rectangular layer to prevent evaporation around the edges.
Results and discussions
1. CSTR dynamics
Fig. 1 shows the observed CSTR dynamics of the IST reaction in the [S2O32−]0–[H2SO4]0 plane. The phase diagram was established by gradually changing [H2SO4]0 for different fixed values of [S2O32−]0. The temporal bistability domain connects to an oscillatory domain through a typical cross-shaped phase diagram topology. For different fixed values of the [S2O32−]0, the CSTR contents at low input concentrations of [H2SO4]0 are in the so-called “flow state” (F), characterized by a high pH and low extent of the reaction; while at high input concentrations of [H2SO4]0, the CSTR contents switch to the so-called “thermodynamic state” (T), characterized by a low pH and high extent of the reaction. In this T state, one can observe the reddish brown color of iodine without having any iodine precipitation in the CSTR. Between the two monostable domains, the temporal bistability of these two steady states appears when [S2O32−]0 is lower than 17 mM. The bistable region was found in the range of 5 mM ≤ [H2SO4]0 ≤ 15 mM that gradually narrows as [S2O32−]0 increases. Above the concentration value of [S2O32−]0 = 17 mM, bistability is no longer observed and an oscillation region appears. The oscillation region becomes wider as thiosulfate concentration increases. Within the oscillation region we also observed complex oscillation in a narrow range of the input [S2O32−]0 and [H2SO4]0.
![CSTR phase diagram of the IST reaction in the [S0O32−]0–[H2SO4]0 plane. Symbols: ▲, flow state (F); ▼, thermodynamic state (T); ◆, bistability; ●, simple oscillations; ★, complex oscillations. [KIO3]0 = 0.075 M, [Na2SO3]0 = 0.2265 M, k0 = 3.64 × 10−3 s−1, T = 20.0 °C. Striped rectangles indicate the CSTR feed conditions where RD patterns developed in the disc OSFR.](/image/article/2012/CP/c1cp22281c/c1cp22281c-f1.gif) |
| Fig. 1 CSTR phase diagram of the IST reaction in the [S0O32−]0–[H2SO4]0 plane. Symbols: ▲, flow state (F); ▼, thermodynamic state (T); ◆, bistability; ●, simple oscillations; ★, complex oscillations. [KIO3]0 = 0.075 M, [Na2SO3]0 = 0.2265 M, k0 = 3.64 × 10−3 s−1, T = 20.0 °C. Striped rectangles indicate the CSTR feed conditions where RD patterns developed in the disc OSFR. | |
The CSTR feed conditions for pattern formation in the OSFR are marked with striped rectangles (Fig. 1). The contents of the CSTR were always maintained on the stationary state branch with low extent of reaction, i.e., the high pH flow branch, which ensured far-from-equilibrium conditions at the feed boundary of the gel.
2. pH pattern formation in OSFR
We first explored the pH patterns in the vicinity of bistability, marked as the large striped rectangle (Fig. 1). The transition from pH traveling waves to hexagonal spots was observed with the concentration of PA as the control parameter with the other parameters kept fixed. In the experiment, the blue color and yellow color represent a state of high pH and low pH, respectively. In the absence of PA, periodic traveling pulse waves were observed with large distances between wave fronts and trailing edges (Fig. 2a). The waves spontaneously and periodically appeared from the bottom part of the gel boundary and then propagated upward. Fig. 2a′ shows the space–time plot of the pulse waves with a period of 20 min. During the first few periods we observed pH waves accompanied by iodine waves. Adding 1.125 mM and 1.50 mM PA into the system, respectively, the striped patterns, with different wavelengths arising from front interactions, were found in this system. The pattern growth usually initiated at an edge of the disc gel but sometimes occurred in the middle. As shown in Fig. 2c, the striped patterns had larger wavelengths than the ones shown in Fig. 2b, which resulted from the greater PA concentration effectively reducing the diffusion coefficient of the hydrogen ions. Fig. 2c′ shows the phenomenon of front instability at the very first periods of the patterns evolved in Fig. 2c. The reddish brown color represents the iodine waves, which always accompanied the appearance of the pH waves during the first few periods of the patterns when the added PA concentration was in the range of 0–1.5 mM. When the PA concentration was increased to 2.25 mM, the transition from striped patterns to hexagonal spots was observed as a coexistence of the pattern shown in Fig. 2d. Further increase in the PA concentration (3.0 mM) results in the enhancement of hexagonal spots with larger wavelengths (Fig. 2e). In addition, as other conditions were fixed, an increase in the flow rate enlarged the pattern wavelength. Similar to the experimental observations of self-replicating spots in the FIS reaction,25 the spots underwent a continuous process of “birth” through replication. A few spots formed first at the gel boundary, then the spots propagated towards the center of the gel. During their propagation the initial spots gradually divided into two daughter spots, and the replication process repeatedly proceeded until the entire domain was filled with spots (movie-1 in ESI†). The spots interacted with each other to reach and maintain a critical distance and finally lead to the stationary spot patterns.
![pH patterns of the IST system in the disc OSFR. Fixed initial concentrations are as follows: [IO3−]0 = 0.075 M, [SO32−]0 = 0.2265 M, [S2O32−]0 = 0.010 M, [H2SO4]0 = 9.08 mM. The yellow (blue) corresponds to the state of low (high) pH state. (a) Snapshot of traveling pH waves; (a′) space–time plot of pH changes across a line section of the disc, following the direction of the yellow arrows on the snapshot; (b) and (c) stripe patterns; (c′) front instability; (d) and (e) hexagonal spots mixed with few stripes. [PA]0 = 0 mM (a); 1.125 mM (b); 1.50 mM (c, c′); 2.25 mM (d); 3.00 mM (e). k0 = 9.52 × 10−3 s−1. T = 24.0 °C.](/image/article/2012/CP/c1cp22281c/c1cp22281c-f2.gif) |
| Fig. 2 pH patterns of the IST system in the disc OSFR. Fixed initial concentrations are as follows: [IO3−]0 = 0.075 M, [SO32−]0 = 0.2265 M, [S2O32−]0 = 0.010 M, [H2SO4]0 = 9.08 mM. The yellow (blue) corresponds to the state of low (high) pH state. (a) Snapshot of traveling pH waves; (a′) space–time plot of pH changes across a line section of the disc, following the direction of the yellow arrows on the snapshot; (b) and (c) stripe patterns; (c′) front instability; (d) and (e) hexagonal spots mixed with few stripes. [PA]0 = 0 mM (a); 1.125 mM (b); 1.50 mM (c, c′); 2.25 mM (d); 3.00 mM (e). k0 = 9.52 × 10−3 s−1. T = 24.0 °C. | |
We also explored the pH pattern formation under the conditions close to the oscillatory domain in the CSTR, marked as the small rectangle (Fig. 1). As shown in Fig. 3, one can observe lattice patterns (Fig. 3a) with [PA]0 = 2.25 mM in the system, and the striped patterns (Fig. 3b) with [PA]0 = 3.00 mM. Different from the patterns observed at the vicinity of bistability in the CSTR, the patterns were only faintly visible.
![pH patterns in the disc OSFR. Fixed initial concentrations are the same as Fig. 1 except for [S2O32−]0 = 0.025 M, [H2SO4]0 = 11.9 mM, [PA]0a = 2.25 mM, [PA]0b = 3.00 mM. k0 = 6.98 × 10−3 s−1. T = 24.0 °C.](/image/article/2012/CP/c1cp22281c/c1cp22281c-f3.gif) |
| Fig. 3 pH patterns in the disc OSFR. Fixed initial concentrations are the same as Fig. 1 except for [S2O32−]0 = 0.025 M, [H2SO4]0 = 11.9 mM, [PA]0a = 2.25 mM, [PA]0b = 3.00 mM. k0 = 6.98 × 10−3 s−1. T = 24.0 °C. | |
3. Iodine pattern formation in OSFR
Here we report the reversible black-blue I3−–starch complex patterns, referred to as iodine waves or iodine patterns, which developed spontaneously from a uniform state at high pH. The transitions from the pulse waves to different patterns such as the analogous spot patterns, branched patterns, and labyrinthine patterns were studied with the content of starch as a control parameter. Fig. 4 shows examples of these patterns. There were no stationary periodic iodine patterns without starch in the system. The propagating pulse waves (Fig. 4a) annihilated and disappeared on collision as [starch]0 is 1.25 g L−1 in the system. We observed analogous white spot patterns in an iodine background (Fig. 4b), branched patterns (Fig. 4c) and labyrinthine patterns (Fig. 4d) as [starch]0 was increased to 2.5 g L−1, 3.75 g L−1 and 5.00 g L−1, respectively. At a starch concentration of 6.25 g L−1, more iodine became visible within the patterns in the form of a starch–triiodide complex as shown in Fig. 4f. Different types of patterns evolved with increasing the amount of starch, which leads to the conclusion that starch concentration acted as a bifurcation parameter for iodine pattern formation. In the above experiment, iodine patterns were observed even in a slight stoichiometric excess of sulfite over iodate. On the one hand, air oxygen unavoidably decreases the concentration of sulfite. On the other hand, in the OSFR consisting of a CSTR and a gel disc for pattern formation, iodine patterns form in the gel disc, however, blue colour with a starch indicator could not be observed in the CSTR at the same time, concluding that the dynamics in homogenous solution is different from that in reaction–diffusion medium. We infer that two autocatalytic processes including proton autocatalysis and iodide autocatalysis in the gel, which open to the CSTR solution, accelerate Dushman reaction31 for forming iodine waves. Moreover, the formed triiodide ion was bound by the starch to slow its consumption.
![Iodine patterns in different starch concentrations and flow rates. Fixed initial concentrations are as follows: [IO3−]0 = 0.075 M, [SO32−]0 = 0.2265 M, [S2O32−]0 = 0.010 M, [H2SO4]0 = 9.08 mM, [starch]0 = 1.25 g L−1 (a); 2.50 g L−1 (b); 3.75 g L−1 (c); 5.00 g L−1 (d, e); 6.25 g L−1 (f). k0 = 9.52 × 10−3 s−1 (a–d, f), k0 = 1.27 × 10−2 s−1 (e), the domain is 20 × 20 mm2. T = 23.5 °C. Black-blue (white) represents a state of low (high) pH.](/image/article/2012/CP/c1cp22281c/c1cp22281c-f4.gif) |
| Fig. 4 Iodine patterns in different starch concentrations and flow rates. Fixed initial concentrations are as follows: [IO3−]0 = 0.075 M, [SO32−]0 = 0.2265 M, [S2O32−]0 = 0.010 M, [H2SO4]0 = 9.08 mM, [starch]0 = 1.25 g L−1 (a); 2.50 g L−1 (b); 3.75 g L−1 (c); 5.00 g L−1 (d, e); 6.25 g L−1 (f). k0 = 9.52 × 10−3 s−1 (a–d, f), k0 = 1.27 × 10−2 s−1 (e), the domain is 20 × 20 mm2. T = 23.5 °C. Black-blue (white) represents a state of low (high) pH. | |
In our experiments, two possible routes may lead to the iodine pattern formation. The first one is the oscillating spots induced route to labyrinthine patterns. In this case, some spots emerge spontaneously first from a uniform high pH state in the gel or from the gel boundary and then grow and shrink or even disappear periodically, just as the oscillating spots, but evolution of these spots was not completely synchronous. During the evolution of the spots, interactions of the chemical fronts lead to their connection in adjacent directions resulting in the formation of stripes. The patterns have a wavelength determined by their interaction toward each other at a constant speed until they reach a critical distance and stop, as Fig. 4d illustrates. In the meantime, the endpoints of stripes develop locally and spread to fill space in the way of growing explosively and then disappearing periodically, which results in the labyrinthine pattern formation in the whole gel area (movie-2 in ESI†). The other route induced pattern formation can be approached by adjusting the flow rate under special conditions. At the beginning, iodine waves spontaneously appear and then grow continuously. The repulsive interaction between the waves is not strong enough to lead to the fusion of the domains. The iodine domain formed this way can separate slowly with appearance of gaps by increasing the flow rate and finally evolve into the stationary patterns in this way (movie-3 in ESI†).
The flow rate also has a remarkable effect on the iodine patterns. A good example of this phenomenon is illustrated in Fig. 4(d) and (e), under the conditions of a higher flow rate 4.0 mL min−1, the stationary labyrinthine patterns have longer wavelengths (Fig. 4e) than the patterns developed at 3.0 mL min−1 (Fig. 4d). In summary, iodine front propagation is inhibited under the condition of high flow rate with low extent of the reaction.
When the concentration of sulfuric acid is decreased and other initial concentrations of reagents, including PA, are fixed, there is a decrease in the rate constant (k = k0 [H+]2) of iodide autocatalysis and consequently a decrease in the restricted ratio of diffusion coefficients between activator and inhibitor that permits Turing structure formation.32 If the ratio of diffusion constants is larger than the restricted ratio, iodine patterns would change from stationary structures such as stripes and labyrinth to traveling waves. With the conditions the same as in Fig. 4d except [H2SO4]0 = 8 mM, stationary lines formed between colliding iodine waves, as illustrated in Fig. 5(a)–(f), which is different from the waves that collided to annihilate each other in Fig. 4a. This phenomenon may be explained as follows, the reaction that generates iodine also produces an inhibitor (OH−) from the iodate–iodide (Dushman) reaction. The inhibitor prevents iodine production and diffuses in front of (as well as behind) the wave, when two waves approach just before collision, the combined inhibitor concentration in the interwave region is high enough to suppress further production of iodine. In other regions, where the inhibitor comes only from a single wave, the autocatalytic production of iodine outweighs the effect of the inhibitor, which is present at about half the concentration in the collision region.
![Stationary lines formed between colliding iodine waves. Input chemical concentrations are the same as in Fig. 4 except for [H2SO4]0 = 8 mM. Time span of the images are, respectively, (a) 0 s, (b) 60 s, (c) 100 s, (d) 272 s, (e) 336 s, (f) 376 s. [starch]0 = 5.00 g L−1. k0 = 9.52 × 10−3 s−1, T = 25.5 °C.](/image/article/2012/CP/c1cp22281c/c1cp22281c-f5.gif) |
| Fig. 5 Stationary lines formed between colliding iodine waves. Input chemical concentrations are the same as in Fig. 4 except for [H2SO4]0 = 8 mM. Time span of the images are, respectively, (a) 0 s, (b) 60 s, (c) 100 s, (d) 272 s, (e) 336 s, (f) 376 s. [starch]0 = 5.00 g L−1. k0 = 9.52 × 10−3 s−1, T = 25.5 °C. | |
4. Coexistence and stability of pH and iodine waves in closed IST RD system
Coexistence of pH and iodine waves.
In the presence of indicators methyl red and starch in the RD medium, the IST reaction exhibits coexistence waves of pH and iodine fronts in a wide range of experimental conditions. Fig. 6a–c illustrate the evolution of the coexistence of waves. As a pH wave propagates it leaves behind acidic conditions that give rise to the birth of iodine waves as a result of autocatalytic production of iodide. This simply means that pH waves are necessarily followed by iodine waves under appropriate initial conditions of reactants since the diffusion coefficient of the hydrogen ion is higher than that of the iodide ion. In the experiment, red color represents pH waves while black-blue stands for iodine waves. pH and iodine fronts result from proton quadratic autocatalysis and iodide cubic autocatalysis, respectively. The individual travelling velocity is approximately constant but unequal, i.e., velocity difference is almost unchanged during propagation of waves in our experiment, so the distance between the pH front and iodine front increased linearly as a function of time as shown in Fig. 7a. As seen from Fig. 7b the distance between the waves was also affected by the initial concentrations of the reactants. When [S2O32−]0 is decreased from 7.6 mM to 7.2 mM the gap between the two forms of waves becomes smaller just as a result of the low production of OH− leading to enhancement of the iodine clock reaction at lower [S2O32−]0. A batch RD phase diagram of the IST reaction in the ([S2O32−]0–[H2SO4]0) plane exhibits three types of waves as shown in Fig. 8. At different fixed [S2O32−]0, the transition from pH pulses, pH front to coexistence waves can be observed as [H2SO4]0 is increased.
![Coexistence of a pH front and an iodine front. [IO3−]0 = 0.0104 M, [SO32−]0 = 0.015 M, [S2O32−]0 = 7.6 mM, [H+]0 = 7.2 mM. The sampling time of the snapshots is at 78 s (a), 228 s (b), 394 s (c), respectively. The domain is 5 × 5 cm.](/image/article/2012/CP/c1cp22281c/c1cp22281c-f6.gif) |
| Fig. 6 Coexistence of a pH front and an iodine front. [IO3−]0 = 0.0104 M, [SO32−]0 = 0.015 M, [S2O32−]0 = 7.6 mM, [H+]0 = 7.2 mM. The sampling time of the snapshots is at 78 s (a), 228 s (b), 394 s (c), respectively. The domain is 5 × 5 cm. | |
![The distance between pH wavefront and iodine wavefront vs. time in the IST RD system. [IO3−]0 = 0.0104 M, [SO32−]0 = 0.015 M, [H+]0 = 7.2 mM, [S2O32−]0 = 7.6 mM (a), 7.2 mM (b). Methyl red and starch were used as indicators for pH fronts and iodine fronts, respectively.](/image/article/2012/CP/c1cp22281c/c1cp22281c-f7.gif) |
| Fig. 7 The distance between pH wavefront and iodine wavefront vs. time in the IST RD system. [IO3−]0 = 0.0104 M, [SO32−]0 = 0.015 M, [H+]0 = 7.2 mM, [S2O32−]0 = 7.6 mM (a), 7.2 mM (b). Methyl red and starch were used as indicators for pH fronts and iodine fronts, respectively. | |
![Phase diagram of the IST reaction–diffusion system in a batch in the [S2O32−]–[H+] plane. [KIO3]0 = 0.0104 M, [Na2SO3]0 = 0.015 M. () pH pulses, () pH fronts, () coexisted fronts.](/image/article/2012/CP/c1cp22281c/c1cp22281c-f8.gif) |
| Fig. 8 Phase diagram of the IST reaction–diffusion system in a batch in the [S2O32−]–[H+] plane. [KIO3]0 = 0.0104 M, [Na2SO3]0 = 0.015 M. ( ) pH pulses, ( ) pH fronts, ( ) coexisted fronts. | |
Instability of pH and iodine waves.
We first chose specific experimental conditions of [H2SO4]0 = 1.7 mM, [Na2SO3]0 = 6.5 mM, [Na2S2O3]0 = 3.5 mM, [KIO3]0 = 2.5 mM for pH front to study the front instability. Upon initiation, a planar pH front propagates toward the reactant medium. In gels without PA the planar front is stable, retaining its geometry. However, the planar front loses stability in gels containing sufficient amount of PA ([PA]0 = 0.9 mM), yielding a cellular structure as shown in Fig. 9a and b where the cells having slightly curved leading segments are joined in sharp cusps. At the same time, a diffusive white area occurred behind the cellular structure along the pH front, resulting from proton negative feedback to high pH. We observed this phenomenon in a very narrow range of PA concentrations, since at higher PA concentration the time necessary to form cellular structure becomes longer than the induction time of the reaction.
![The cellular fronts in IST RD system. Red and white (bright color) stand for low pH and high pH, respectively. (a) Front at t = 20 min after initiation; (b) front at t = 100 min exhibits definite cell formation. The reagent concentrations are [H2SO4]0 = 1.7 mM, [Na2SO3]0 = 6.5 mM, [Na2S2O3]0 = 3.5 mM, [KIO3]0 = 2.5 mM, [PA]0 = 0.9 mM. The domain is 2.5 cm × 6 cm.](/image/article/2012/CP/c1cp22281c/c1cp22281c-f9.gif) |
| Fig. 9 The cellular fronts in IST RD system. Red and white (bright color) stand for low pH and high pH, respectively. (a) Front at t = 20 min after initiation; (b) front at t = 100 min exhibits definite cell formation. The reagent concentrations are [H2SO4]0 = 1.7 mM, [Na2SO3]0 = 6.5 mM, [Na2S2O3]0 = 3.5 mM, [KIO3]0 = 2.5 mM, [PA]0 = 0.9 mM. The domain is 2.5 cm × 6 cm. | |
As we set [KIO3]0 = 3.75 mM with keeping other initial concentrations unchanged, the iodine front appeared to propagate behind the pH front. When PA concentration is increased to 0.7 mM, at this point, the coexistence pH and iodine fronts remain basically stable with the planar initiation as shown in Fig. 10a. However, a white stripe, also resulting from proton negative feedback, appeared between pH and iodine fronts as shown in Fig. 10b. The white stripe always existed (Fig. 10c). Even a new iodine stripe appears ahead of the white striped zone, as seen in Fig. 10d and e. pH is high, and no I3−–starch complex exists in the white striped zone due to the lack of iodine formation from the Dushman reaction. With all above initial concentrations, Fig. 11 further shows the evolution of the pattern in the absence of a pH indicator by perturbing the homogeneous gel medium with three parallel planar initiations, leading to transient iodine Turing structures.
![Stripe formation in IST RD system. Red, white (bright color) and dark stand for low pH, high pH and existence of iodine, respectively. The conditions are the same as in Fig. 9 except [KIO3]0 = 3.75 mM, [PA]0 = 0.7 mM and [starch] = 0.83 g L−1. The domain is 5.5 cm × 5 cm. The sampling time of the snapshots is at 10 min (a), 47 min (b), 56 min (c), 63 min (d), 92 min (e).](/image/article/2012/CP/c1cp22281c/c1cp22281c-f10.gif) |
| Fig. 10 Stripe formation in IST RD system. Red, white (bright color) and dark stand for low pH, high pH and existence of iodine, respectively. The conditions are the same as in Fig. 9 except [KIO3]0 = 3.75 mM, [PA]0 = 0.7 mM and [starch] = 0.83 g L−1. The domain is 5.5 cm × 5 cm. The sampling time of the snapshots is at 10 min (a), 47 min (b), 56 min (c), 63 min (d), 92 min (e). | |
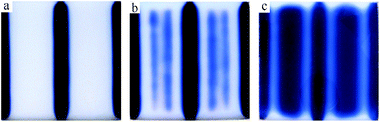 |
| Fig. 11 Turing structure in IST RD system. The conditions are the same as in Fig. 10 except for the absence of a pH indicator. The sampling time of the snapshots is at 37 min (a), 58 min (b), 116 min (c). The domain is 9.5 × 8.0 cm. | |
5. Mechanism analysis of the IST system for pattern formation
The IST system is a mixed Landolt type reaction. The well-known iodate–sulfite reaction is autocatalytic both for protons and iodide ions. Except the Landolt type reaction for iodide-ion autocatalysis, the iodate–thiosulfate reaction consumes proton, resulting in the proton negative feedback. In previous studies, because the iodide autocatalytic process only becomes important at the end of the oxidation of sulfite when the pH drops below 5,33 the role of the iodate–iodide (Dushman) reaction31 was neglected. But for pattern formation of the IST system in RD medium under special conditions, for example, for the iodine patterns in the OSFR and coexistence waves observed above, the iodide ion autocatalytic process and the reactions relating to iodine cannot be neglected. The following mechanism was proposed for discussing pattern formation for the IST RD system. | IO3− + 3HSO3− → I− + 3SO42− + 3H+ | (R3) |
| 5I− + IO3− + 6H+ → 3I2 + 3H2O | (R4) |
| HSO3− + I2 + H2O → SO42− + 2I− + 3H+ | (R5) |
| I2 + 2S2O32− → 2I− + S4O62− | (R6) |
| IO3− + 6S2O32− + 6H+ → I− + 3S4O62− + 3H2O | (R7) |
| 2IO3− + 3S2O32− + 3H2O → 2I− + 6HSO3− | (R8) |
The mechanism of the composed IST system contains multiple positive and negative feedback processes. The proton autocatalytic oxidation of hydrogen sulfite by iodate is a positive feedback (R3), while the proton consuming oxidation of thiosulfate by iodate into tetrathionate is a negative feedback (R7). Parallel oxidation of thiosulfate by iodate reproducing hydrogen sulfite (R8) contributes to the proton positive feedback coupling with reaction (R3). For the existence of parallel oxidation of thiosulfate ions by iodate ions into tetrathionate and sulfite ions, Rábai and Beck28 established different rate equations and their corresponding rate coefficients, and Horváth34 further improved them without the inhibition item of sulfite by the simultaneous curve fitting procedure to the preliminary experiments of the system. The autocatalytic reactions for iodide (R4 + 3R5 and R4 + 3R6), and the reactions for iodine production (R4) and consumption (R6) mainly determine the iodine pattern formation and evolution in the disc gel. The fast reversible equilibrium reaction (R1) has a buffering effect to keep the pH around 7 at the beginning of the reaction. The R2 reaction provides I3− necessary for the formation of a starch–triiodide complex, which effectively reduces the diffusion coefficient of iodide ions. The autocatalytic reaction with respect to iodide ions always occurs at the later phase of the reaction when the pH is low enough to ignite the Dushman reaction (R4) followed by instantaneous processes of (R5) and (R6).
Conclusions
As we expected, two types of pH patterns and iodine patterns were observed in the IST RD system. A systematic study of pH pattern formation ranging from traveling waves to striped patterns to hexagonal spot patterns is presented by increasing the PA content in the system, and the iodine pattern formation transition from traveling waves to branched patterns to labyrinthine patterns was observed by increasing the starch content. In accordance with De Kepper's experimental design method for pattern formation, the proton and iodide-ion autocatalytic reactions coupled with inhibitory reactions naturally produce stationary patterns by control of the effective diffusivity with PA and starch, respectively, in the IST RD system. Coexistence of pH and iodine waves was observed at a wide range of initial concentrations in a batch RD system. Cellular fronts and Turing structures arise as a result of the different diffusion constants of the interacting species caused by selective binding of the autocatalyst. The iodine waves always appeared behind the pH waves, which leads to the conclusion that the iodine clock reaction can be induced by a proton positive feedback process in the RD medium. Our study may inspire further interest for searching other types of patterns in the oscillatory region (including complex oscillations) and to study the relationship between the pH and iodine patterns.
Acknowledgements
This work was supported in part by Grants (No. 21073232 and 50921002) from the National Natural Science Foundation of China and the Fundamental Research Fund (No. 2010LKHX02) from the Chinese Central University. H. L. is grateful for the financial support of the visiting program to Louisiana State University from the Chinese Scholarship Council. J. P. acknowledges Louisiana State University for financial support. A. K. H. is grateful for the János Bolyai Research Scholarship of the Hungarian Academy of Sciences. We also thank Prof. Irving R. Epstein (Brandies University) for giving his idea to explain experimental phenomena.
References
-
J. D. Murray, Mathematical Biology, Springer, New York, 2004 Search PubMed.
-
H. Meinhardt, Models of Biological Pattern Formation, Academic Press, London, England, 1982 Search PubMed.
-
Chemical Patterns and Waves, ed. R. Kapral and K. Showalter, Kluwer Academic Publisher, Amsterdam, The Netherlands, 1995 Search PubMed.
- V. Castets, E. Dulos, J. Boissonade and P. De Kepper, Phys. Rev. Lett., 1990, 64, 2953 CrossRef CAS.
- A. T. Winfree, Science, 1972, 175, 634 CrossRef CAS.
- H. Guo, L. Li, Q. Ouyang, J. Liu and Zh. She, J. Chem. Phys., 2003, 118, 5038 CrossRef CAS.
- A. F. Taylor, Prog. React. Kinet. Mech., 2002, 27, 247 CAS.
- Q. Ouyang and H. L. Swinney, Chaos, 1991, 1, 411 CrossRef.
-
V. K. Vanag and I. R. Epstein, In Patterns of Nanodroplets: The Belousov–Zhabotinsky-Aerosol OT-Microemulsion System, Springer, Berlin, 2008, Ch 5 Search PubMed.
- K. J. Lee, W. D. McCormick, Q. Ouyang and H. L. Swinney, Science, 1993, 261, 192 CrossRef CAS.
- K. J. Lee, W. D. McCormick, H. E. Pearson and H. L. Swinney, Nature, 1994, 369, 215 CrossRef.
- A. De Wit, Adv. Chem. Phys., 1999, 109, 435 CAS.
- B. Rudovics, E. Barillot, P. W. Davies, E. Dulos, J. Boissonade and P. De Kepper, J. Phys. Chem. A, 1999, 103, 1790 CrossRef CAS.
- P. Blanchedeau, J. Boissonade and P. De Kepper, Phys. D, 2000, 147, 283 CrossRef CAS.
- J. Boissonade, E. Dulos, F. Gauffre, M. N. Kuperman and P. De Kepper, Faraday Discuss., 2001, 120, 353 Search PubMed.
- M. Fuentes, M. N. Kuperman, J. Boissonade, E. Duols, F. Gauffre and P. De Kepper, Phys. Rev. E: Stat., Nonlinear, Soft Matter Phys., 2002, 66, 056205 CrossRef CAS.
- I. Szalai, F. Gauffre, V. Labrot, J. Boissonade and P. De Kepper, J. Phys. Chem. A, 2005, 109, 7843 CrossRef CAS.
- I. Szalai and P. De Kepper, Phys. Chem. Chem. Phys., 2006, 8, 1105 RSC.
- Z. Virányi, I. Szalai, J. Boissonade and P. De Kepper, J. Phys. Chem. A, 2007, 111, 8090 CrossRef CAS.
- I. Szalai and P. De Kepper, Chaos, 2008, 18, 026105 CrossRef.
- I. Szalai and P. De Kepper, J. Phys. Chem. A, 2008, 112, 783 CrossRef CAS.
- J. Horváth, I. Szalai and P. De Kepper, Science, 2009, 324, 772 CrossRef CAS.
- J. Horváth, I. Szalai and P. De Kepper, Phys. D, 2010, 239, 776 CrossRef CAS.
- J. Boissonade, P. De Kepper, F. Gauffre and I. Szalai, Chaos, 2006, 16, 037110 CrossRef CAS.
- G. Li, Q. Ouyang and H. L. Swinney, J. Chem. Phys., 1996, 105, 10830 CrossRef CAS.
- K. J. Lee and H. L. Swinney, Phys. Rev. E: Stat. Phys., Plasmas, Fluids, Relat. Interdiscip. Top., 1995, 51, 1899 CrossRef CAS.
- G. Rábai and B. M. T. Beck, J. Phys. Chem., 1988, 92, 2804 CrossRef CAS.
- G. Rábai and B. M. T. Beck, J. Phys. Chem., 1988, 92, 4831 CrossRef CAS.
- H. M. Liu, J. X. Xie, L. Yuan and Q. Y. Gao, J. Phys. Chem. A, 2009, 113, 11295 CrossRef CAS.
- Q. Y. Gao and R. Y. Xie, ChemPhysChem, 2008, 9, 1153 CrossRef CAS.
- S. Dushman, J. Phys. Chem., 1904, 8, 453 CrossRef.
-
I. R. Epstein and J. A. Pojman, An Introduction to Nonlinear Chemical Dynamics, Oscillations, Waves, Patterns, and Chaos, Oxford University Press, New York, America, 1998, p. 299–323 Search PubMed.
- G. Rábai, A. Kaminaga and I. Hanazaki, J. Phys. Chem., 1995, 99, 9795 CrossRef CAS.
- A. K. Horváth, J. Phys. Chem. A, 2008, 112, 3935 CrossRef CAS.
Footnote |
† Electronic supplementary information (ESI) available: Movies for self-replication of pH spots (movie-1), labyrinthine patterns induced by oscillations of iodine spots (movie-2) and stripe pattern induced by domain fusion of iodine when adjusting flow rate (movie-3). See DOI: 10.1039/c1cp22281c |
|
This journal is © the Owner Societies 2012 |
Click here to see how this site uses Cookies. View our privacy policy here.