DOI:
10.1039/C1CE05841J
(Paper)
CrystEngComm, 2012,
14, 193-199
From two-dimensional trapezoid-like layer to three-dimensional porous indium-4,4′-biphenyldicarboxylate MOFs†
Received
5th July 2011
, Accepted 13th September 2011
First published on 21st October 2011
Abstract
Three new indium coordination polymers formulated as In(phen)(BPDA)1.5 (1), In(OH)(H2O)(BPDA) (2), and In(BPDA)2·Me2NH2·H2O (3) (phen = 1,10-phenanthroline, C12N2H8; H2BPDA = 4,4′-biphenyldicarboxylic acid, C14O4H10; Me2NH = dimethylamine, C2H7N), have been synthesized under hydro(solvo)thermal conditions and characterized by infrared (IR), thermal gravimetric analysis (TGA) and luminescence studies. In the two-dimensional (2D) trapezoid-like layer of 1, three BPDA2− anions and one phen group bind to a seven-coordinated In3+ ion with the distorted pentagonal bi-pyramid geometry. In 2, the extended –In–OH–In– chains are connected through BPDA2− to form a 3D framework. Complex 3 possesses a two-fold interpenetrating structure, which consists of two independent infinite diamond-like frameworks with point symbol 66. All three samples exhibit varied solid-state fluorescence: emissions at 468 nm (intense blue), 559 nm (greenish-yellow), and 577–645 nm (broad intense orange band with a maximum at 611 nm, λex = 257 nm) for 1, 567 nm (weak greenish-yellow, λex = 344 nm) for 2, and 480 nm (intense greenish-blue, λex = 385 nm) for 3.
Introduction
The design and synthesis of coordination polymers and novel metal–organic framework (MOF) complexes are under extensive investigation in recent years due to their fascinating topological structures and potential applications as functional solid materials.1–3 The divalent transition metal coordination polymers, in particular, have received worldwide attention for their desirable topological structures and properties relevant to the fields of materials science, molecular adsorption, catalysis, and magnetism.4–10 However, compared with the well-developed divalent transition metal coordination polymers, Group 13 metal complexes especially coordination polymers are rarely documented except for the aluminosilicate, gallium-phosphate and phosphite zeolites,11–13 as well as some indium phosphates, phosphites, and aromatic carboxylate complexes.14–21 Because Group 13 metals and divalent transition metals have obvious differences in electronic structures, it presents a great challenge for chemists to design and prepare new Group 13 metal complexes and coordination polymers with fascinating topological structures. Besides, Group 13 metal complexes have great potential as emitting materials for optoelectronic devices, and the most successful example is the discovery of tris(8-hydroxyquinolinolate)aluminum (Alq3) fabricated as organic light-emitting diodes (OLEDs).22,23 By employing appropriate organic ligands as linkers, In3+ metal cations exhibit excellent structural and coordinative flexibility as six-coordinated octahedra MO6, seven-coordinated pentagonal bi-pyramids MO7, and eight-coordinated polyhedra MO8. Some indium complexes even adopt a high coordination number (e.g. 10) configuration.24 The ligand 4,4′-biphenyldicarboxylic acid (H2BDPA) has been employed to design and synthesize transition metal-organic frameworks with various structures that find potential applications as ion-exchange, adsorption, and luminescent materials.25–28 In this work, we report the syntheses, structures, thermal properties and luminescent properties of three new indium coordination polymers formulated as In(phen)(BPDA)1.5 (1), In(OH)(H2O)(BPDA) (2), and In(BPDA)2·Me2NH2·H2O (3) (phen = 1,10-phenanthroline, C12N2H8; H2BPDA = 4,4′-biphenyldicarboxylic acid, C14O4H10; Me2NH = dimethylamine, C2H7N), in which H2BDPA are employed as organic linkers.
Experimental
Materials and instrumentation
All materials and reagents were obtained commercially and used without further purification. IR spectra were recorded on a Nicolet Impact 410 FTIR spectrometer using KBr pellets. Elemental analyses (C, H, and N) were performed on a Perkin-Elmer 240c elemental analyzer. Metal In was determined by a Perkin-Elmer Optima 3300DV ICP spectrometer. TG analyses were carried out on a Diamond TG/DTA instrument (Perkin-Elmer) thermal analyzer (50–950 °C) in air at a scan rate of 10 °C min−1. Luminescence spectra were measured on a Perkin-Elmer LS55 luminescence spectrometer at room temperature.
Preparation of In(phen)(BPDA)1.5 (1)
A mixture of In(NO3)3 (0.0603 g), H2BPDA (0.0482 g), and phen (0.0394 g) with a molar ratio of 2
:
2
:
2 was mixed in H2O (10 mL). After stirring for one hour in air, it was transferred into a 15 mL Teflon-lined stainless steel autoclave and heated at 160 °C for four days. The mixture was washed with distilled water and colorless prism crystals were filtered off and dried at room temperature (yield 54% based on In). The ICP and elemental analyses: Calcd. for C33H20InN2O6 (%): In, 17.55; C, 60.43; H, 3.05; N, 4.27. Found: In, 17.56; C, 60.45; H, 3.07; N, 4.30. IR data (KBr cm−1): 3670(w), 3064(m), 1684(m), 1608(s), 1519(s), 1420(s), 1175(m), 1106(m), 1006(m), 867(s), 799(w), 769(s), 724(s), 689(s), 644(w), 531(w), 450(m).
Preparation of in(OH)(H2O)(BPDA) (2)
A mixture of In(NO3)3 (0.0608 g), H2BPDA (0.0969 g), and phen (0.0199 g) with a molar ratio of 2
:
4
:
1 was mixed in H2O (10 mL). After stirring for one hour in air, it was transferred into a 15 mL Teflon-lined stainless steel autoclave and heated at 160 °C for three days. The mixture was washed with distilled water and colorless hexagonal block crystals were filtered off and dried at room temperature (yield 48% based on In). The ICP and elemental analyses: Calcd. for C14H11InO6 (%): In, 29.48; C, 43.07; H, 2.82. Found: In, 29.49; C, 43.10; H, 2.85. IR data (KBr cm−1): 3431(m), 1681(s), 1607(s), 1579(m), 1517(w), 1426(s), 1295(s), 1180(w), 1130(w), 1007(w), 932(w), 847(m), 804(w), 759(s), 691(w), 669(w), 556(w), 448(w).
Preparation of in(BPDA)2·Me2NH2·H2O (3)
A mixture of In(NO3)3 (0.0609 g), H2BPDA (0.0968 g), and phen (0.0199 g) with a molar ratio of 2
:
4
:
1 was mixed in H2O (5 mL) and N,N-dimethylformamide (DMF) (5 mL). After stirring for half an hour in air, it was transferred into a 15 mL Teflon-lined stainless steel autoclave and heated at 160 °C for three days. The mixture was washed with distilled water and colorless octahedral block crystals were filtered off and dried at room temperature (yield 51% based on In). The ICP and elemental analyses: Calcd. for C30H26InNO9 (%): In, 17.41; C, 54.60; N, 2.12; H, 3.94. Found: In, 17.40; C, 54.63; N, 2.14; H, 3.92. IR data (KBr cm−1): 3420(m), 3066(m), 1660(m), 1607(s), 1538(m), 1404(s), 1176(m), 1105(w), 1005(m), 872(s), 770(s), 691(m), 525(w), 444(m).
Crystallographic data of complexes 1–3 were collected on a Bruker Apex II CCD diffractometer using graphite-monochromated Mo-Kα radiation (λ = 0.71073 Å) at 293 ± 2 K. Data processing was accomplished with the SAINT processing program.29 The structures were solved by direct method and refined by full-matrix, least squares based on F2 using the SHELXTL 5.1 software package.30 The indium atoms were first located, and then the oxygen, carbon, and nitrogen atoms. The hydrogen atoms of the organic amine molecules were refined in calculated positions. All non-hydrogen atoms were refined anisotropically. Crystal data and details of data collection and refinement are given in Table 1. CCDC reference numbers: 832523 for 1, 832524 for 2, and 832525 for 3.†
Table 1
Crystal data and structure refinement for complexes 1–3
Complexes |
1
|
2
|
3
|
R
1 = ∑‖Fo| − |Fc‖/∑|Fo|, wR2 = [∑[w(Fo2 − Fc2)2]/∑[w(Fo2)2]]1/2.
|
Empirical formula |
C33H20InN2O6 |
C14H11InO6 |
C30H26InNO9 |
Fw
|
655.33 |
390.05 |
659.34 |
T/K |
294(2) |
294(2) |
294(2) |
λ/Å |
0.71073 |
0.71073 |
0.71073 |
Crystal system |
Monoclinic |
Orthorhombic |
Orthorhombic |
Space group |
P21/c |
Pnma
|
Fddd
|
a/Å |
11.805(2) |
5.9716(12) |
23.129(5) |
b/Å |
24.903(5) |
28.516(6) |
39.470(8) |
c/Å |
9.881(2) |
7.5076(15) |
40.610(8) |
α (°) |
90 |
90 |
90 |
β (°) |
103.12(3) |
90 |
90 |
γ (°) |
90 |
90 |
90 |
V/Å3 |
2828.8(10) |
1278.4(4) |
37073(13) |
Z
|
4 |
4 |
32 |
μ/mm−1 |
0.885 |
1.875 |
0.544 |
θ range (°) |
3.03–27.46 |
3.46–27.48 |
3.11–25.00 |
R [I > 2σ(I)]a |
R
1 = 0.0405 |
R
1 = 0.0366 |
R
1 = 0.0696 |
|
wR2 = 0.0783 |
wR2 = 0.1212 |
wR2 = 0.1404 |
Results and discussion
Synthesis and characterization
Complexes 1, 2, and 3 have been successfully synthesized under hydro(solvo)thermal conditions. Complexes 1 and 2 were prepared using the same reactants but with different molar ratios and reactions. This suggests that the molar ratio is a critical factor in determining the final products. In addition, reaction time plays an important role in the growth of crystals. If the reaction time is too short, crystals are usually too small; if the reaction time is too long, amorphous powder is obtained instead of crystals. The synthetic method of complex 3 is similar to that of complex 2, the difference is that the solvent is changed from H2O to a mixture of H2O and DMF. In different solvents, the solubility of the synthesized mixture is different, and the result is that the crystal structure of complex 3 is completely different to that of 2. Though phen was not incorporated in the final products, complexes 2 and 3 cannot be obtained in a series of control experiments without using phen, indicating that phen plays important roles in the reaction process of complexes 2 and 3. The three complexes are all stable in the solid state in open air. Complexes 1, 2, and 3 were characterized by IR spectroscopy, elemental analyses and single-crystal X-ray diffraction analyses. The three complexes are not soluble in common solvents such as CH2Cl2, CH3CN, DMF, or DMSO, and as a result, their 1H NMR, UV-Vis and fluorescent spectroscopy in solutions could not be obtained.
The elemental analysis and ICP analysis results reveal that the components of the three complexes are in agreement with their formula. The IR spectra of the free ligand 4,4′-biphenyldicarboxylic acid (H2BPDA) shows the characteristic band of the carboxyl group at 1705 cm−1. When coordinated with In3+ metal cations, the absorption peak splits into two: 1608 and 1420 cm−1 for 1, 1607 and 1426 cm−1 for 2, and 1607 and 1404 cm−1 for 3. These results correspond to asymmetric and symmetric stretching vibrations of the carboxyl groups, respectively. In the above three complexes, the stretching bands of the carboxyl groups shift toward lower frequencies and their intensity is significantly reduced. These phenomena confirm the coordinative interaction between the carboxyl O atoms and In3+ metal cations.
Structural descriptions
Structure of In(phen)(BPDA)1.5 (1).
Single crystal X-ray diffraction analysis reveals that complex 1 crystallizes in a space groupP21/c. The central In3+ cation is coordinated by three BPDA2− anions and one phen molecule, as shown in Fig. 1. Each In3+ cation is seven-coordinated with five carboxyl oxygen atoms (O(1), O(2), O(3), O(4), O(6B)) of three BPDA2− anions and two nitrogen atoms (N(1), N(2)) from one phen molecule, forming a distorted InO5N2 pentagonal bi-pyramid. In the molecular structure of 1, the BPDA2− ligands have two different coordination modes. The first type of BPDA2− is coordinated to two In3+ cations through three carboxyl oxygen atoms (O(1), O(2), O(6)), in which one carboxyl arm adopts a bi-dentate coordination mode and the other adopts a mono-dentate mode. The second type of BPDA2− links two In3+ cations through four carboxyl oxygen atoms, in which the two carboxyl arms both adopt a bi-dentate coordination mode. In the first coordination mode, the bond distances of In–OCOO– vary from 2.142(3) to 2.358(2) Å, of which the bond distance of In(1)–O(6) is the shortest. The bond distances of C–OCOO– of the bi-dentate coordinated carboxyl arms are 1.250(4) Å for C(13)–O(1) and 1.279(4) Å for C(13)–O(2). In the mono-dentate coordinated carboxyl arm, the C(26)–O(6) bond distance is 1.269(5) Å; it is coordinated to the central In3+ cation. The remaining C(26)–O(5) bond distance is slightly shorter with a value of 1.250(5) Å, and it is not coordinated to the central In3+ cation. In the second coordination mode, the bond distances of In–OCOO– are between 2.208(2) and 2.383(2) Å. The C–OCOO– bond distances are between 1.253(4) and 1.272(4) Å. The other phen molecule is coordinated to the central In3+ cation through two N atoms, with the bond distances of In(1)–N(1) = 2.384(3) Å, In(1)–N(2) = 2.332(3) Å, and the bond angle of N(1)–In(1)–N(2) = 69.86(10)°. Moreover, the bond angle of the mono-dentate carboxyl arm is 121.1(4)°, which is slightly larger than that of the bi-dentate carboxyl arm (about 119.6°). As shown in Fig. 2, the adjacent In3+ cations are connected through BPDA2− ligands to form an infinite chain, in which the BPDA2− ligands adopt the first coordination mode. The distance between the two In3+ centers is about 15.25 Å. The dihedral angle between the two benzene planes of the BPDA2− ligand is about 27.35°. Besides, the neighboring chains are further linked through BPDA2− ligands to form a 2D layered structure, in which the carboxyl ligands adopt the second coordination mode. In the 2D layered structure of complex 1, each In3+ cation is coordinated to three BPDA2− ligands, and each BPDA2− ligand links two In3+ cations. In this way, six In3+ centers and six BPDA2− ligands connect to each other through carboxyl groups, forming a 12-membered grid with dimensions of about 23.7 Å × 33.2 Å (atom-to-atom distances, as shown in Fig. S1†). Fig. 3 shows the trapezoid-like (6,3) layer of complex 1, in which the In3+ centers are treated as 3-connected nodes. There exist aromatic π–π packing interactions between the neighboring layers in the structure of complex 1. The shortest distance between the adjacent benzene rings is about 3.39 Å, as shown in Fig. 4.
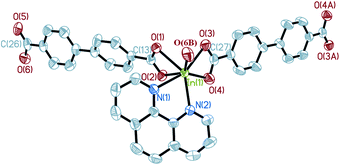 |
| Fig. 1 The coordination environment around In3+ in complex 1 (50% thermal ellipsoids). Hydrogen atoms on the ligands are omitted for clarity. Symmetry codes: A: −x, −y, −z; B: x + 1, −y + 1/2, z − 1/2. | |
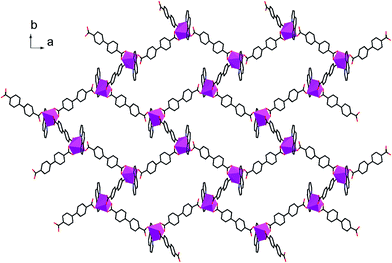 |
| Fig. 2 The 2D layered structure of complex 1 along the c axis. | |
![A view of the (6,3) network of complex 1 along [100] (a), [010] (b), and [001] (c) directions.](/image/article/2012/CE/c1ce05841j/c1ce05841j-f3.gif) |
| Fig. 3 A view of the (6,3) network of complex 1 along [100] (a), [010] (b), and [001] (c) directions. | |
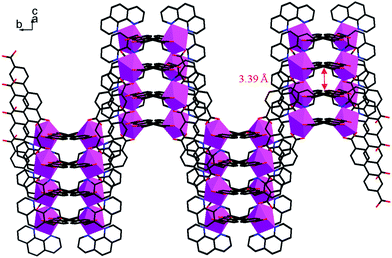 |
| Fig. 4 A view of the 2D trapezoid-like structure of complex 1 on the bc plane. | |
Structure of In(OH)(H2O)(BPDA) (2).
Single crystal X-ray diffraction analysis reveals that complex 2 crystallizes in the space groupPnma. As shown in Fig. 5, the molecular structure of 2 is composed of one In3+ cation, two BPDA2− anions, two hydroxyl oxygen atoms, and one coordinated water molecule. Each central In3+ is seven-coordinated by four carboxyl oxygen atoms (O(1), O(2), O(1A), O(2A)) from two BPDA2− anions, two hydroxyl oxygen atoms (O(3), O(3D)), and one coordinated water molecule (O(4)), forming a slightly distorted In(OH)2(H2O)O4 pentagonal bi-pyramid geometry. The bond distances of In(1)–OCOO– are between 2.259(4) and 2.362(4) Å. Compared with the In(1)–OCOO– bond distances, the bond distances of the central In3+ cation and hydroxyl O atoms and coordinated water molecule are much shorter, which vary from 2.068(5) to 2.180(5) Å. The C–OCOO– bond distances of the bi-dentate coordinated carboxyl arms are 1.250(6) Å for C(1)–O(1) and 1.269(6) Å for C(1)–O(2). The bond angles of the bi-dentate coordinated carboxyl arm O(1)–C(1)–O(2) is 119.7(4)°, with the O(1)–In(1)–O(2) bond angle of 56.22(13)°. The bond angle of the vertexes and central In3+ O(3)–In(1)–O(4) is 171.3(2)°, which is close to 180°. As shown in Fig. 6a, every four BPDA2− ligands and two hydroxyl groups link six In3+ cations to generate a In6 ring with dimensions of about 5.88 × 28.78 Å (atom-to-atom distances). As shown in Fig. 6b, every two BPDA2− ligands and four hydroxyl groups join six In3+ cations to form another In6 ring (different from the above one) with dimensions of about 5.97 × 18.47 Å (atom-to-atom distances). On the one hand, the adjacent In3+ centers are connected through BPDA2− anions to form a wavelike –In–BPDA–In–1D chain along the b axis, in which the shortest In–In distance is about 15.41 Å. On the other hand, the neighboring In3+ centers are linked by hydroxyl groups to form another sawtooth –In–OH–In–1D chain along the a axis. The neighboring parallel –In–BPDA–In– chains are joined through –In–OH–In– chains to form a 2D double-layer, which is further connected through –In–OH–In– chains to construct the 3D open-framework with 10-membered ring channels of complex 2 along the a axis (Fig. 7a). Molecular structure analysis reveals that complex 2 possesses 4-connected topological structure (Fig. 7b). Moreover, the In(1)–O(4) vectors point toward the 10-membered ring channels and form strong hydrogen bonds with the adjacent carboxyl O atoms (O(4)–H(4A)…O(2), 2.678(2) Å, x − 1/2, y, −z − 1/2). Moreover, the coordinated water molecules form weak hydrogen bonds with the adjacent hydroxyl O atoms (O(3)–H(3A)…O(2), 3.088(2) Å, x − 1/2, y, −z + 1/2; O(3)–H(3A)…O(2), 3.088(2) Å, x − 1/2, −y + 3/2, −z + 1/2).
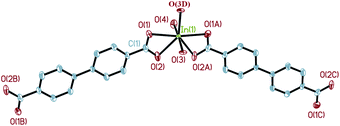 |
| Fig. 5 The coordination environment around In3+ in complex 2 (50% thermal ellipsoids). Hydrogen atoms are omitted for clarity. Symmetry codes: A: x, −y + 3/2, z; B: −x + 1, −y + 1, −z; C: −x + 1, y + 1/2, −z; D: x − 1/2, −y + 3/2, −z + 1/2. | |
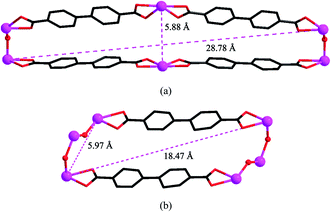 |
| Fig. 6 Views of the two types of In6 ring in complex 2. | |
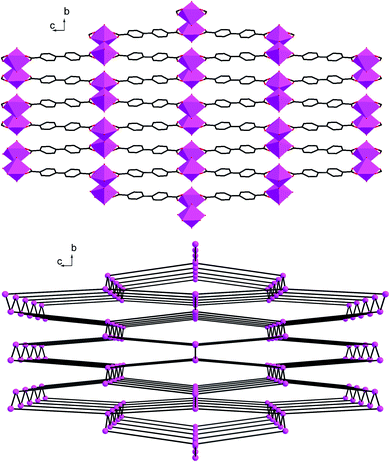 |
| Fig. 7 (a) A polyhedral view of the 3D open-framework of complex 2 along a axis; (b) The 4-connected topological structure of complex 2. | |
Structure of In(BPDA)2·Me2NH2·H2O (3).
Single crystal X-ray diffraction analysis reveals that complex 3 crystallizes in the space groupFddd. The asymmetric unit of complex 3 contains two crystallographically independent In3+ cations (In(1) and In(2)), but they have the same chemical environments. As shown in Fig. 8, the central In(1)3+ cation is coordinated by eight carboxyl oxygen atoms (O(5), O(6), O(5A), O(6A), O(7B), O(8B), O(7C), O(8C)) of four BPDA2− anions, forming a slightly distorted InO8 polyhedron geometry. The bond distances of In(1)–OCOO– are between 2.234(3) and 2.321(3) Å. Compared with the In(1)–OCOO– bond distances, the bond distances of the In(2)–OCOO– are varying from 2.190(3) to 2.406(3) Å. The C–OCOO– bond distances of the bi-dentate coordinated carboxyl arms are between 1.200(6) and 1.286(5) Å. As shown in Fig. 9a, each In(1)3+ center joins the neighboring four In(1)3+ cations through four bridging ligands to form a tetrahedron geometry, and each BDPA2− ligand links the adjacent two In(1)3+ centers through two carboxyl groups in bi-dentate chelating modes. The adjacent In(1)3+ centers are connected through the bridging BDPA2− anions to form an infinite diamond-like framework with 66 point symbol. In the same way, another infinite diamond-like framework is constructed from In(2)3+ cations and bridging organic ligands (Fig. 9b). Notably, though the chemical environments of the central In(1)3+ and In(2)3+ cations are the same, the major difference is the dihedral angle between the planes of the two benzene rings in one bridging organic ligand. The dihedral angle between the two benzene ring planes in one briding ligand is 5.27(27)° in the In(1) network, which is much smaller than that of 27.54(19)° in the In(2) network. Due to the strong distortion, the unique two-fold interpenetrating structure of complex 3 is generated, in which the metal centers provide the tetrahedral nodes, and the BDPA2− ligands act as linear bridges between the metal centers. Every tetrahedral node is located at the center of an adamantane unit of the other framework, and the four connections to the nearest neighbor project through the four surrounding adamantine windows. Therefore, the two independent networks are interpenetrated, as shown in Fig. 9c. Moreover, protonated dimethylamine molecules are employed as charge compensation components, which are formed by the decomposition of the DMF molecules. The protonated dimethylamine and crystallizing water molecules are located in the channels of the network. As far as we know, the well-known interpenetrated structure has only been found in transition metal MOFs31–34 and has not been reported in Group 13 metal frameworks.
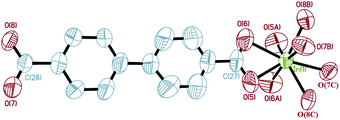 |
| Fig. 8 The coordination environment around In(1)3+ in complex 3 (50% thermal ellipsoids). Hydrogen atoms on the ligands are omitted for clarity. Symmetry codes: A: −x + 1/4, y, −z + 1/4; B: x − 1/4, y − 1/4, −z; C: −x + 1/2, y − 1/4, z + 1/4. | |
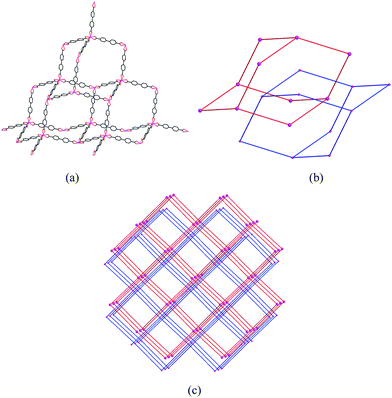 |
| Fig. 9 A view of the diamond-like framework (a); two independent diamond-like frameworks (b); and the two-fold interpenetrating structure (c) of complex 3. | |
Thermal properties
Thermal gravimetric analyses (TGA) were carried out on complexes 1–3 to investigate their thermal stabilities (Fig. S2†). Complex 1 is thermally stable until around 400 °C. In the temperature range of 400–550 °C, a weight loss of about 79.50% is observed, which corresponds to the removal of phen and H2BPDA (Calcd. 79.82%). The TGA curve of complex 2 shows the first weight loss of 1.83% up to 320 °C, which corresponds to the loss of part of the coordinated water molecules. The second weight loss of 18.16% occurs at around 320–435 °C, owing to the loss of coordinated water molecules and part of the H2BPDA. Finally, a sharp weight loss of 45.50% is observed due to the removal of the rest of the H2BPDA between 435 and 510 °C. Complex 3 decomposes gradually from 50 to 325 °C with a weight loss of 21.56%. After the removal of crystallizing water, dimethylamine, and part of H2BPDA, the framework collapsed sharply at 325–950 °C due to the loss of the rest of the H2BPDA, with a weight loss of 57.23%. Because the TG analyses were carried out in air, the three complexes finally transformed to the final residual of the In2O3 phase after the release of water and organic ligands (Calcd. 21.18% for 1, 35.58% for 2, and 21.05% for 3), which are in agreement with the experimental results (Found: 20.50% for 1, 34.51% for 2, and 21.21% for 3).
Luminescence properties
The luminescence properties of complexes 1, 2, and 3 are investigated in the solid state at room temperature (as shown in Fig. 10). Complex 1 displays intense blue emission at 468 nm, greenish-yellow emission at 559 nm, and an intense orange emission band (577–645 nm) maximum at 611 nm (λex = 257 nm). Complex 2 exhibits an intense fluorescent emission at 414 nm and a weak greenish-yellow emission band at around 567 nm (λex = 344 nm). An intense fluorescent emission band (395–406 nm) and an intense greenish-blue emission at 480 nm of complex 3 are observed (λex = 385 nm). Free H2BPDA ligand is known to exhibit a maximum emission at around 397 nm (λex = 236 nm).35 In comparison, the emission frequencies of the three complexes are obviously red-shifted. Among them, the biggest red-shift of complex 1 is observed, of which the luminescent band extends from blue to orange emission. The red-shift of emission energy is likely to be caused by the intermolecular interaction in the solid state that effectively decreases the energy gap. The fluorescent emission of the three complexes may be assigned to the charge transition of the ligand-to-metal In(III) center (LMCT).2,16,17,19
Conclusion
In conclusion, three new main group In3+ coordination polymers have been hydro(solvo)thermally synthesized. In the 2D layer of complex 1, phen groups hinder the further connection of central metals and H2BPDA ligands. Through adjusting the molar ratios of the metal sources and organic ligands and using different solvents, more open 3D In3+ coordination polymers of 2 and 3 are successfully prepared. TGA reveals that they are materials with good thermal stability. Compared with 2 and 3, complex 1 displays a broader luminescent band extending from the blue to orange region. The main reason is that the nice coplanar feature of the 2D layer structure in 1 can enhance the mobility of π electrons in organic aromatic rings, which are in favor of fluorescent emission. The good luminescent properties in the solid state reveal that they are a new class of luminescent main group metal materials with potential applications in optoelectronic devices. Further work to investigate the structural features and luminescent properties of main group metal MOFs related to organic carboxylic acids is in progress.
Acknowledgements
This work was supported by the National Science Foundation of China (Grant No. 20971031, 21071035 and 21171044), the China Postdoctoral Science Foundation Funded Project (No. 65204), and the key Natural Science Foundation of the Heilongjiang Provence, China (No. ZD201009).
References
- D. J. Tranchemontagne, L. J. Mendoza-Cortés, M. O'Keeffe and O. M. Yaghi, Chem. Soc. Rev., 2009, 38, 1257 RSC.
- Q. Gao, F. Jiang, M. Wu, Y. Huang, D. Yuan, W. Wei and M. Hong, CrystEngComm, 2009, 11, 918 RSC.
- F. Nouar, J. Eckert, J. F. Eubank, P. Forster and M. Eddaoudi, J. Am. Chem. Soc., 2009, 131, 2864 CrossRef CAS.
- R. J. Kuppler, D. J. Timmons, Q. Fang, J. Li, T. A. Makal, M. D. Young, D. Yuan, D. Zhao, W. Zhaung and H. Zhou, Chem. Soc. Rev., 2009, 253, 3042 CAS.
- J. R. Li, Q. Yu, Y. Tao, X. H. Bu, J. Ribas and S. R. Batten, Chem. Commun., 2007, 2290 RSC.
- L. Wang, W. You, W. Huang, C. Wang and X. You, Inorg. Chem., 2009, 48, 4295 CrossRef CAS.
- C. A. Bauer, T. V. Timofeeva, T. B. Settersten, B. D. Patterson, V. H. Liu, B. A. Simmons and M. D. Allendorf, J. Am. Chem. Soc., 2007, 129, 7136 CrossRef CAS.
- B. Ye, M. Tong and X. Chen, Coord. Chem. Rev., 2005, 249, 545 CrossRef CAS.
- J. L. C. Rowsell and O. M. Yaghi, Microporous Mesoporous Mater., 2004, 73, 3 CrossRef CAS.
- M. D. Allendorf, C. A. Bauer, R. K. Bhakta and R. J. T. Houk, Chem. Soc. Rev., 2009, 38, 1330 RSC.
- A. K. Cheetham, G. Férey and T. Loiseau, Angew. Chem., Int. Ed., 1999, 38, 3268 CrossRef CAS.
- L. Huang, T. Song, L. Zhang, Y. Chen, J. Jiang, J. Xu and L. Wang, CrystEngComm, 2010, 12, 2198 RSC.
- K. Lii and Y. Huang, Inorg. Chem., 1999, 38, 1348 CrossRef CAS.
- C. Volkringer, M. Meddouri, T. Loiseau, N. Guillou, J. Marrot, G. Férey, M. Haouas, F. Taulelle, N. Audebrand and M. Latroche, Inorg. Chem., 2008, 47, 11892 CrossRef CAS.
- M. Vougo-Zanda, J. Huang, E. Anokhina, X. Wang and A. J. Jacobson, Inorg. Chem., 2008, 47, 11535 CrossRef CAS.
- Z. Zhong, F. Jiang, L. Chen, C. Yue, D. Yuan, A. Lan and M. Hong, Cryst. Growth Des., 2007, 7, 1712 Search PubMed.
- Y. Liu, V. Ch. Kravtsov, R. Larsen and M. Eddaoudi, Chem. Commun., 2006, 1488 RSC.
- Y. Liu, J. F. Eubank, A. J. Cairns, J. Eckert, V. Ch. Kravtsov, R. Luebke and M. Eddaoudi, Angew. Chem., Int. Ed., 2007, 46, 3278 CrossRef CAS.
- Z. Han, Y. He, M. Tong, Y. Song, X. Song and L. Yang, CrystEngComm, 2008, 10, 1070 RSC.
- G. S. Papaefstathiou, A. Manessi, C. P. Raptopoulou, A. Terzis and T. F. Zafiropoulos, Inorg. Chem., 2006, 45, 8823 CrossRef CAS.
- S. Zheng, F. Zuo, T. Wu, B. Irfanoglu, C. Chou, R. A. Nieto, P. Feng and X. Bu, Angew. Chem., Int. Ed., 2011, 50, 1849 CrossRef CAS.
- C. Tang and S. A. Van Slyke, Appl. Phys. Lett., 1987, 51, 913 CrossRef CAS.
- C. Chen and J. M. Shi, Coord. Chem. Rev., 1998, 171, 161 CrossRef CAS.
- B. F. T. Cooper and C. L. B. Macdonald, J. Organomet. Chem., 2008, 693, 1707 CrossRef CAS.
- N. L. Rosi, M. Eddaoudi, J. Kim, M. O'Keeffe and O. M. Yaghi, Angew. Chem., Int. Ed., 2002, 41, 284 CrossRef CAS.
- A. C. Sudik, A. P. Côté, A. G. Wong-Foy, M. O'Keeffe and O. M. Yaghi, Angew. Chem., Int. Ed., 2006, 45, 2528 CrossRef CAS.
- Y. Liu, Y. Qi, Y. Lv, Y. Che and J. Zheng, Cryst. Growth Des., 2009, 9, 4797 CAS.
- X. Shi, G. Zhu, X. Wang, G. Li, Q. Fang, G. Wu, G. Tian, M. Xue, X. Zhao, R. Wang and S. Qiu, Cryst. Growth Des., 2005, 5, 207 CAS.
-
SMART and SAINT software package; Siemens Analytical X-ray Instruments Inc.: Madison, WI, 1996 Search PubMed.
-
G. M. Sheldrick, SHELXL Program, version 5.1; Siemens Industrial Automation, Inc.: Madison, WI, 1997 Search PubMed.
- B. F. Hoskins and R. Robson, J. Am. Chem. Soc., 1990, 112, 1546 CrossRef CAS.
- T. Kitazawa, S. Nishikiori, R. Kuroda and T. Iwamoto, J. Chem. Soc., Dalton Trans., 1994, 1029 RSC.
- S. R. Batten and R. Robson, Angew. Chem., Int. Ed., 1998, 37, 1460 CrossRef.
- S. R. Batten, CrystEngComm, 2001, 18, 1 Search PubMed.
- X. Guo, G. Zhu, Q. Fang, M. Xue, G. Tian, J. Sun, X. Li and S. Qiu, Inorg. Chem., 2005, 44, 3850 CrossRef CAS.
|
This journal is © The Royal Society of Chemistry 2012 |