DOI:
10.1039/C1CE05877K
(Paper)
CrystEngComm, 2012,
14, 286-299
A series of coordination polymers based on 5,5′-(ethane-1,2-diyl)-bis(oxy)diisophthalic acid and structurally related N-donor ligands: syntheses, structures and properties†
Received
13th July 2011
, Accepted 9th September 2011
First published on 18th October 2011
Abstract
Ten new coordination polymers, namely, [Ni2(L)(H2O)3]·5H2O (1), [Ni2(L)(pbib)2(H2O)]·H2O (2), [Co2(L)(pbib)1.5]·2.5H2O (3), [Co2(L)(bbtz)1.5(H2O)5] (4), [Ni(L)0.5(biim-3)0.5(H2O)(CH3CH2OH)0.5]·2H2O (5), [Zn3(L)(biim-3)(OH)2] (6), [Ni(L)0.5(btp)(H2O)2]·H2O (7), [Zn2(L)(btp)2]·3H2O (8), [Co(L)0.5(btb)0.5(H2O)2]·1.5H2O (9), and [Zn(L)0.5(btb)]·0.5H2O (10), where pbib = 1,4-bis(imidazol-1-ylmethyl)benzene, bbtz = 1,4-bis(1H-1,2,4-triazol-1-ylmethyl)benzene, biim-3 = 1,3-bis(imidazol-1-yl)propane, btp = 1,3-bis(1,2,4-triazol-1-yl)propane, btb = 1,4-bis(1,2,4-triazol-1-yl)butane, and H4L = 5,5′-(ethane-1,2-diyl)-bis(oxy)diisophthalic acid, have been synthesized under hydrothermal conditions. Their structures have been determined by single-crystal X-ray diffraction analyses and further characterized by infrared spectra (IR), elemental analyses, powder X-ray diffraction (PXRD) and thermogravimetric (TG) analyses. Compound 1 features a 2-fold interpenetrating diamond-like framework. Compound 2 is a 3D 4-connected net with the (86)(73·82·9)(82·92·102)(82·94) topology. Compound 3 is a 3D 2-fold interpenetrating framework with the (5·62)(4·54·6)(42·58·85) topology. Compound 4 shows a rare 3D (4,7)-connected net with the (3·4·5·63)(32·42·5·613·73) topology. Compounds 5 and 6 exhibit 3D 2-fold interpenetrating frameworks with the (52·62·82)(42·82·92)(4·54·6) and (54·62) (58·66·7) topologies, respectively. Compounds 7 and 8 are 3D 3-fold interpenetrating frameworks with the 64·82 and (64·82)2(66)2 topologies, respectively. Compound 9 has a (3,4)-connected 2D layer structure with the (4·62)(42·62·82) topology. Compound 10 is a 3D 3-fold interpenetrating framework with the (62·8)(62·82·102) topology. The effects of the L anions, the N-donor ligands, and the metal ions on the structures of the coordination polymers have been discussed. The luminescent properties of 6, 8 and 10 and the optical band gaps of 1–10 have also been investigated in details.
Introduction
In the past decades, coordination polymers have received a great deal of attention due to their attractive topologies and potential applications in luminescent materials, catalyst, magnetism, nonlinear optics, and gas absorption.1 So far, although much effort has been carried out on the rational design and syntheses of coordination polymers, it is still a great challenge to predict the structures and properties of the target coordination polymers.2 Usually, the structures of the coordination polymers are dependent upon several factors, such as the pH values of the solution, reaction temperatures, geometric requirements of the metal atoms, and the organic ligands.3 Among these factors, the organic ligands play a key role in the design and construction of various coordination polymers. In this regard, the versatile multicarboxylic acids were widely used to construct coordination polymers because of their reliable and rich coordination modes.4Tetracarboxylic acids, bearing flexible spacers between the two oxyisophthalic acid fragments, are of special interest because of their flexibility and conformational freedom, which may provide more possibilities for the construction of frameworks with new topologies and properties.5 Up to now, several tetracarboxylic acids of this kind, such as 5,5′-(propane-1,3-diyl)-bis(oxy)diisophthalic acid, 5,5′-(butane-1,4-diyl)-bis(oxy)diisophthalic acid, 5,5′-(phenyl-1,4-diylbis(methylene))bis(oxy)diisophthalic acids, and 5,5′-(biphenyl-4,4′-diylbis(methylene))bis(oxy)diisophthalic acids, have been used in the syntheses of coordination polymers with interesting topologies and properties.5 Among these tetracarboxylic acids, the 5,5′-(ethane-1,2-diyl)-bis(oxy)diisophthalic acid (H4L) (Scheme 1), possessing a (–CH2–)2 spacer between the two oxyisophthalic acid moieties, is particularly attractive.5a,5h,6 The flexible nature of the (–CH2–)2 linker will cause the carboxylate groups to coordinate to central metals in different directions, providing various possibilities for the formation of the frameworks with unique structures and properties.
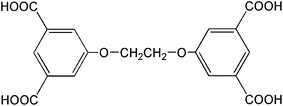 |
| Scheme 1 Structure of H4L. | |
On the basis of the aforementioned points, in this work, we selected the flexible H4L as the organic anion, and investigated the effects of the N-donor ligands on the structures of the complexes (Scheme 2). Ten new complexes, namely [Ni2(L)(H2O)3]·5H2O (1), [Ni2(L)(pbib)2(H2O)]·H2O (2), [Co2(L)(pbib)1.5]·2.5H2O (3), [Co2(L)(bbtz)1.5(H2O)5] (4), [Ni(L)0.5(biim-3)0.5(H2O)(CH3CH2OH)0.5]·2H2O (5), [Zn3(L)(biim-3)(OH)2] (6), [Ni(L)0.5(btp)(H2O)2]·H2O (7), [Zn2(L)(btp)2]·3H2O (8), [Co(L)0.5(btb)0.5(H2O)2]·1.5H2O (9), and [Zn(L)0.5(btb)]·0.5H2O (10), where pbib = 1,4-bis(imidazol-1-ylmethyl)benzene, bbtz = 1,4-bis(1H-1,2,4-triazol-1-ylmethyl)benzene, biim-3 = 1,3-bis(imidazol-1-yl)propane, btp = 1,3-bis(1,2,4-triazol-1-yl)propane, btb = 1,4-bis(1,2,4-triazol-1-yl)butane, and H4L = 5,5′-(ethane-1,2-diyl)-bis(oxy)diisophthalic acid, have been synthesized under hydrothermal conditions. All the compounds are characterized by X-ray crystallography, elemental analyses, infrared spectra (IR), powder X-ray diffraction (PXRD) and thermogravimetric analyses (TG). The structures and topological analyses of these compounds, as well as the effects of L anions, N-donor ligands, and metal ions on the structures of the coordination polymers are discussed in detail. Furthermore, the photoluminescent properties of compounds 6, 8 and 10 and the optical band gaps of compounds 1–10 have also been investigated.
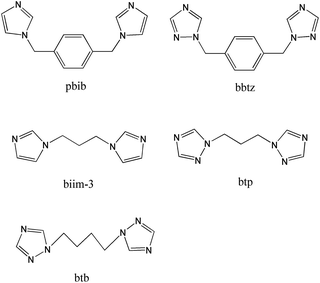 |
| Scheme 2 Structures of the N-donor ligands used in this work. | |
Experimental
Materials and methods
H4L and the N-donor ligands were prepared by the procedures reported.6,7 Other reagents were obtained commercially and used as received. The C, H and N elemental analyses were conducted on a Perkin-Elmer 240C elemental analyzer. The FT-IR spectra were recorded from KBr pellets in the range of 4000–400 cm−1 on a Mattson Alpha-Centauri spectrometer. Thermogravimetric analyses (TGA) were performed on a Perkin-Elmer TG-7 analyzer heated from room temperature to 800 °C under nitrogen gas. The photoluminescent properties of the compounds were measured on a Perkin-Elmer FLS-920 spectrometer. PXRD patterns of the samples were collected on a Rigaku Dmax 2000 X-ray diffractometer with graphite monochromatized Cu-Kα radiation (λ = 0.154 nm) and 2θ ranging from 5 to 50°. The experimental PXRD patterns are in good agreement with the corresponding simulated ones (Fig. S1 and S2, ESI†). Diffuse reflectivity spectra were collected on a finely ground sample with a Cary 500 spectrophotometer equipped with a 110 mm diameter integrating sphere, which were measured from 200 to 1000 nm using barium sulfate as a standard with 100% reflectance.
Synthesis of [Ni2(L)(H2O)3]·5H2O (1).
A mixture of H4L (0.039 g, 0.1 mmol), Ni(OAc)2·4H2O (0.050 g, 0.20 mmol), NaOH (0.016 g, 0.4 mmol) and water (8 mL) was placed in a Teflon reactor (15 mL) and heated at 130 °C for 3 days. After the mixture had been cooled to room temperature at a rate of 10 °C h−1, green crystals of 1 were obtained in 46% yield (based on Ni(OAc)2·4H2O). Anal. Calcd for C18H26Ni2O18 (Mr = 647.81): C, 33.37; H, 4.05. Found: C, 33.33; H, 4.14. IR (KBr, cm−1): 3442(s), 1740(w), 1676(m), 1628(s), 1533(s), 1463(s), 1393(s), 1263(w), 1138(w), 1067(w), 1011(w), 898(w), 784(m), 748(w), 668(w), 580(w), 453(w), 423(w).
Synthesis of [Ni2(L)(pbib)2(H2O)]·H2O (2).
The preparation of 2 was similar to that of 1 except that pbib (0.047 g, 0.2 mmol) was introduced. Green crystals of 2 were collected in 55% yield (based on Ni(OAc)2·4H2O). Anal. Calcd for C46H42Ni2N8O12 (Mr = 1016.30): C, 54.36; H, 4.17; N, 11.03. Found: C, 54.44; H, 4.28; N, 11.08. IR (KBr, cm−1): 3443(w), 3139(w), 1741(w), 1693(w), 1645(w), 1615(w), 1547(s), 1456(s), 1376(s), 1262(m), 1232(m), 1087(m), 1060(m), 1026(m), 922(m), 803(s), 783(s), 757(s), 721(s), 658(s), 422(m).
Synthesis of [Co2(L)(pbib)1.5]·2.5H2O (3).
The preparation of 3 was similar to that of 2 except that Co(OAc)2·4H2O (0.050 g, 0.20 mmol) was used instead of Ni(OAc)2·4H2O. Purple crystals of 3 were obtained in 39% yield (based on Co(OAc)2·4H2O). Anal. Calcd for C39H36Co2N6O12.5 (Mr = 906.60): C, 51.66; H, 4.01; N, 9.27. Found: C, 51.59; H, 4.09; N, 9.22. IR (KBr, cm−1): 3446(m), 3128(w), 1675(m), 1625(s) 1564(s), 1454(s), 1373(s), 1263(m), 1093(m), 1013(w), 947(w), 887(w), 832(w), 778(m), 721(m), 657(w), 462(w).
Synthesis of [Co2(L)(bbtz)1.5(H2O)5] (4).
The preparation of 4 was similar to that of 3 except that bbtz (0.048 g, 0.2 mmol) was used instead of pbib. Purple crystals of 4 were obtained in 25% yield (based on Co(OAc)2·4H2O). Anal. Calcd for C36H38Co2N9O15 (Mr = 954.61): C, 45.29; H, 4.02; N, 13.21. Found: C, 45.22; H, 4.13; N, 13.15. IR (KBr, cm−1): 3440(s), 1740(w), 1634(s), 1564(s), 1452(m), 1375(s), 1268(m), 1130(m), 1074(w), 1012(w), 890(w), 784(m), 721(w), 675(w).
Synthesis of [Ni(L)0.5(biim-3)0.5(H2O)(CH3CH2OH)0.5]·2H2O (5).
A mixture of H4L (0.039 g, 0.1 mmol), Ni(OAc)2·4H2O (0.050 g, 0.20 mmol), biim-3 (0.035 g, 0.2 mmol), NaOH (0.016 g, 0.4 mmol) and 8 mL of water-ethanol [3
:
1 (v/v)] solution was placed in a Teflon reactor (15 mL) and heated at 130 °C for 3 days. After the mixture had been cooled to room temperature at a rate of 10 °C h−1, green crystals of 5 were obtained in 43% yield (based on Ni(OAc)2·4H2O). Anal. Calcd for C29H40Ni2N4O17 (Mr = 834.07): C, 41.76; H, 4.84; N, 6.72. Found: C, 41.85; H, 4.79; N, 6.68. IR (KBr, cm−1): 3442(s), 3129(m), 2944(m), 1625(s), 1566(s), 1456(s), 1373(s), 1265(m), 1104(w), 1069(w), 1005(w), 949(w), 894(w), 832(w), 782(m), 722(m), 660(w), 425(w).
Synthesis of [Zn3(L)(biim-3)(OH)2] (6).
A mixture of H4L (0.039 g, 0.1 mmol), Zn(OAc)2·2H2O (0.044 g, 0.20 mmol), biim-3 (0.035 g, 0.2 mmol), NaOH (0.016 g, 0.4 mmol) and water (8mL) was placed in a Teflon reactor (15 mL) and heated at 160 °C for 3 days. After the mixture had been cooled to room temperature at a rate of 10 °C h−1, colorless crystals of 6 were obtained in 52% yield (based on Zn(OAc)2·2H2O). Anal. Calcd for C27H24N4O12Zn3 (Mr = 792.61): C, 40.91; H, 3.06; N, 7.07. Found: C, 40.85; H, 3.13; N, 7.19. IR (KBr, cm−1): 3450(m), 3116(w), 2945(w), 1625(s), 1551(s), 1454(s), 1361(s), 1261(m), 1102(m), 1066(w), 1004(w), 955(w), 888(w), 845(w), 778(m), 725(m), 652(w), 513(w), 418(w).
Synthesis of [Ni(L)0.5(btp)(H2O)2]·H2O (7).
The preparation of 7 was similar to that of 2 except that btp (0.035 g, 0.2 mmol) was used instead of pbib. Green crystals of 7 were obtained in 39% yield (based on Ni(OAc)2·4H2O). Anal. Calcd for C16H21NiN6O8 (Mr = 484.10): C, 39.69; H, 4.38; N, 17.36. Found: C, 39.58; H, 4.32; N, 17.28. IR (KBr, cm−1): 3439(s), 3129(m), 1550(s), 1452(m), 1369(s), 1284(m), 1262(m), 1205(w), 1131(m), 1059(w), 990(w), 891(w), 779(m), 719(m), 677(w), 648(w), 422(w).
Synthesis of [Zn2(L)(btp)2]·3H2O (8).
The preparation of 8 was similar to that of 7 except that Zn(OAc)2·2H2O (0.044 g, 0.2 mmol) was used instead of Ni(OAc)2·4H2O. Colorless crystals of 8 were obtained in 41% yield (based on Zn(OAc)2·2H2O). Anal. Calcd for C32H36Zn2N12O13 (Mr = 927.47): C, 41.44; H, 3.92; N, 18.13. Found: C, 41.39; H, 4.05; N, 18.05. IR (KBr, cm−1): 3442(m), 1627(s), 1577(s), 1459(s), 1387(s), 1271(m), 1133(w), 1057(w), 997(w), 923(w), 891(w), 777(m), 729(w), 672(w), 549(w), 423(w).
Synthesis of [Co(L)0.5(btb)0.5(H2O)2]·1.5H2O (9).
The preparation of 9 was similar to that of 3 except that btb (0.038 g, 0.2 mmol) was used instead of pbib. Purple crystals of 9 were obtained in 35% yield (based on Co(OAc)2·4H2O). Anal. Calcd for C13H18CoN3O8.5 (Mr = 411.23): C, 37.97; H, 4.42; N, 10.22. Found: C, 37.86; H, 4.49; N, 10.13. IR (KBr, cm−1): 3441(s), 1835(w), 1740(w), 1677(w), 1627(s), 1550(s), 1455(s), 1381(s), 1264(w), 1134(w), 1072(w), 1002(w), 893(w), 778(w), 724(w), 672(w), 573(w), 449(w).
Synthesis of [Zn(L)0.5(btb)]·0.5H2O (10).
The preparation of 10 was similar to that of 9 except that Zn(OAc)2·2H2O (0.044 g, 0.2 mmol) was used instead of Co(OAc)2·4H2O. Colorless crystals of 10 were obtained in 64% yield (based on Zn(OAc)2·2H2O). Anal. Calcd for C17H18ZnN6O5.5 (Mr = 459.74): C, 44.41; H, 3.95; N, 18.28. Found: C, 44.33; H, 3.89; N, 18.21. IR (KBr, cm−1): 3444(m), 3101(w), 2945(w), 2881(w), 1625(s), 1578(s), 1452(s), 1343(s), 1287(m), 1261(m), 1215(w), 1132(s), 1067(s), 996(s), 893(w), 782(s), 731(m), 669(w), 644(w), 544(w), 475(w), 440(w).
Single-crystal X-ray diffraction data for 1–10 were recorded on an Oxford Diffraction Gemini R Ultra diffractometer with graphite-monochromated Mo-Kα radiation (λ = 0.71073 Å) at 293 K. Absorption corrections were applied using multi-scan technique. All the structures were solved by Direct Method of SHELXS-978 and refined by full-matrix least-squares techniques using the SHELXL-97 program.9Non-hydrogen atoms of the complexes were refined anisotropically. All hydrogen atoms on carbon atoms were generated geometrically and refined using a riding model with d(C–H) = 0.93 Å, Uiso = 1.2Ueq(C) for aromatic and d(C–H) = 0.97 Å, Uiso = 1.2Ueq(C) for CH2 atoms. Aqua hydrogen atoms of compounds 1–5 and 7–10, hydrogen atoms of the ethanol molecule for 5 and hydroxyl hydrogen atoms for 6 were not included in the model. Some C and N atoms of 5 (N1, C10, C11, C12, C13, C15 and C16) and 9 (N2 and C10) are disordered, and split over two sites, with a total occupancy of 1. The hydrogen atoms of the disordered C atoms were not included in the model. The detailed crystallographic data and structure refinement parameters for these compounds are summarized in Table 1. Selected bond distances and angles for them are listed in Tables S1–S10 (see ESI†).
|
1
|
2
|
3
|
4
|
5
|
6
|
7
|
8
|
9
|
10
|
R
1 = Σ‖Fo| − |Fc‖/Σ|Fo|.
wR2 = |Σw(|Fo|2 − |Fc|2)|/Σ|w(Fo2)2|1/2.
|
Formula |
C18H26Ni2O18 |
C46H42N8Ni2O12 |
C39H36Co2N6O12.50 |
C36H38Co2N9O15 |
C29H40N4Ni2O17 |
C27H24N4O12Zn3 |
C16H21N6NiO8 |
C32H36N12O13 Zn2 |
C13H18CoN3O8.50 |
C17H18N7O5.50Zn |
fw
|
647.81 |
1016.30 |
906.60 |
954.61 |
834.07 |
792.61 |
484.10 |
927.47 |
411.23 |
459.74 |
Crystal system |
Monoclinic |
Monoclinic |
Triclinic |
Orthorhombic |
Monoclinic |
Triclinic |
Monoclinic |
Monoclinic |
Triclinic |
Monoclinic |
Space group |
P21/n |
P21/c |
P
|
pbcn
|
C2/m |
P
|
P21/c |
P21/n |
P
|
C2/c |
a/Å |
13.3464(6) |
7.429 |
12.6861(8) |
17.930(5) |
13.2776(4) |
9.536(5) |
6.6602(3) |
13.088(5) |
9.731(5) |
21.092(5) |
b/Å |
13.8945(4) |
24.143 |
13.3872(10) |
20.008(5) |
22.1202(6) |
10.243(5) |
16.2245(7) |
16.376(5) |
10.120(5) |
15.132(5) |
c/Å |
14.5650(6) |
24.275 |
13.4602(8) |
22.662(5) |
14.2690(4) |
15.501(5) |
18.3730(9) |
18.092(5) |
10.597(5) |
12.318(5) |
α (°) |
90 |
90 |
91.588(5) |
90 |
90 |
89.398(5) |
90 |
90.000(5) |
67.258(5) |
90.000(5) |
β (°) |
114.553(5) |
96.58 |
100.888(5) |
90 |
112.540(4) |
76.990(5) |
90.984(4) |
101.446(5) |
62.830(5) |
100.208(5) |
γ (°) |
90 |
90 |
115.561(7) |
90 |
90 |
88.190(5) |
90 |
90.000(5) |
75.866(5) |
90.000(5) |
V/Å3 |
2456.73(17) |
4325.2 |
2009.7(2) |
8130(4) |
3870.72(19) |
1474.5(12) |
1985.06(16) |
3801(2) |
853.3(7) |
3869(2) |
Z |
4 |
4 |
2 |
8 |
4 |
2 |
4 |
4 |
2 |
8 |
D
c/g cm−3 |
1.751 |
1.561 |
1.498 |
1.560 |
1.431 |
1.785 |
1.620 |
1.621 |
1.601 |
1.578 |
GOF on F2 |
1.115 |
0.953 |
0.797 |
0.990 |
1.148 |
1.018 |
1.034 |
0.985 |
1.025 |
1.144 |
R
1
a[I > 2σ(I)] |
0.0306 |
0.0308 |
0.0462 |
0.0629 |
0.0607 |
0.0517 |
0.0440 |
0.0605 |
0.0691 |
0.0996 |
wR2b[I > 2σ(I)] |
0.0893 |
0.0640 |
0.0613 |
0.1167 |
0.1770 |
0.1014 |
0.1133 |
0.0866 |
0.1566 |
0.1502 |
R
int
|
0.0172 |
0.0280 |
0.0574 |
0.1177 |
0.0213 |
0.0408 |
0.0344 |
0.0683 |
0.0561 |
0.1154 |
Results and discussion
Structure of [Ni2(L)(H2O)3]·5H2O (1)
X-ray crystallography reveals that the asymmetric unit of 1 consists of two Ni(II) ions, one L anion, three coordinating water molecules, and five lattice water molecules. As shown in Fig. 1a, although both Ni1 and Ni2 are six-coordinated in octahedral coordination spheres, their coordination environments are entirely different. Ni1 is coordinated by six carboxylate oxygen atoms from four different L anions, whereas Ni2 is coordinated by three carboxylate oxygen atoms and three water molecules. The Ni–O bond distances are in the range of 1.9665(18)–2.2079(19) Å. Each L anion coordinates to seven Ni(II) ions with its four carboxylate groups. Two of the carboxylate groups exhibit μ2-η1:η1 modes, while the other two display μ1-η1:η1 and μ2-η1:η2 modes, respectively (mode I, Scheme 3). In this way, the L anions bridge the Ni(II) ions to generate a 3D framework (Fig. 1b). In the 3D framework, there are binuclear units, each of which was constructed by two Ni(II) ions and one μ3–O10 atom.
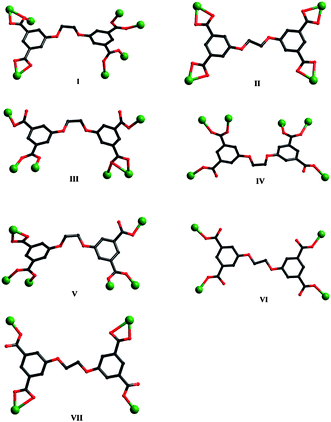 |
| Scheme 3 Coordination modes of L anions in compounds 1–10. | |
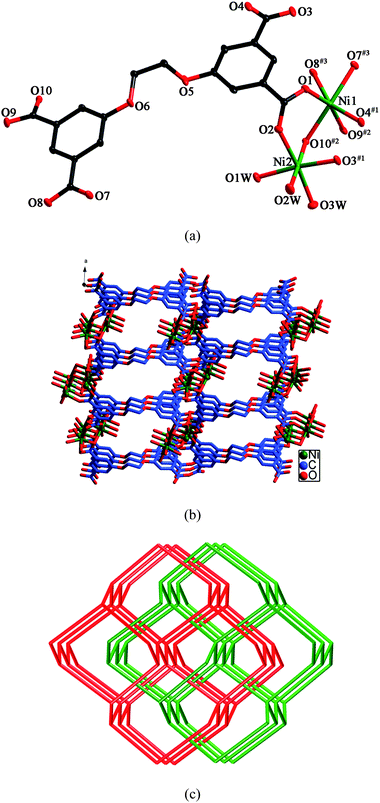 |
| Fig. 1 (a) ORTEP view of 1 showing the local coordination environments of Ni(II) ions with hydrogen atoms and lattice water molecules omitted for clarity (30% probability displacement ellipsoids). Symmetry codes: #1 −x + 1/2, y − 1/2, −z + 3/2; #2 −x + 1/2, y − 1/2, −z + 1/2; #3 x + 1, y, z + 1. (b) Schematic representation of the 3D framework of 1. (c) View of the 2-fold interpenetrating diamond framework of 1. | |
Topologically, if both the binuclear units and the L anions can be considered as 4-connected nodes, the whole 3D framework can be regarded as a 4-connected diamond-like framework. Two identical frameworks interpenetrate each other, giving rise to a 2-fold interpenetrating framework (Fig. 1c).
Structure of [Ni2(L)(pbib)2(H2O)]·H2O (2)
The asymmetric unit of 2 consists of two kinds of Ni(II) ions, two kinds of L anions (LA and LB), two kinds of pbib ligands and two kinds of water molecules. As shown in Fig. 2a, Ni1 is six-coordinated by two nitrogen atoms from two individual pbib ligands and four oxygen atoms from two different L anions and one water molecule in an octahedral coordination sphere. Ni2 also exhibits a distorted octahedral coordination environment, completed by two nitrogen atoms from two individual pbib ligands and four oxygen atoms from two distinct L anions. The Ni–N and Ni–O bond lengths vary from 2.018(2) to 2.061(2) Å and 2.0441(16) to 2.2701(15) Å, respectively. Both LA and LB anions are μ4-bridges, but their coordination modes are different. For LA, all the carboxylate groups adopt μ1-η1:η1 modes (mode II, Scheme 3), while, for LB, two of the carboxylate groups adopt μ1-η1:η1 modes, and the other two adopt μ1-η1:η0 modes (mode VII, Scheme 3). In this fashion, the L anions bridge the Ni(II) ions to yield a 3D framework (Fig. 2b). The two pbib ligands adopt two different conformations with the dihedral angles between the two imidazole rings of 57.3 and 12.5°, respectively, and further coordinate to the 3D framework (Fig. 2c).
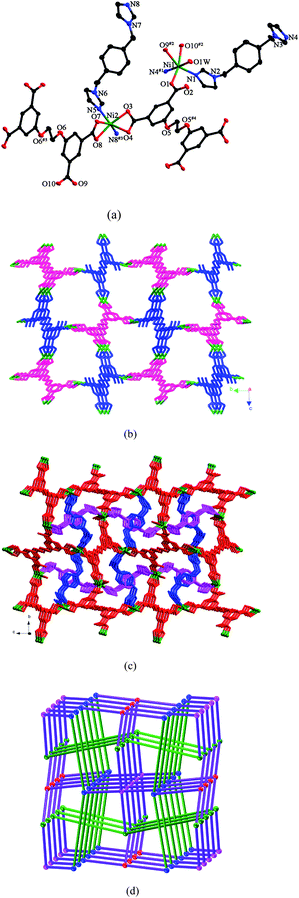 |
| Fig. 2 (a) ORTEP view of 2 showing the local coordination environments of Ni(II) ions with hydrogen atoms and lattice water molecules omitted for clarity (30% probability displacement ellipsoids). Symmetry codes: #1 x, −y + 1/2, z − 1/2; #2 −x, y + 1/2, −z + 1/2; #3 −x + 1, y − 1/2, −z + 1/2; #4 −x − 1, −y, −z + 1; #5 −x − 2, −y, −z. (b) 3D framework constructed by Ni(II) ions, LA (pink) and LB (blue) anions. (c) View of the 3D framework stabilized by two kinds of the pbib ligands. (d) 3D framework with the (86)(73·82·9)(82·92·102)(82·94) topology (the blue, green, red and pink balls represent Ni1, Ni2, LA and LB, respectively, and the green lines represent the pbib ligands). | |
From a topological perspective, if the Ni(II) ions and the L anions are simplified as four-connected nodes, the pbib ligands are reduced to linkers, the 3D framework of 2 can be described as a 4-connected net with the (86)(73·82·9)(82·92·102)(82·94) topology (Fig. 2d).
Structure of [Co2(L)(pbib)1.5]·2.5H2O (3)
The asymmetric unit of 3 consists of two kinds of Co(II) ions, one kind of L anion, two kinds of pbib ligands (pbib-1 and pbib-2) and two and a half water molecules. As shown in Fig. 3a, Co1 is four-coordinated by two nitrogen atoms from two individual pbib-1 ligands and two oxygen atoms from two different L anions in a tetrahedral coordination environment. Co2 is six-coordinated by one nitrogen atom from one pbib-2 ligand and five oxygen atoms from four L anions in an octahedral arrangement. The Co–N and Co–O bond lengths range from 2.009(4) to 2.024(4) Å and 1.984(3) to 2.391(3) Å, respectively. Each L anion coordinates to six Co(II) ions with two of the carboxylate groups in μ1-η1:η0 coordination modes and the other two in μ2-η1:η1 and μ2-η2:η1 modes, respectively (mode III, Scheme 3). The L anions bridge the Co(II) ions to furnish a double layer (Fig. 3b). Each double layer contains two single layers, which share the same binuclear [Co2(COO)4] units. Two symmetry-related Co2 ions and four carboxylate groups from four L anions are involved in the construction of the binuclear unit. The pbib ligands adopt two different conformations with the dihedral angles between the two imidazole rings of 66.6 and 0°, respectively. The double layers are connected by the pbib-1 ligands to generate a 3D framework, which are further strengthened by the pbib-2 ligands (Fig. 3c).
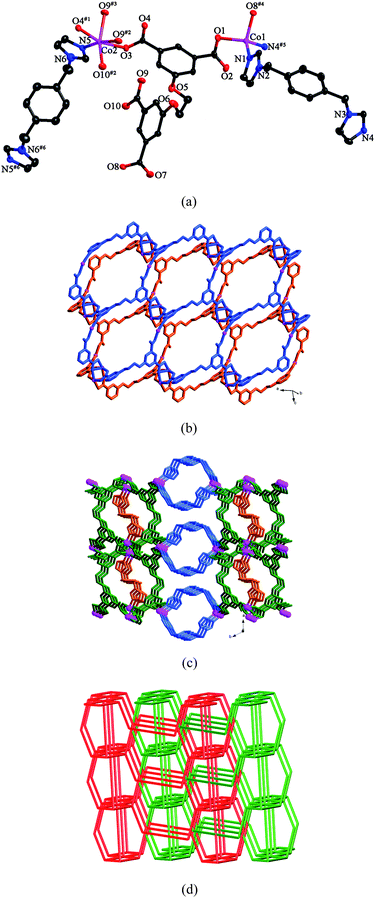 |
| Fig. 3 (a) ORTEP view of 3 showing the local coordination environments of Co(II) ions with hydrogen atoms and lattice water molecules omitted for clarity (30% probability displacement ellipsoids). Symmetry codes: #1 −x + 3, −y, −z + 3; #2 x− 1, y, z; #3 −x + 4, −y, −z + 3; #4 x − 1, y, z − 1; #5 −x + 4, −y + 2, −z + 2; #6 −x + 4, −y, −z + 4. (b) View of the double layer generated by Co(II) ions and L anions. (c) View of the 3D framework of 3. (d) View of the 2-fold interpenetrating framework with the (5·62)(4·54·6)(42·58·85) topology. | |
From the topological point of view, if the Co1 ions are considered as 3-connected nodes, the L anions are regarded as 4-connected nodes, the binuclear units are viewed as 6-connected nodes, and the pbib ligands are reduced to linkers, the whole framework can be simplified as a (3,4,6)-connected net with the (5·62)(4·54·6)(42·58·85) topology. Two identical nets interlock each other to form a 2-fold interpenetrating framework (Fig. 3d).
Structure of [Co2(L)(bbtz)1.5(H2O)5] (4)
Compound 4 crystallizes in the orthorhombic space groupPbcn. The asymmetric unit of 4 consists of two Co(II) ions, one L anion, one and a half bbtz ligands, two coordinating water molecules and three lattice water molecules. As shown in Fig. 4a, both Co1 and Co2 are six-coordinated in octahedral coordination geometries, but their coordination environments are different. Co1 is coordinated by one nitrogen atom from one bbtz ligand and five oxygen atoms from three different L anions and two water molecules. Co2 is coordinated by two nitrogen atoms from two bbtz ligands and four oxygen atoms from three individual L anions and one water molecule. The Co–N bond distances are in the range of 2.126(4)–2.145(4) Å, and the Co–O bond lengths are in the range of 2.007(4)–2.229(3) Å. Adjacent Co1 and Co2 ions are connected by the O1W atom to generate a binuclear unit. Each L anion acts as a μ6-bridge with two of the carboxylate groups in μ1-η1:η0 coordination modes and the other two in μ2-η1:η1 modes (mode IV, Scheme 3). In this way, the L anions connect the binuclear units to form a double layer (Fig. 4b). Notably, there are two kinds of bbtz ligands (bbtz-1 and bbtz-2) in the crystal structure of 4, with the dihedral angles between the two triazole rings of 66.6 and 0°, respectively. The bbtz-1 ligands link the double layer to yield a 3D framework, which is further connected by the bbtz-2 ligands (Fig. 4c).
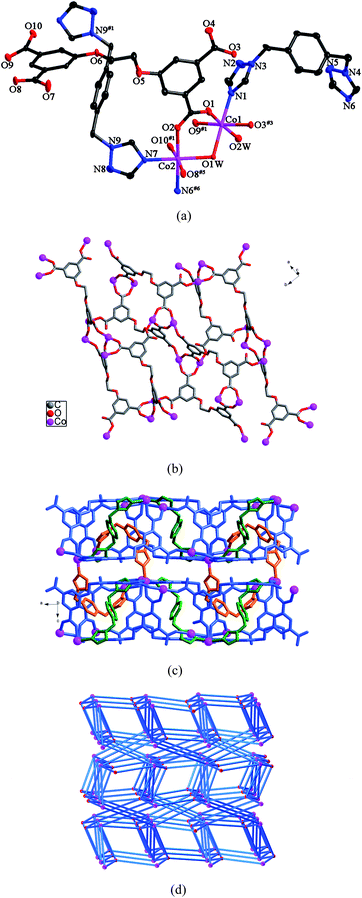 |
| Fig. 4 (a) ORTEP view of 4 showing the local coordination environments of Co(II) ions with hydrogen atoms and lattice water molecules omitted for clarity (30% probability displacement ellipsoids). Symmetry codes: #1 −x, −y + 1, −z + 1; #3 x + 1/2, −y + 1/2, −z + 1; #5 x + 1/2, y + 1/2, −z + 1/2; #6 x − 1/2, −y + 1/2, −z + 1. (b) View of the double layer constructed by Co(II) ions and L anions. (c) 3D framework of 4. (d) 3D framework with the (3·4·5·63)(32·42·5·613·73) topology. | |
Topologically, if the L anions are considered as 4-connected nodes, the binuclear units are viewed as 7-connected nodes and the bbtz ligands are regarded as linkers, the 3D framework can be described as a (4,7)-connected net with the (3·4·5·63)(32·42·5·613·73) topology (Fig. 4d).10
Structure of [Ni(L)0.5(biim-3)0.5(H2O)(CH3CH2OH)0.5]·2H2O (5)
X-ray crystallography reveals that the asymmetric unit of 5 contains two Ni(II) ions, half a L anion, half a biim-3 ligand, half an ethanol molecule and three water molecules. As shown in Fig. 5a, Ni1 is six-coordinated by two nitrogen atoms from two different biim-3 ligands and four oxygen atoms from two individual L anions and two water molecules in an octahedral coordination sphere. Ni2 is five-coordinated by five oxygen atoms from four different L anions and one ethanol molecule in a trigonal bipyramid arrangement. The Ni–N bond length is 2.037(14) Å and the Ni–O bond lengths range from 1.989(6) to 2.125(3) Å. Each L anion acts as a μ6-bridge with two of the carboxylate groups in μ2-η1:η1 coordination modes and the other two in μ1-η1:η0 fashions (mode IV, Scheme 3). Two symmetry-related Ni2 ions are connected by the carboxylate groups to form a binuclear unit. The L anions connect the Ni1 ions and the binuclear units to yield a 3D framework (Fig. 5b). The biim-3 ligands further coordinate to the 3D framework with the dihedral angle between the two imidazole rings of 49.3° (Fig. 5c).
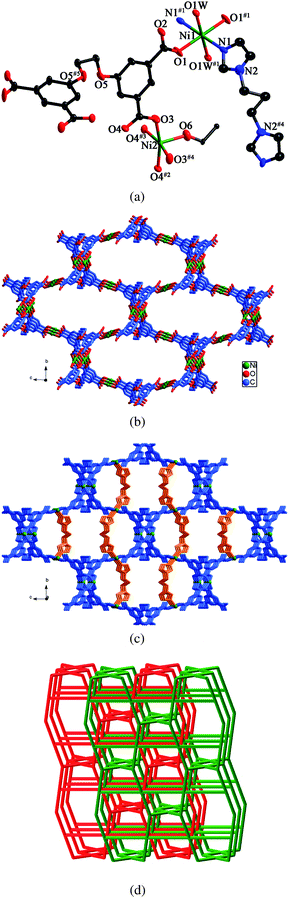 |
| Fig. 5 (a) ORTEP view of 5 showing the local coordination environments of Ni(II) ions with hydrogen atoms and lattice water molecules omitted for clarity (30% probability displacement ellipsoids). Symmetry codes: #1 −x + 3/2, −y + 3/2, −z + 1; #2 −x + 2, −y + 2, −z + 2; #3 −x + 2, y, −z + 2; #4 x, −y + 2, z; #5 −x + 1, y, −z + 2. (b) View of the 3D framework formed by Ni(II) ions and L anions. (c) 3D framework stabilized by the biim-3 ligands. (d) View of the 2-fold interpenetrating framework with the (52·62·82)(42·82·92)(4·54·6) topology. | |
Topologically, if the Ni(II) ions, the binuclear units and the L anions are considered as 4-connectors, and the biim-3 ligands are regarded as linkers, the whole framework can be simplified as a 4-connected net with the (52·62·82)(42·82·92)(4·54·6) topology. Two identical frameworks interpenetrate each other to yield a 2-fold interpenetrating framework (Fig. 5d).
Structure of [Zn3(L)(biim-3)(OH)2] (6)
Single crystal X-ray analysis reveals that compound 6 crystallizes in a triclinic crystal system of the P
space group. The asymmetric unit consists of three Zn(II) ions, one L anion, one biim-3 ligand and two hydroxyl groups. As shown in Fig. 6a, Zn1 is four-coordinated by one nitrogen atom from one biim-3 ligand and three oxygen atoms from one hydroxyl group and two different L anions in a tetrahedral coordination environment. Zn2 is five-coordinated by one nitrogen atom from one biim-3 ligand and four oxygen atoms from one hydroxyl group and two individual L anions in a trigonal bipyramid coordination sphere. Zn3 is four-coordinated by four oxygen atoms from one hydroxyl group and three different L anions in a tetrahedral arrangement. The Zn–N bond lengths are in the range of 1.985(5)–2.004(4) Å, and the Zn–O bond distances are in the range of 1.896(4)–2.431(4) Å. The two hydroxyl groups bridge the three Zn(II) ions to generate a trinuclear motif [Zn3(μ2–O)2]. The carboxylate groups of L anions, in μ1-η1:η0, μ1-η1:η1 and μ2-η1:η1 coordination modes (mode V, Scheme 3), bridge the trinuclear units to form a 3D framework (Fig. 6b). The biim-3 ligands further connect the 3D framework with the dihedral angle between the two imidazole rings of 79.5° (Fig. 6c).
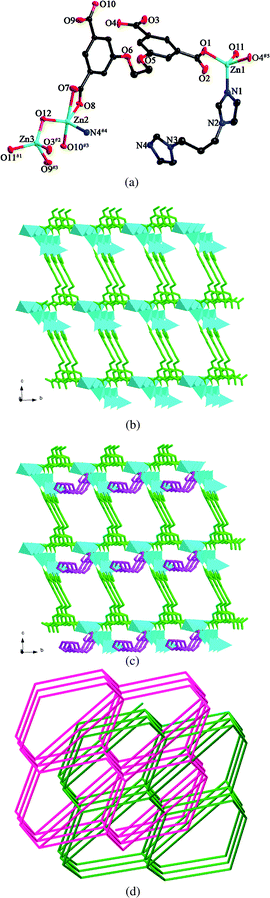 |
| Fig. 6 (a) ORTEP view of 6 showing the local coordination environments of Zn(II) ions with hydrogen atoms omitted for clarity (30% probability displacement ellipsoids). Symmetry codes: #1 x − 1, y + 1, z − 1; #2 x, y + 1, z − 1; #3 x, y + 1, z; #4 x, y, z − 1. (b) 3D framework formed by Zn(II) ions and L anions. (c) 3D framework stabilized by the biim-3 ligands. (d) View of the 2-fold interpenetrating framework. | |
From a topological perspective, if the L anions can be regarded as 4-connectors, the trinuclear units are viewed as 6-connectors, and the biim-3 ligands are reduced to linkers, the whole framework can be considered as a (4,6)-connected net with the (54·62) (58·66·7) topology. Two independent nets interpenetrate each other, giving rise to a 2-fold interpenetrating framework (Fig. 6d).
Structure of [Ni(L)0.5(btp)(H2O)2]·H2O (7)
The asymmetric unit of 7 contains one crystallographically independent Ni(II) ion, half a L anion, one btp ligand and three water molecules. As shown in Fig. 7a, Ni1 is six-coordinated by two nitrogen atoms from two distinct btp ligands and four oxygen atoms from two different L anions and two water molecules in a distorted octahedral coordination environment. The Ni–N bond lengths are 2.071(3) and 2.087(3) Å, and the Ni–O bond distances vary from 2.059(2) to 2.113(2) Å. The carboxylate groups of L anions, in μ1-η1:η0 coordination modes (mode VI, Scheme 3), link the Ni(II) ions to generate a 2D sheet (Fig. 7b). Further, the btp ligands bridge the sheets in pairs to yield a 3D framework (Fig. 7c). The dihedral angle between the two triazole rings of the btp ligand is 79.8°.
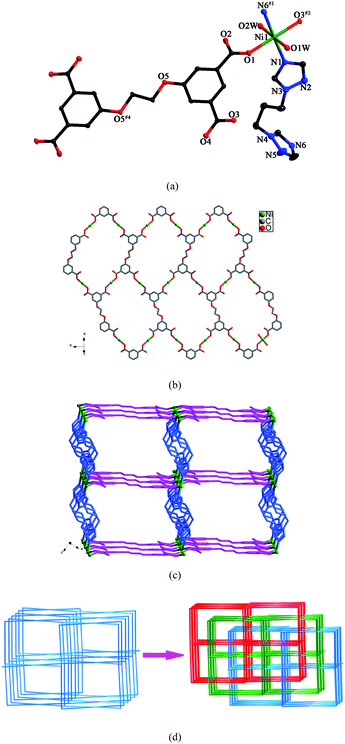 |
| Fig. 7 (a) ORTEP view of 7 showing the local coordination environment of Ni(II) ion with hydrogen atoms and lattice water molecules omitted for clarity (30% probability displacement ellipsoids). Symmetry codes: #1 x + 1, −y + 1/2, z + 1/2; #2 −x + 3, y + 1/2, −z + 5/2; #4 −x + 1, −y, −z + 3. (b) Schematic representation of the 2D layer generated by Ni(II) ions and L anions. (c) 3D framework of 7. (d) View of the 3-fold interpenetrating framework of 7. | |
From a topological point of view, if the Ni(II) ions and the L anions are regarded as 4-connected nodes, the btp ligands are reduced to linkers, the whole structure can be described as a 4-connected framework with the 64·82 topology. Three independent nets interlock each other to form a 3-fold interpenetrating framework (Fig. 7d).
Structure of [Zn2(L)(btp)2]·3H2O (8)
There are two crystallographically independent Zn(II) ions, two halves L anions, two btp ligands and three water molecules in the asymmetric unit of 8. As shown in Fig. 8a, Zn1 is four-coordinated by two nitrogen atoms from two different btp ligands and two oxygen atoms from two individual L anions in a tetrahedral coordination environment. Zn2 displays a similar tetrahedral arrangement to Zn1, coordinated by two nitrogen atoms from two distinct btp ligands and two oxygen atoms from two different L anions. The Zn–N bond lengths range from 1.999(4) to 2.050(4) Å, and the Zn–O bond distances vary from 1.946(3) to 1.993(3) Å. The carboxylate groups of L anions, in μ1-η1:η0 coordination modes (mode VI, Scheme 3), connect the Zn(II) ions to yield a 2D wavelike layer (Fig. 8b). Further, the 2D layers are extended by the btp ligands into a 3D framework (Fig. 8c). In the structure, there are two kinds of btp ligands with the dihedral angles between the two triazole rings of 73.6 and 82.5°, respectively.
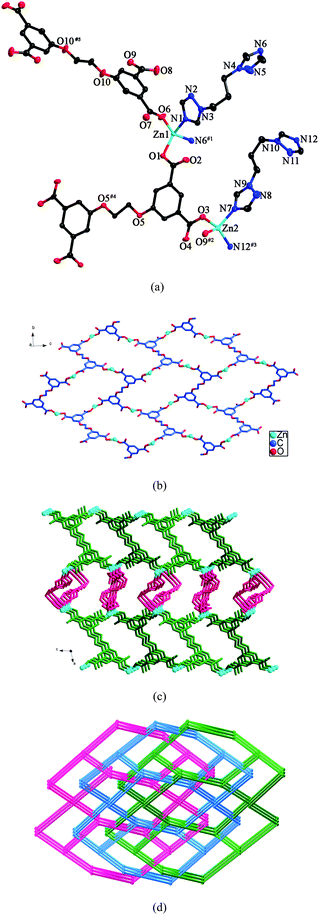 |
| Fig. 8 (a) ORTEP view of 8 showing the local coordination environments of Zn(II) ions with hydrogen atoms and lattice water molecules omitted for clarity (30% probability displacement ellipsoids). Symmetry codes: #1 −x + 3/2, y + 1/2, −z + 1/2; #2 x, y, z − 1 #3 −x + 3/2, y + 1/2, −z − 1/2; #4 −x, −y + 1, −z; #5 −x, −y, −z + 1. (b) View of the 2D wavelike layer formed by Zn(II) ions and L anions. (c) 3D framework of 8. (d) View of the 3-fold interpenetrating framework with the (64·82)2(66)2 topology. | |
Topologically, if the Zn(II) ions and the L anions can be considered as 4-connected nodes, the btp ligands are regarded as linkers, the 3D framework can be simplified as a 4-connected net with the (64·82)2(66)2 topology. Three independent nets interpenetrate each other, generating a 3-fold interpenetrating framework (Fig. 8d).
Structure of [Co(L)0.5(btb)0.5(H2O)2]·1.5H2O (9)
Compound 9 displays a 2D layer structure. The asymmetric unit of 9 contains one Co(II) ion, half a L anion, half a btb ligand, and three and a half water molecules. As shown in Fig. 9a, Co1 is six-coordinated by one nitrogen atom from one btb ligand and five oxygen atoms from three individual L anions and two water molecules in a distorted octahedral coordination sphere. The Co–N bond length is 2.066(5) Å and the Co–O bond lengths are in the range of 2.061(4)–2.216(4) Å. Each L anion acts as a μ4-bridge with two of the carboxylate groups in μ1-η1:η0 coordination modes and the other two in μ1-η1:η1 fashions (mode VII, Scheme 3). The L anions connect the Co(II) ions to form 1D infinite chains (Fig. 9b), which are further connected by the btb ligands to generate a 2D layer (Fig. 9c). The dihedral angle between the two triazole rings of the btb ligands is 0°
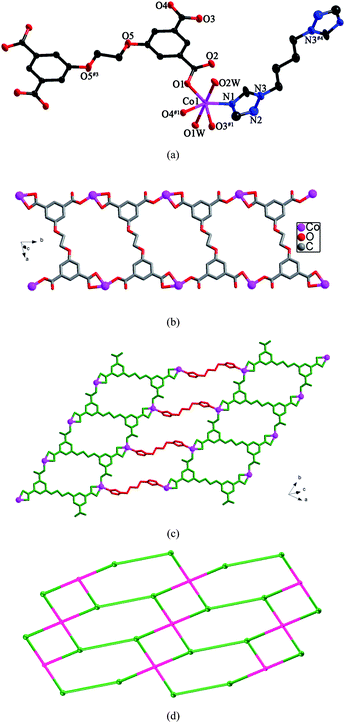 |
| Fig. 9 (a) ORTEP view of 9 showing the local coordination environment of Co(II) ion with hydrogen atoms and lattice water molecules omitted for clarity (30% probability displacement ellipsoids). Symmetry codes: #1 x, y − 1, z; #2 x, y + 1, z; #3 −x, −y + 2, −z; #4 −x + 2, −y + 1, −z + 1. (b) View of the 1D infinite chain furnished by Co(II) ions and L anions. (c) View of the 2D layer of 9. (d) View of the (3,4)-connected net with the (4·62)(42·62·82) topology. | |
From a topological point of view, if the Co(II) ions are considered as 3-connected nodes, the L anions are regarded as four-connected nodes and the btb ligands are regarded as linkers, the 2D layer can be simplified as a (3,4)-connected net with the (4·62)(42·62·82) topology (Fig. 9d).
Structure of [Zn(L)0.5(btb)]·0.5H2O (10)
Compound 10 is a 3-fold interpenetrating framework. The asymmetric unit of 10 contains one Zn(II) ion, half a L anion, one btb ligand and half a water molecule. As shown in Fig. 10a, Zn1 is five-coordinated by two nitrogen atoms from two individual btb ligands (Zn(1)–N(1) = 2.014(7) and Zn(1)–N(6)#2 = 2.040(7) Å) and three oxygen atoms from two different L anions (Zn(1)–O(1) = 1.947(5), Zn(1)–O(3)#1 = 2.017(6) and Zn(1)–O(4)#1 = 2.480(8) Å) in a trigonal bipyramid arrangement. The carboxylate groups of L anions, in μ1-η1:η0 coordination modes (mode VII, Scheme 3), link the Zn(II) ions to form a 2D layer (Fig. 10b). Further, the btb ligands connect the layers in pairs to yield a 3D framework. The dihedral angle between the two triazole rings of the btb ligands is 8.0° (Fig. 10c).
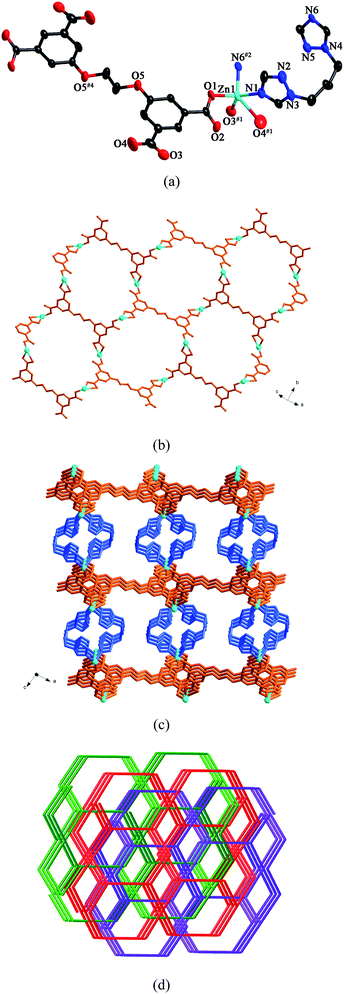 |
| Fig. 10 (a) ORTEP view of 10 showing the local coordination environments of Zn(II) ions with hydrogen atoms and lattice water molecules omitted for clarity (30% probability displacement ellipsoids). Symmetry codes: #1 −x + 3/2, y + 1/2, −z + 3/2; #2 −x + 1, y, −z + 1/2; #4 −x + 2, −y, −z + 1. (b) 2D layer furnished by Zn(II) ions and L anions. (c) View of the 3D framework of 10. (d) View of the 3-fold interpenetrating framework of 10. | |
From a topological perspective, the Zn(II) ions can be reduced to 3-connected nodes, the L anions can be considered as 4-connected nodes and the btb ligands can be regarded as linkers. Thus, the 3D framework of 10 can be simplified as a (3,4)-connected net with the (62·8)(62·82·102) topology. Three independent nets interlock each other to yield a 3-fold interpenetrating framework (Fig. 10d).
Effects of the L Anions on the structures of the compounds.
From the structure description above, we can see that the L anion displays diverse coordination modes in compounds 1–10 (Scheme 3). The difference of the coordination modes can be attributed to the discrimination of the dihedral angles (θ) between the two phenyl rings of L. From Table 2, we can find that when the θ angle is 0°, each L anion coordinates to four metal ions (compounds 2 and 7–10). When the θ angles are in the range of 63–78°, each L anion coordinates to six metal ions (compounds 3–6). When the θ angle is greater than 80°, each L anion coordinates to seven metal ions (compound 1). These led to the formation of ten different structures of 1–10.
Table 2
θ angles and N in compounds 1–10 (θ represent dihedral angles between the two phenyl rings of the L anion, and N represent numbers of metal ions coordinated to each L anion)
Compound |
1
|
2
|
3
|
4
|
5
|
6
|
7
|
8
|
9
|
10
|
θ/° |
80.4 |
0 |
70.0 |
77.6 |
75.9 |
63.5 |
0 |
0 |
0 |
0 |
N |
7 |
4 |
6 |
6 |
6 |
6 |
4 |
4 |
4 |
4 |
Effects of the N-donor ligands on the structures of the compounds.
The N-donor ligands have a significant effect on the resultant structures of the compounds. Compound [Ni2(L)(H2O)3]·5H2O (1) has a 2-fold interpenetrating diamond-like framework. However, when the N-donor ligands were introduced into the reaction system of 1, structurally-different compounds 2–10 were obtained.
Compounds 3–5 and 7, as well as 6 and 8 display the effects of a heteroatom in the five-membered rings on the structures. Here, we take compounds [Co2(L)(pbib)1.5]·2.5H2O (3) and [Co2(L)(bbtz)1.5(H2O)5] (4) for example. Compared with pbib, bbtz has an extra nitrogen atom in the 2-position of the five-membered ring, which replaces the carbon atom of the pbib ligand. Consequently, although both pbib and bbtz are bidentate ligands, their structures are different. In compound 3, the L anions bridge the Co(II) ions to form a double layer. The double layers are connected by the pbib-1 ligands to generate a 3D framework, which are further strengthened by the pbib-2 ligands. Two identical nets interlock each other to form a 2-fold interpenetrating framework. In compound 4, the L anions connect the Co(II) ions to generate a double layer. The bbtz-1 ligands link the double layer to yield a 3D framework, which is further connected by the bbtz-2 ligands.
Compounds 8 and 10 exhibit the effects of the length of the N-donor ligands on the structures. Compared with btp, btb has a longer alkyl spacer. In 8, the L anions connect the Zn(II) ions to yield a 2D wavelike layer, which are further extended by the btp ligands to yield a 3D framework with the (64·82)2(66)2 topology. Three nets interpenetrate in a 3-fold mode. Whereas, in 10, the L anions connect the Zn(II) ions to form a 2D layer. Further, the btb ligands link the layers in pairs to yield a 3D framework with the (62·8)(62·82·102) topology. Three independent nets interlock each other to form a 3-fold interpenetrating framework.
Compounds 2 and 5 show the effects of the spacers on the structures. When the central phenyl group of pbib in 2 is replaced by the carbon atom, a structurally different compound 5 was obtained. In 2, the L anions bridge the Ni(II) ions to yield a 3D framework, and the two pbib ligands further stabilize the 3D framework. Whereas, in 5, the L anions connect the Ni(II) ions to yield a 3D framework, which is further strengthened by the biim-3 ligands. Two identical nets interpenetrate in a 2-fold mode.
From the results above, we can see that the flexible bridging N-donor ligands used in this work are very easy to form high dimensional structures. However, when the rigid or chelating N-donor ligands were used, the compounds are inclined to form low dimensional structures.11 For example, in the reported compound [(Me-bpa)Cu(Cl)2] (Me-bpa = N-methyl-[bis(2-pyridyl)methyl]amine), the chelating N-donor Me-bpa ligand and the Cl− anions coordinate with the Cu(II) ions to form a 0D structure.11c The formation of the low dimensional structure may be attributed to the steric hindrance of the chelating ligand Me-bpa.
Effects of the central metals on the frameworks.
It is noteworthy that the central metals also play important roles in the formation of the final structures. The varieties in coordination number of the central metals are the main reasons for the structural differences. The coordination numbers of the Zn(II) ions are lower than those of the Co(II) and Ni(II) ions. The Zn(II) ions in compounds 6, 8, and 10 adopt the coordination numbers of 4, except that Zn2 in compound 6 shows the coordination number of 5. The Co(II) ions in compounds 3, 4 and 9 exhibit the coordination numbers of 6, except that Co1 in compound 3 shows the coordination number of 4. The Ni(II) ions in compounds 1, 2, 5 and 7 exhibit the coordination numbers of 6, except that Ni2 in compound 5 shows the coordination number of 5.
Luminescent properties
Luminescent properties of compounds with d10 metal centers have fascinated much attention because of their potential applications in chemical sensors, electroluminescent display, and photochemistry.12 Therefore, in the present work, the photoluminescent properties of compounds 6, 8 and 10 have been studied in the solid state at room temperature. The emission peaks are shown in Fig. 11.
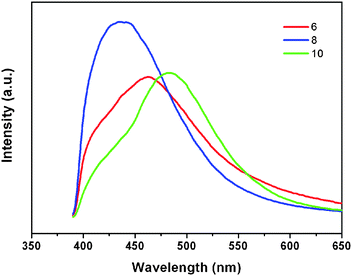 |
| Fig. 11 Solid-state photoluminescent spectra of 6, 8, and 10 at room temperature. | |
The free H4L shows the emission maximum at 429 nm (λex = 328 nm),6 which may be caused by the π*–n or π*–π transition.13 The emission peaks of compounds 6, 8 and 10 are located at 463 nm (λex = 361 nm), 435 nm (λex = 354 nm), and 484 nm (λex = 350 nm), respectively. The emission band of 8 is near to that of the free H4L, so the emission band of this compound can probably be attributed to the intraligand fluorescent emission.14 The emission bands of 6 and 10 are red-shifted by 34 nm and 55 nm compared with the free H4L ligand. According to the literature,15 the Zn(II) ions are difficult to oxidize or reduce because of the d10 configuration. As a result, the emissions of these compounds are neither MLCT nor LMCT.14 They can probably be attributed to the intraligand transitions.16
Optical band gaps
Some coordination polymers have been reported to be promising semiconductors in the previous literature.17 Inspired by these documents, we investigated the conductivity potentials of compounds 1–10. The measurement of diffuse reflectivity for powder samples of 1–10 were carried out to obtain the band gaps (Eg), which was determined as the intersection point between the energy axis and the line extrapolated from the linear portion of the adsorption edge in a plot of Kubelka-Munk function F against energy E.18 The F versus E plots are shown in Fig. S3 and S4 (see ESI†). For compounds 1–10, the corresponding well-defined optical absorption associated with band gaps (Eg) can be assessed at 3.51, 3.49, 3.44, 3.61, 3.36, 2.59, 3.61, 3.37, 3.54 and 3.20 eV, respectively. The reflectance spectra indicate the nature of semiconductivities with wide band gaps for compounds 1–10.
Thermal analyses
Thermogravimetric analyses are carried out to study the thermal behaviors of compounds 1–10. The experiments were performed under N2 atmosphere with a heating rate of 10 °C min−1, as shown in Fig. S5 and S6 (see ESI†).
The first weight losses corresponding to the release of water molecules are observed before 214 °C for compound 1 (obsd 21.5%, calcd 22.2%), 227 °C for compound 2 (obsd 4.2%,calcd 3.5%), 141 °C for compound 3 (obsd 7.6%, calcd 5.0%), 234 °C for compound 4 (obsd 8.4%, calcd 9.4%), 128 °C for compound 7 (obsd 13.9%, calcd 11.2%), 101 °C for compound 8 (obsd 7.6%, calcd 5.8%), 146 °C for compound 9 (obsd 14.5%, calcd 15.3%), and 106 °C for compound 10 (obsd 3.6%, calcd 1.9%). For compound 5, the weight loss before 158 °C is attributed to the release of water molecules and ethanol molecules (obsd 12.6%, calcd 9.2%). For compound 6, the weight loss before 31 °C is attributed to the release of hydroxyl groups (obsd 5.5%, calcd 4.3%). The departure of organic components occurred at the ranges of 214–547 °C for 1, 227–449 °C for 2, 141–501 °C for 3, 234–582 °C for 4, 158–463 °C for 5, 373–485 °C for 6, 128–451 °C for 7, 101–513 °C for 8, 146–759 °C for 9, and 333–459 °C for 10, respectively. The remaining weight may correspond to the formation of NiO for compound 1 (obsd 25.8%, calcd 23.1%), compound 2 (obsd 16.2%, calcd 14.7%), compound 5 (obsd 11.2%, calcd 9.0%), and compound 7 (obsd 17.8%, calcd 15.4%); CoO for compound 3 (obsd 18.2%, calcd 16.5%), compound 4 (obsd 16.2%, calcd 15.7%), and compound 9 (obsd 19.9%, 18.2%); ZnO for compound 6 (obsd 34.5%, calcd 30.7%), compound 8 (obsd 20.4%, calcd 17.5%), and compound 10 (obsd 19.9%, calcd 17.7%).
Conclusions
Ten new coordination polymers were successfully synthesized under hydrothermal conditions by varying the N-donor ligands and central metals. The structural differences of the compounds indicate that the organic ligands and the metal centers have great effects on the resultant topologies. The results also show that the flexible bridging N-donor ligands are good candidates for construction of high dimensional coordination polymers. The optical band gaps of 1–10 indicate that these compounds are potential wide gap semiconductive materials. The photoluminescent behaviors of the compounds 6, 8 and 10 imply that these complexes may be good optical materials.
Acknowledgements
We thank the National Science Foundation of China (21001023 and 21071028), the Specialized Research Fund for the Doctoral Program of Higher Education, the Science Foundation of Jilin Province (20090137 and 20100109), the Fundamental Research Funds for the Central Universities, and the Training Fund of NENU'S Scientific Innovation Project for support.
Referrences
-
(a) J. R. Li, R. J. Kuppler and H. C. Zhou, Chem. Soc. Rev., 2009, 38, 1477 RSC;
(b) O. K. Farha, C. D. Malliakas, M. G. Kanatzidis and J. T. Hupp, J. Am. Chem. Soc., 2009, 132, 950 CrossRef;
(c) S. Kitagawa, R. Kitaura and S. Noro, Angew. Chem., Int. Ed., 2004, 43, 2334 CrossRef CAS;
(d) J. W. Ko, K. S. Min and M. P. Suh, Inorg. Chem., 2002, 41, 2151 CrossRef CAS;
(e) G. Ferey, C. Mellot-Draznieks, C. Serre and F. Millange, Acc. Chem. Res., 2005, 38, 217 CrossRef CAS;
(f) L. Pan, D. H. Olson, L. R. Ciemnolonski, R. Heddy and J. Li, Angew. Chem., Int. Ed., 2006, 45, 616 CrossRef CAS;
(g) D. Bradshaw, J. B. Claridge, E. J. Cussen, T. J. Prior and M. J. Rosseinsky, Acc. Chem. Res., 2005, 38, 273 CrossRef CAS;
(h) L. Pan, M. B. Sander, X. Y. Huang, J. Li, M. Smith, E. Bittner, B. Bockrath and J. K. Johnson, J. Am. Chem. Soc., 2004, 126, 1308 CrossRef CAS.
-
(a) K. Adachi, Y. Sugiyama, K. Yoneda, K. Yamada, K. Nozaki, A. Fuyuhiro and S. Kawata, Chem.–Eur. J., 2005, 11, 6616 CrossRef CAS;
(b) T. Lee and M. S. Lin, Cryst. Growth Des., 2007, 7, 1803 CrossRef CAS;
(c) J. Pasan, J. Sanchiz, F. Lloret, M. Julve and C. Ruiz-Perez, CrystEngComm, 2007, 9, 478 RSC;
(d) L. F. Ma, Y. Y. Wang, L. Y. Wang, J. Q. Liu, Y. P. Wu, J. G. Wang, Q. Z. Shi and S. M. Peng, Eur. J. Inorg. Chem., 2008, 693 CrossRef CAS;
(e) A. R. Choudhury and T. N. Guru Row, CrystEngComm, 2006, 8, 265 RSC.
-
(a) W. H. Zhang, Y. Y. Wang, E. K. Lermontova, G. P. Yang, B. Liu, J. C. Jin, Z. Dong and Q. Z. Shi, Cryst. Growth Des., 2010, 10, 76 CrossRef CAS;
(b) Z. B. Han, X. N. Cheng and X. M. Chen, Cryst. Growth Des., 2005, 5, 695 CrossRef CAS;
(c) L. F. Ma, L. Y. Wang, J. L. Hu, Y. Y. Wang and G. P. Yang, Cryst. Growth Des., 2009, 9, 5334 CrossRef CAS.
-
(a) T. M. Reineke, M. Eddaoudi, D. Moler, M. O'Keeffe and O. M. Yaghi, J. Am. Chem. Soc., 2000, 122, 4843 CrossRef CAS;
(b) Q. R. Fang, G. S. Zhu, M. Xue, Q. L. Zhang, J. Y. Sun, X. D. Guo, S. L. Qiu, S. T. Xu, P. Wang, D. J. Wang and Y. Wei, Chem.–Eur. J., 2006, 12, 3754 CrossRef CAS;
(c) S. N. Wang, J. F. Bai, H. Xing, Y. Z. Li, Y. Song, Y. Pan, M. Scheer and X. Z. You, Cryst. Growth Des., 2007, 7, 747 CrossRef CAS;
(d) J. Yang, J. F. Ma, Y. Y. Liu, J. C. Ma and S. R. Batten, Inorg. Chem., 2007, 46, 6542 CrossRef CAS;
(e) Z. R. Pan, H. G. Zheng, T. W. Wang, Y. Song, Y. Z. Li, Z. J. Guo and S. R. Batten, Inorg. Chem., 2008, 47, 9528 CrossRef CAS.
-
(a) A. Karmakar and I. Goldberg, CrystEngComm, 2011, 13, 339 RSC;
(b) S. M. Hawxwell, G. M. Espallargas, D. Bradshaw, M. J. Rosseinsky, T. J. Prior, A. J. Florence, J. Van de Streeke and L. Brammer, Chem. Commun., 2007, 1532 RSC;
(c) J. J. Perry, V. C. Kravtsov, G. J. McManus and M. J. Zaworotko, J. Am. Chem. Soc., 2007, 129, 10076 CrossRef CAS;
(d) Z. Pan, H. Zheng, T. Wang, Y. Song, Y. Li, Z. Guo and S. R. Batten, Inorg. Chem., 2008, 47, 9528 CrossRef CAS;
(e) A. Zafar, J. Yang, S. J. Geib and A. D. Hamilton, Tetrahedron Lett., 1996, 37, 2327 CrossRef CAS;
(f) Q. Wenge, A. P. Jason, W. Łukasz, E. Mohamed and J. Z. Michael, Chem. Commun., 2010, 46, 8734 RSC;
(g) L. L. Qu, Y. L. Zhu, Y. Z. Li, H. B. Du and X. Z. You, Cryst. Growth Des., 2011, 11, 2444 CrossRef CAS;
(h) A. Karmakar and I. Goldberg, CrystEngComm, 2011, 13, 350 RSC.
- W. Q. Kan, Y. Y. Liu, J. Yang, Y. Y. Liu and J. F. Ma, CrystEngComm, 2011, 13, 4256 RSC.
-
(a) S. L. Li, Y. Q. Lan, J. F. Ma, J. Yang, J. Wei, L. P. Zhang and Z. M. Su, Cryst. Growth Des., 2008, 8, 675 CrossRef CAS;
(b) J. F. Ma, J. Yang, G. L. Zheng, L. Li, Y. M. Zhang, F. F. Li and J. F. Liu, Polyhedron, 2004, 23, 553 CrossRef CAS;
(c) J. Yang, J. F. Ma, Y. Y. Liu, S. L. Li and G. L. Zheng, Eur. J. Inorg. Chem., 2005, 2174 CrossRef CAS;
(d) Y. Y. Liu, J. F. Ma, J. Yang and Z. M. Su, Inorg. Chem., 2007, 46, 3027 CrossRef CAS;
(e) W. L. Zhang, Y. Y. Liu, J. F. Ma, H. Jiang and J. Yang, Polyhedron, 2008, 27, 3351 CrossRef CAS;
(f) Y. Y. Liu, J. F. Ma, J. Yang, J. C. Ma and Z. M. Su, CrystEngComm, 2008, 10, 894 RSC;
(g) J. Yang, J. F. Ma, Y. Y. Liu, J. C. Ma, H. Q. Jia and N. H. Hu, Eur. J. Inorg. Chem., 2006, 6, 1208 CrossRef;
(h) J. F. Ma, J. Yang, G. L. Zheng, L. Li and J. F. Liu, Inorg. Chem., 2003, 42, 7531 CrossRef CAS;
(i) F. F. Li, J. F. Ma, S. Y. Song, J. Yang, Y. Y. Liu and Z. M. Su, Inorg. Chem., 2005, 44, 9374 CrossRef CAS.
-
G. M. Sheldrick, SHELXS-97: Programs for X-ray Crystal Structure Solution; University of Göttingen: Göttingen, Germany, 1997 Search PubMed.
-
G. M. Sheldrick, SHELXL-97: Programs for X-ray Crystal Structure Refinement; University of Göttingen: Göttingen, Germany, 1997 Search PubMed.
-
(a) G. E. Kostakis, G. Abbas, C. E. Anson and A. K. Powell, CrystEngComm, 2009, 11, 82 RSC;
(b) G. X. Liu, R. Y. Huang, L. F. Huang, X. J. Kong and X. M. Ren, CrystEngComm, 2009, 11, 643 RSC.
-
(a) M. Khanpour, A. Morsali and P. Retailleau, Polyhedron, 2010, 29, 1520 CrossRef CAS;
(b) Y. G. Li, Q. Wu, L. Lecren and R. Clérac, J. Mol. Struct., 2008, 890, 339 CrossRef CAS;
(c) J. Astner, M. Weitzer, S. P. Foxon, S. Schindler, F. W. Heinemann, J. Mukherjee, R. Gupta, V. Mahadevan and R. Mukherjee, Inorg. Chim. Acta, 2008, 361, 279 CrossRef CAS;
(d) L. Hashemi, A. Morsali and P. Retailleau, Inorg. Chim. Acta, 2011, 367, 207 CrossRef CAS.
-
(a) J. E. McGarrah, Y. J. Kim, M. Hissler and R. Eisenberg, Inorg. Chem., 2001, 40, 4510 CrossRef CAS;
(b) Q. Wu, M. Esteghamatian, N. X. Hu, Z. Popovic, G. Enright, Y. Tao, M. D'Iorio and S. Wang, Chem. Mater., 2000, 12, 79 CrossRef CAS;
(c) G. D. Santis, L. Fabbrizzi, M. Licchelli, A. Poggi and A. Taglietti, Angew. Chem., Int. Ed. Engl., 1996, 35, 202 CrossRef.
-
(a) X. J. Zhang, L. P. Jin and S. Gao, Inorg. Chem., 2004, 43, 1600 CrossRef;
(b) J. Yang, Q. Yue, G. D. Li, J. J. Cao, G. H. Li and J. S. Chen, Inorg. Chem., 2006, 45, 2857 CrossRef CAS;
(c) A. Thirumurugan and S. Natarajan, J. Chem. Soc., Dalton Trans., 2004, 2923 RSC;
(d) X. J. Zheng, L. P. Jin, S. Gao and S. Lu, New J. Chem., 2005, 29, 798 RSC.
-
(a) L. Y. Zhang, J. P. Zhang, Y. Y. Lin and X. M. Chen, Cryst. Growth Des., 2006, 6, 1684 CrossRef CAS;
(b) Q. Chu, G. X. Liu, Y. Q. Huang, X. F. Wang and W. Y. Sun, Dalton Trans., 2007, 4302 RSC;
(c) X. L. Wang, C. Qin, E. B. Wang, Y. G. Li, N. Hao, C. W. Hu and L. Xu, Inorg. Chem., 2004, 43, 1850 CrossRef CAS;
(d) Z. L. Chen, Y. Su, W. Xiong, L. X. Wang, F. P. Liang and M. Shao, CrystEngComm, 2009, 11, 318 RSC.
-
(a) L. Wen, Y. Li, Z. Lu, J. Lin, C. Duan and Q. Meng, Cryst. Growth Des., 2006, 6, 530 CrossRef CAS;
(b) L. Wen, Z. Lu, J. Lin, Z. Tian, H. Zhu and Q. Meng, Cryst. Growth Des., 2007, 7, 93 CrossRef CAS;
(c) J. G. Lin, S. Q. Zang, Z. F. Tian, Y. Z. Li, Y. Y. Xu, H. Z. Zhu and Q. J. Meng, CrystEngComm, 2007, 9, 915 RSC.
- H. Y. Liu, H. Wu, J. F. Ma, Y. Y. Liu, B. Liu and J. Yang, Cryst. Growth Des., 2010, 10, 4795 CAS.
- H. S. Liu, Y. Q. Lan and S. L. Li, Cryst. Growth Des., 2010, 10, 5221 CAS.
-
(a) L. Zhang, Y. Wei, C. Wang, H. Guo and P. Wang, J. Solid State Chem., 2004, 177, 3433 CrossRef CAS;
(b) J. H. Liao, J. S. Juang and Y. C. Lai, Cryst. Growth Des., 2006, 6, 354 CrossRef CAS;
(c) Y. Xia, P. F. Wu, Y. G. Wei, Y. Wang and H. Y. Guo, Cryst. Growth Des., 2006, 6, 253 CrossRef CAS.
Footnote |
† Electronic supplementary information (ESI) available: X-ray crystallographic files (CIF), selected bond lengths and angles, TG and PXRD patterns, K-M functions vs. energy (eV) plots of the compounds 1–10. CCDC reference numbers 834798–834807. For ESI and crystallographic data in CIF or other electronic format see DOI: 10.1039/c1ce05877k |
|
This journal is © The Royal Society of Chemistry 2012 |