DOI:
10.1039/C1SC00162K
(Minireview)
Chem. Sci., 2011,
2, 2301-2305
Molecular recognition of cytochrome c by designed receptors for generation of in vivo and in vitro functions
Received
19th March 2011
, Accepted 27th June 2011
First published on 21st July 2011
Abstract
Cytochrome c is a common guest in biological protein recognition processes and works not as an enzyme, but as an electron carrier in biological respiration. Although this is a relatively small protein, its structure is too complicated to be easily recognized by common synthetic receptors. This review is an overview of the molecular recognition of cytochrome c by synthetic receptors and highlights two examples exhibiting in vivo and in vitro non-biological functions: (i) crown ether receptors effectively interact with cationic residues via multiple crown ether complexations and (ii) dendrimer receptors strongly bind with a negatively charged patch via complementary electrostatic interactions. These designed receptors offer effective cytochrome c recognition to generate non-biological catalytic activity and in cell functions.
1. Introduction
In addition to genetic and other biological methods, many chemical efforts have been devoted toward the understanding of biological protein recognition and the generation of sophisticated functions. The tuning of metal center structures, reorganization of substrate recognition modes and addition of uncommon environmental domains have been successfully applied in metalloprotein systems.1 Several synthetic receptors have also been developed to modify the structures and functions of metalloproteinsvia molecular recognition, which has provided a useful basis for the design of metalloprotein-based supramolecules and applications in pharmacology, biosensing and nano-biomaterials chemistry.2
Fig. 1 illustrates representative examples of protein guests, which include histone, cytochrome c, lysozyme, trypsin, proteinase K, albumin and ferritin proteins.3 Since each protein has a mosaic-like charge distribution on its nanoscale surface, many kinds of synthetic receptors developed in common molecular recognition chemistry have many difficulties in recognizing these proteins.4,5 We focus here on synthetic receptors offering the recognition of cytochrome c because its structure and folding have been extensively characterized experimentally and computationally.6 Several excellent receptors, described below, have been demonstrated to form supramolecular complexes with cytochrome c and two further examples are detailed that generate non-biological structures and functions via multiple non-covalent interactions. Although only cytochrome c is targeted as a guest protein in this review, various designed receptors have been recently reported to provide precise recognition of other proteins.7 Since some of them perturbed and inhibited biological protein–protein interactions, the development of designed receptors offers enormous challenges in chemical, biological and medical research.8
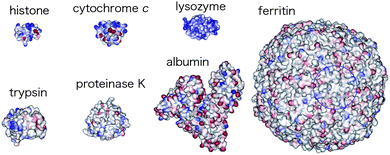 |
| Fig. 1 Representative examples of protein guests. Reprinted with permission from ref. 3. Copyright 2006, American Chemical Society. | |
Cytochrome c is the most studied guest in biological protein recognition processes and works not as an enzyme, but as an electron carrier in biological respiration. Horse heart cytochrome c has two coordinative residues, His 18 and Met 80 (horse heart cyt c), that form a six-coordinate low-spin heme complex, whereas most catalytically active heme enzymes have highly reactive five-coordinate hemes with open sites.6 Although this is a relatively small protein with a positively charged surface, its recognition requires multiple interactions with the designed receptor. As schematically shown in Fig. 2, effective synthetic receptors provide three classes of non-covalent interactions with cytochrome c: (1) point interactions with specific cationic residues of the protein; (2) patch interactions with a lysine cluster located near the entrance of the heme crevasse; (3) environmental (non-stoichiometric) interactions, such as solvation and ion-pairing interactions with polycations. When the receptor interacts with cationic residues of the protein in a “point interaction” fashion, the charge density on the protein surface decreases to convert the heme coordination structure from biological to non-biological forms. In nature, cytochrome c forms a stable 1
:
1 supramolecular complex with cytochrome b5, in which two porphyrinate centers are well arranged in the proper geometry for efficient electron transfer.9 Since cytochrome c has a positively charged patch composed of four protonated lysine residues, and because cytochrome b5 has several carboxylate anions on the surface, the two unsymmetrically distributed patches effectively dock to offer complementary electrostatic interactions in a “patch interaction” fashion. “Environmental interactions”, such as solvation and non-stoichiometric binding, also induce protein denaturation, in which both local and global structures are dynamically perturbed.
Representative examples of reported cytochrome c receptors are summarized in Fig. 3. They significantly involve “point interactions”, “patch interactions” and “environmental interactions”, although two or more interaction modes participate in some systems. Although cytochrome c has a large surface, Kunitake et al. demonstrated that membrane aggregates of anionic surfactant1 had polycationic interfaces suitable for domain binding of cytochrome cvia electrostatic interactions to generate peroxidase activity.10 Hamachi et al. presented Ru-complex 2 with polyanionic bipyridineligands as dendrimer receptors and found that photoreduction efficiency of the complexed cytochrome c largely depended on the number of carboxylate groups.11 Hamilton et al. reported metalloporphyrin derivative 3 with several –CO2−groups that matched the positively charged patch of cytochrome c.12 They further demonstrated that the metalloporphyrin dimer induced the unfolding of cytochrome c and accelerated proteolytic degradation. Calixarene4 with polyanionic peptides was also developed as an effective receptor for patch interaction with cytochrome c.13Ogawaet al. employed polyanionic oligo(glutamic acids) 5 in cytochrome c binding,14 while Hirota et al. used oligo(aspartic acids) 6.15 The latter interacted with cytochrome c in the same way as plastocyanin. Their resonance Raman spectroscopic and electrochemical measurements showed an enhancement of the electron donor ability of cytochrome c upon complexation with polyanionic receptor 6. Hirsch et al. prepared fullerene-derived polyanionic dendrimer 7 to offer nanoscale complexation with cytochrome c.16 Although a family of water soluble fullerene derivatives exhibited a high reactivity against toxic reactive oxygen species and anti-HIV activity, dendrimer 7 showed pronounced neuroprotective properties, both in vitro and in vivo. Goto et al., Ohno et al. and others employed ionic liquids in the solubilization of cytochrome c.17 When cytochrome c was typically dissolved in ethylmethylimidazolium ethylsulfate, its secondary structure was unchanged, but the tertiary structure was disturbed. Several kinds of nanoparticles have recently been developed for recognition and sensing of cytochrome c.18 When nanoparticles were typically modified by amino acids to bind cytochrome c, their recognition profiles largely depended on the nature of the introduced amino acids. The monolayer-coated nanoparticles were also found to provide effective platforms for recognition of protein surfaces.19 Further combinations of the designed receptors described above with nanoparticles can offer precise recognition and sensing of cytochrome c.20
The development of these designed receptors provides a better understanding of how to effectively manage protein recognition processes and engineer more sophisticated functional systems. Since some cytochrome c–receptor complexes have exhibited characteristic electron transfer abilities in electrochemical processes, selective receptors have many applications in sensing, imaging, and other research. Indeed, fluorescent Ru complexes and porphyrinates 2, 3, and 5 were applicable in analytical processes. In addition to their role as a key participant in biological electron transfer,6cytochrome c works as a trigger of programmed cell death.21 Excess apoptosis accounts for the pathogenesis of various human disorders, such as hepatitis, spinal cord injury and Parkinson's disease. The designed receptors of cytochrome c can be promising candidates for new nanomedicines inhibiting apoptosis, if their structures are optimized to operate well, even in living cells.
(a) Multiple crown ether complexation in organic media
Several crown ethers bind –NH3+, –CO2−M+ or other ionic residues exposed on protein surfaces and form n
:
1 (crown ether
:
protein) types of supramolecular complexes. Odell and Earlam previously reported that common crown ethers solubilize water-soluble cytochrome c into organic solvents.22 Based on ESI-MS studies, Julian and Beauchamp found that four 18-crown-6 molecules strongly bound cytochrome c.23 The resulting cytochrome c–crown ether complexes have particularly interesting features as asymmetric catalysts working in organic media: (1) the biologically inactive heme structure was converted to a catalytically active form; (2) the protein matrix provided an asymmetric environment around the activated heme center; (3) the high solubility in organic media allowed low temperature reaction to suppress the oxidative degradation of heme and enhance the enantiomer selectivity; (4) a different variety of substrates and conditions were available from those in aqueous systems; (5) no laborious procedures, such as derivatization, purification, dialysis and lyophilization, were required. Although this oxidizes phospholipid “cardiolipin” via 1
:
1 complexation in nature,24 we demonstrated that the cytochrome c–crown ether complex effectively promoted the asymmetric oxidation of sulfoxides in methanol at −40 °C.25
Lariat ethers, possessing an 18-crown-6 ring, form supramolecular complexes with horse heart, pigeon breast and yeast cytochrome cproteins in methanol (Fig. 4). Although the employed cytochrome c is insoluble in methanol, its supramolecular complexes gave homogeneous solutions and rarely precipitated, even when at −75 °C after several days. Polyethylene-glycolated cytochrome c was soluble in several organic media, but formed an insoluble gel material in methanol at <0 °C. The LMCT bands due to Met 80 (horse heart cyt c) coordination disappeared in the electronic spectra around 695 nm, indicating that the coordinative methionine residue was displaced from the sixth coordination position of the heme. The resonance Raman spectra exhibited the characteristic bands of a six-coordinate low-spin iron(III) complex at 1376, 1508, 1590 and 1641 cm−1, suggesting that lysine or another neighboring amino acid occupied the sixth coordination position. The enhanced circular dichroism bands, observed at around 209 and 420 nm, further confirmed that the artificially activated heme was located in the asymmetrically structured protein.
(b) Asymmetric catalysis at low temperature
Cytochrome c is known to display minor peroxidase activity in aqueous solution, but the degradation of its heme center with H2O2 has frequently occurred.6 The supramolecular complexes of cytochrome c with lariat ethers exhibited higher reactivity toward H2O2 degradation because of their artificially activated heme structures.26 Typically, the lifetime of a horse heart cytochrome c–lariat ether complex was estimated to be 0.3 min in methanol and 20 min in water at 20 °C, but the degradation was greatly decreased by lowering the temperature in methanol: lifetime = 150 min (−40 °C) and >2500 min (−60 °C). Such a cytochrome c–lariat ether complex catalyzed the oxidation of several sulfoxides, sulfides and anthracene in methanol at low temperature, although styrene, ethyl benzene and benzyl alcohol rarely reacted. When racemic naphthyl methyl sulfoxide was oxidized in the presence of a cytochrome c–lariat ether complex with H2O2, (S)-naphthyl methyl sulfoxide was more rapidly oxidized than the (R)-isomer (Fig. 4). The horse heart cytochrome c complex exhibited the highest conversion (73%) and enantiomer selectivity (e.e.% = 47%) at −40 °C after 15 h. Methyl tolyl sulfoxide, iso-propyl phenyl sulfoxide, benzyl methyl sulfoxide and 4-methylsulfenyl acetophenone were also oxidized in an (S)-selective fashion: conversion after 15 h, 15–93%; e.e.% at 80% conversion, 8–47%. Pigeon breast cytochrome c complex exhibited comparable activities in oxidation of these sulfoxides to those of horse heart cytochrome c, while the yeast cytochrome c complex modestly promoted sulfoxideoxidation. Although the active intermediate could not be detected in this catalytic reaction, the oxygen atom on the reactive heme was directly added to the sulfoxide substrate as proposed in microperoxidase-catalyzed oxidation. In biological heme enzymes, protein compositions, external circumstances and other factors are finely tuned to give specific catalytic functions. The lariat ethers worked well as an artificial stimulus for activating cytochrome c and attained non-biological catalysis.
4.
Dendrimer receptors for cytochrome c recognition
(a) Nanoscale protein complexation in test tubes
A variety of dendrimers were developed as nanoscale receptors to accommodate several substrates in their interior domains and/or at their exterior surfaces.27 A series of dendrimer receptors were designed to have unsymmetrically distributed patches on the surfaces, which acted as the main binding sites of cytochrome c.28 They included unsymmetrically distributed polyanionic hepta(glutamic acids), a fluorescent zinc porphyrinate core, a hydrophilic polyether surface and peripheric, hydrophobic dendric components (Fig. 5). They were composed of the same hepta(glutamic acids) but different generations of hydrophobic dendritic interiors. Furthermore, the dendrimers have comparable molecular weights and diameters to those of the cytochrome cproteins: (12173–12588 Dalton and 4.0 nm); 2nd generation (3680 Dalton and 3.6 nm); 3rd generation (6371 Dalton and 5.0 nm); 4th generation (11753 Dalton and 6.0 nm). Thus, these dendrimer type receptors offered nanoscale protein recognition.
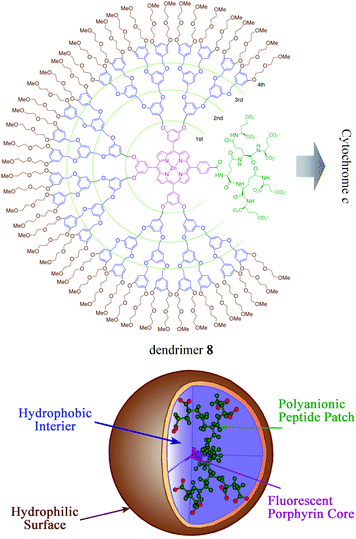 |
| Fig. 5
Dendrimer receptors for patch interactions with cytochrome c. Copyright Wiley-VCH Verlag GmbH & Co. KGaA. Reproduced from ref. 28 with permission. | |
Dendrimer receptors 8 of the 2nd, 3rd and 4th generations exhibited much larger log K values for horse heart cytochrome c at neutral pH (log K1 = 7.6 and log K2 = 7.2 for the 2nd generation, log K1 = 7.7 and log K2 = 7.2 for the 3rd generation and log K1 = 6.5 and log K2 = 7.1 for the 4th generation) than biological partner proteincytochrome b5 (log K = 4.8). These dendrimers included the same oligo(glutamic acids)-functionalized zinc porphyrinates for binding and signaling functions, but smaller dendrimers of the 2nd and 3rd generations exhibited higher binding constants than the larger dendrimers of the 4th generation, indicating the significance of the generation of the peripheric dendritic components. Both pigeon breast and yeast cytochromes c had similarly polycationic patches and formed complementary complexes with the dendrimers. Since these dendrimer receptors competed with cytochrome b5 in cytochrome c binding, both synthetic and biological receptors occupied the polycationic patch of cytochrome c in a similar fashion.
(b)
Apoptosis inhibition in living cells
Several synthetic receptors specifically bound cytochrome c in test tubes, but their cytotoxity, cell membrane permeability and related properties have rarely been characterized for uses in living cells. We found that dendrimers 8 of the 2nd, 3rd and 4th generations showed no significant cytotoxicity and inhibited ceramide-induced mitochondrial apoptosis in HeLa cells.29
Fig. 6 illustrates the outline of ceramide-induced mitochondrial apoptosis, in which cytochrome c is released from the mitochondria to the cytoplasm and activates pro-caspase-9 in the formation of apoptosome.21 The pretreatment of cells with dendrimer 8 of the 2nd generation typically decreased both the activity levels of caspase-3 and poly(ADP-ribose) polymerase, suggesting that the released cytochrome c was effectively trapped by the added dendrimer receptor in the cells. Although the employed biochemical assay experiments gave no direct evidence, the trapping of the released cytochrome c probably suppressed the downstream caspase cascade. In other words, the dendrimer receptors worked as a new-type of apoptosis inhibitors and are promising candidates as therapeutic agents for certain apoptosis-mediated diseases.
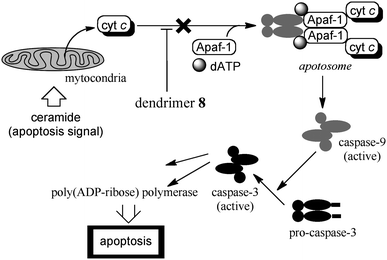 |
| Fig. 6
Dendrimer inhibition of ceramide-induced mitochondrial apoptosis. | |
5. Conclusion
This review is an overview of designed receptors of cytochrome c, and highlighted two examples that generated non-biological functions based on molecular recognition. Crown ether receptors are able to effectively interact with cationic residues of cytochrome c to give asymmetric catalysts working in organic media. Dendrimer receptors can bind cytochrome cvia complementary electrostatic interactions and further inhibited cell apoptosis. As discussed in previous studies,5 the development of effective receptors provides a fundamental understanding of biological cytochrome c recognition. Such development further offers an effective receptor strategy for the discovery of high-performance biosensors, practical catalysts and novel nanomedicines. Since many proteins exert multiple biological actions in the human body, the receptor strategy has wide applicability in the uses of many proteins as non-biological functionalized materials. This shows great promise, particularly in the field of nanomedicine. Targeted and/or controlled delivery systems of biologically interesting proteins and a series of bio-tools and clinical devices have received recent attention. Thus, the development of designed receptors is advancing research in various areas of chemical science and technology.
Acknowledgements
The authors deeply appreciate Professor Jesse L. Beauchamp of the California Institute of Technology, Professor Takeshi Nagasaki of Osaka City University and their research groups for valuable collaborations. They gratefully acknowledge financial support from the Japan Society for Promotion of Science. Permission to use Fig. 1, 4 and 5 were kindly given from the American Chemical Society and Wiley-VCH.
Notes and references
- Y. Lu, S. M. Berry and T. D. Pfister, Chem. Rev., 2001, 101, 3047 CrossRef CAS; M. A. Case and G. L. McLendon, Acc. Chem. Res., 2004, 37, 754 CrossRef; C. M. Thomas and T. R. Ward, Chem. Soc. Rev., 2005, 34, 337 RSC; Y. Lu, N. Yeung, N. Sieracki and N. M. Marshall, Nature, 2009, 460, 855 CrossRef.
- R. de la Rica and H. Matsui, Chem. Soc. Rev., 2010, 39, 3499 RSC; V. Köhler, Y. M. Wilson, C. Lo, A. Sardo and T. R. Ward, Curr. Opin. Biotechnol., 2010, 21, 744 CrossRef CAS; J. J. Yang, J. Yang, L. Wei, O. Zurkiya, W. Yang, S. Li, J. Zou, Y. Zhou, A. L. W. Maniccia, H. Mao, F. Zhao, R. Malchow, S. Zhao, J. Johnson, X. Hu, E. Krogstad and Z.-R. Liu, J. Am. Chem. Soc., 2008, 130, 9260 CrossRef; A. V. Patil and J. J. Davis, J. Am. Chem. Soc., 2010, 132, 16938 CrossRef.
- C. Renner, J. Piehler and T. Schrader, J. Am. Chem. Soc., 2006, 128, 620 CrossRef CAS.
- G. W. Gokel, W. M. Leevy and M. E. Weber, Chem. Rev., 2004, 104, 2723 CrossRef CAS.
- S. Fletcher and A. D. Hamilton, Curr. Opin. Chem. Biol., 2005, 9, 632 CrossRef CAS; V. Martos, P. Castreño, J. Valero and J. de Mendoza, Curr. Opin. Chem. Biol., 2008, 12, 698 CrossRef.
-
R. A. Scott and A. G. Mauk ed. “Cytochrome c: A Multidisciplinary Approach”, University Science Books, Sausalito, CA ( 1996) Search PubMed.
- S. Jiwpanich, B. S. Sandanaraj and S. Thayumanavan, Chem. Commun., 2009, 806 RSC; A. A. Reinke, P. M. Ung, J. J. Quintero, H. A. Carison and J. E. Gestwicki, J. Am. Chem. Soc., 2010, 132, 17655 CrossRef CAS; S. Machida, N. Kato, K. Harada and J. Ohkanda, J. Am. Chem. Soc., 2011, 133, 958 Search PubMed.
- J. A. Wells and C. L. McClendon, Nature, 2007, 450, 1001 CrossRef CAS; A. J. Wilson, Chem. Soc. Rev., 2009, 38, 3289 RSC.
- Y. Ren, W.-H. Wang, Y.-H. Wang, M. Case, W. Qian, G. McLendon and Z.-X. Huang, Biochemistry, 2004, 43, 3527 CrossRef CAS.
- I. Hamachi, A. Fujita and T. Kunitake, J. Am. Chem. Soc., 1997, 119, 9096 CrossRef CAS.
- H. Takashima, S. Shinkai and I. Hamachi, Chem. Commun., 1999, 2345 RSC.
- L. Baldini, A. J. Wilson, J. Hong and A. D. Hamilton, J. Am. Chem. Soc., 2004, 126, 5656 CrossRef CAS.
- Y. Hamuro, M. C. Calama, H. S. Park and A. D. Hamilton, Angew. Chem., Int. Ed. Engl., 1997, 36, 2680 CrossRef CAS.
- R. C. Lasey, L. Liu, L. Zang and M. Y. Ogawa, Biochemistry, 2003, 42, 3904 CrossRef CAS.
- S. Hirota, M. Endo, K. Hiyamizu, T. Tsukazaki, T. Takabe, T. Kohzuma and O. Yamauchi, J. Am. Chem. Soc., 1999, 121, 849 CrossRef CAS.
- A. Hirsch, Pure Appl. Chem., 2008, 80, 571 CrossRef CAS.
- K. Shimojo, N. Kamiya, F. Tani, H. Naganawa, Y. Naruta and M. Goto, Anal. Chem., 2006, 78, 7735 CrossRef CAS; K. Fujita, D. R. MacFarlane, M. Forsyth, M. Yoshizawa-Fujita, K. Murata, N. Nakamura and H. Ohno, Biomacromolecules, 2007, 8, 2080 CrossRef; M. Bihari, T. P. Russell and D. A. Hoagland, Biomacromolecules, 2010, 11, 2944 CrossRef.
- H. Bayraktar, C.-C. You, V. M. Rotello and M. J. Knapp, J. Am. Chem. Soc., 2007, 129, 2732 CrossRef CAS; A. F. Loftus, K. P. Reighard, S. A. Kapourales and M. C. Leopold, J. Am. Chem. Soc., 2008, 130, 1649 CrossRef; A. Hung, S. Mwenifumbo, M. Mager, J. J. Kuna, F. Stellacci, I. Yarovsky and M. M. Stevens, J. Am. Chem. Soc., 2011, 133, 1438 CrossRef.
- S. Rana, Y.-C. Yeh and V. M. Rotello, Curr. Opin. Chem. Biol., 2010, 14, 828 CrossRef CAS; L. Li, Q. Mu, B. Zhang and B. Yan, Analyst, 2010, 135, 1519 RSC.
- R. Minchin, Nat. Nanotechnol., 2008, 3, 12 CrossRef CAS.
- S. Orrenius, Toxicol. Lett., 2004, 149, 19 CrossRef CAS; Y.-L. P. Ow, D. R. Green, Z. Hao and T. W. Mak, Nat. Rev. Mol. Cell Biol., 2008, 9, 532 CrossRef.
- B. Odell and G. Earlam, J. Chem. Soc., Chem. Commun., 1985, 359 RSC.
- R. R. Julian and J. L. Beauchamp, Int. J. Mass Spectrom., 2001, 210/211, 613 CrossRef CAS.
- N. A. Belikova, Y. Y. Tyurina, G. Borisenko, V. Tyurin, A. K. S. Arias, N. Yanamala, P. G. Furtmüller, J. Klein-Seetharaman, C. Obinger and V. E. Kagan, J. Am. Chem. Soc., 2009, 131, 11288 CrossRef CAS.
- D. Paul, A. Suzumura, H. Sugimoto, J. Teraoka, S. Shinoda and H. Tsukube, J. Am. Chem. Soc., 2003, 125, 11478 CrossRef CAS.
- A. Suzumura, D. Paul, H. Sugimoto, S. Shinoda, R. R. Julian, J. L. Beauchamp, J. Teraoka and H. Tsukube, Inorg. Chem., 2005, 44, 904 CrossRef CAS.
- D. Astruc, E. Boisselier and C. Ornelas, Chem. Rev., 2010, 110, 1857 CrossRef CAS.
- D. Paul, H. Miyake, S. Shinoda and H. Tsukube, Chem.–Eur. J., 2006, 12, 1328 CrossRef CAS.
- H. Azuma, Y. Yoshida, D. Paul, S. Shinoda, H. Tsukube and T. Nagasaki, Org. Biomol. Chem., 2009, 7, 1700 CAS.
|
This journal is © The Royal Society of Chemistry 2011 |