DOI:
10.1039/C0SC00635A
(Edge Article)
Chem. Sci., 2011,
2, 862-867
Received
20th December 2010
, Accepted 21st February 2011
First published on 8th March 2011
Abstract
We have been developing host–guest methodology for the separation of single-walled carbon nanotubes (SWNTs) according to handedness and diameter with gable-type chiral diporphyrins. The diporphyrins, designated as porphyrin nanotweezers, consist of two porphyrins and rigid spacer in between. As an extension of our strategy, novel nanotweezers having two pyrenes instead of porphyrins have been designed, because pyrene is known to have high affinity toward SWNT surface. The pyrene nanotweezers presented here consist of two 1- or 2-pyrenes and 3,6-carbazolylenes with various N-substituents. For the extraction of SWNTs, the 1- and 2-pyrene nanotweezers show a marked contrast: 1-pyrene nanotweezers selectively extract SWNTs with diameters ranging from 0.84 nm to 0.97 nm, while 2-pyrene nanotweezers are not able to extract SWNTs at all.
Introduction
Single-walled carbon nanotubes (SWNTs) possess excellent mechanical, optical and electrical properties, which have made them attractive candidates for many applications.1 As the electronic and optical properties are largely dependent on their structures,2 structural control of SWNTs is very important for their applications in nanoelectronics and nanooptics. While the selective synthesis of metallic and semiconducting SWNTs,3,4 and (6,5)-SWNTs5 has been accomplished quite recently, as-grown SWNTs usually consist of complex mixtures containing many different structures. Such heterogeneity in length, diameter, and roll-up index, or (n, m), of SWNTs has hindered their ultimate utility. Therefore, post-growth separation of SWNTs is a general approach in obtaining selected diameters and (n, m) structures.6,7 Several methods have been developed for separation of SWNTs such as density gradient ultracentrifugation (DGU),8–13electrophoresis,14chromatography,15–18polymer-assisted selective extraction,19–23 and electrochemical doping.24–26 Relatively small molecules have also been utilized for separation of SWNTs through recognition of a specific (n, m) structure.22,23,27–29
In this context, we have been developing host–guest methodology for separation of SWNTs with chiral gable-type diporphyrins, designated as porphyrin nanotweezers.30–34 The chiral porphyrin nanotweezers discriminated the handedness and diameter simultaneously, giving optically active SWNTs with limited (n, m) structures. Since pyrene is well known to have great affinity for the surface of SWNTs like porphyrin,35–37 we designed pyrene nanotweezers consisting of two pyrenes instead of porphyrins and a rigid spacer in between. Diameter or (n, m) structure of SWNTs is expected to be recognized by the pyrene nanotweezers, giving SWNTs with limited structure.22,35,38 While chiral diporphyrin nanotweezers were synthesized via several steps,30 the pyrene nanotweezers are much more facile to prepare as shown in Scheme 1. In addition, SWNTs with one or limited (n, m) structures are sufficient for most applications; that is, optically enriched SWNTs are rarely required at present in view of practical applications of SWNTs. Therefore, pyrene nanotweezers are more practical than porphyrin ones in separation of SWNTs by molecular recognition. In this paper, we report on the novel nanotweezers, consisting of two pyrenes with an N-substituted carbazole in between, their synthesis, extraction ability, and diameter selectivity.
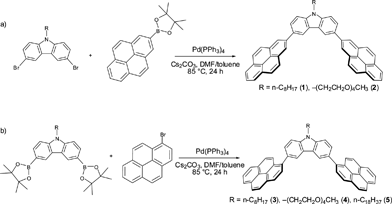 |
| Scheme 1 Synthesis of 2-pyrene nanotweezers 1–2 (a) and 1-pyrene nanotweezers 3–5 (b) viaSuzuki-Miyaura coupling reactions. | |
Results and discussion
Synthesis and structure of dipyrene nanotweezers
As in the case of the porphyrin nanotweezers, the pyrene nanotweezers were synthesized viaSuzuki-Miyaura coupling reaction of pyrene derivatives with N-substituted carbazoles (Scheme 1). Although Suzuki-Miyaura coupling reaction was employed in the final step of the preparation of both pyrene and porphyrin nanotweezers, the pyrene derivatives used for Suzuki-Miyaura coupling reaction are much more facile to prepare than the chiral monoporphyrin derivatives. The pyrene-2-boronate was directly synthesized from commercial pyrene through Ir-catalyzed C–H bond activation.39 It was coupled with N-substituted 3,6-dibromocarbazoles to give 3,6-bis(2′-pyrenyl)carbazoles 1 and 2, designated as 2-pyrene nanotweezers, in 45% and 35% respectively, as shown in Scheme 1a. On the other hand, the commercial 1-bromopyrene was reacted with the N-substituted 3,6-bis(2-dioxaborolanyl)carbazoles to give N-substituted 3,6-bis(1′-pyrenyl)carbazoles 3–5, designated as 1-pyrene nanotweezers, in 70%, 32% and 45% respectively (Scheme 1b). As in the case of the synthesis of porphyrin nanotweezers,31 either combination of bromide and boronate worked in the synthesis of pyrene nanotweezers through Suzuki coupling reaction. The combinations shown in Scheme 1 were chosen by considering the availability of the compounds employed for the Suzuki coupling reaction. The 1- and 2-pyrene nanotweezers thus synthesized were characterized by 1H NMR, 13C NMR, mass, and absorption spectroscopies, and melting points (see experimental section in the ESI†).
In 1-pyrene nanotweezers, two diastereomers, meso and dl, are conceivable as shown in Charts 1a and 1b, if the rotation of the 1-pyrenes is constrained under ambient conditions. Since it is reported that a minimum of three ortho substituents are required to impart a sufficient barrier to prevent biphenyls from rotating,40 our triaryl systems can freely rotate and hence no configurational stereoisomers have to be considered in 1-pyrene nanotweezers. In fact, no change in 1H NMR spectra was observed even at −60 °C (Fig. S1 in the ESI†), indicating that the structures shown in Charts 1a and 1b are not the configurational stereoisomers, but the conformational stereoisomers. 1-Pyrene nanotweezers take one of the conformational stereoisomers, when they form complex with SWNTs, as shown in Fig. 5.
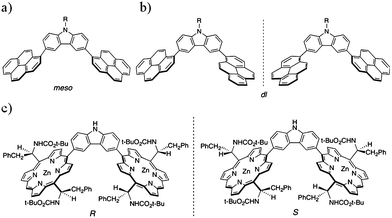 |
| Chart 1 Structures of 1-pyrene and porphyrin nanotweezers; conformational stereoisomers of 1-pyrene nanotweezers, meso (a) and dl (b), and configurational stereoisomers of porphyrin nanotweezers (c). | |
Commercial CoMoCAT (SWNTs prepared by chemical vapor deposition (CVD) process using a silica-supported Co–Mo catalyst) was employed for the extraction with pyrene nanotweezers.41 Since the porphyrin nanotweezers with carbazole spacers (Chart 1c) exhibited selectivity toward the SWNTs around 0.85 nm in diameters,32 we mainly used commercial 76-CoMoCAT, where SWNTs with 0.85–0.90 nm are enriched, rather than 65-CoMoCAT, which includes smaller diameters predominantly. In fact, neither 1- nor 2-pyrene nanotweezers extracted 65-CoMoCAT, implying that the pyrene nanotweezers prefer larger diameters as in the case of the porphyrin nanotweezers shown in Chart 1c.32 When 76-CoMoCAT was used, 1-pyrene nanotweezers successfully extracted SWNTs in the methanol layer to give blackish supernatant after centrifugation. However, no SWNTs were extracted by use of 2-pyrene nanotweezers under the same conditions. A marked contrast was observed in the extraction ability between these positional isomers. This is probably because of the difference in the solubility of these nanotweezers in methanol and stability of the complexes. Since 2-pyrene nanotweezers 1 is not soluble in methanol, it was not able to extract SWNTs at all. Although 2-pyrene nanotweezers 2 exhibit similar solubility to 1-pyrene nanotweezers, SWNTs were not extracted in methanol with 2. This may be attributed to difference in the complex structures. The comparison of the complex structures and their stability will be discussed below by use of the computer generated molecular models.
In the extraction, much longer time (65 h, see experimental section in the ESI†) of bath-sonication was needed to exfoliate the bundles of the SWNTs with 1-pyrene nanotweezers, as compared with the case of diporphyrin nanotweezers shown in Chart 1c.32 This is probably because of the lower affinity of pyrene to the SWNT surface than porphyrin. As for the solvent, methanol was found to be a solvent of choice. All the 1-pyrene nanotweezers 3–5 are much better soluble in chloroform, dichloromethane, ethyl acetate, toluene, tetrahydrofuran (THF), and acetone than in alcohols. However, no SWNTs were extracted in these solvents and only alcoholic solvents such as methanol, ethanol, and 2-propanol can dissolve the complex formed by 1-pyrene nanotweezers and SWNTs. Similar phenomena have been observed in the case of porphyrin nanotweezers.30–34,42 This implies the polarity of the complexes between the porphyrin or pyrene and SWNTs. In addition to their π–π interaction, ionic interaction caused by (partial) electron transfer might conceivably give rise to the polarity of the complex.43–45 The polarity of the resulting electron donor–acceptor (EDA) complexes is considered to make them stable and soluble in methanol.
In the methanol supernatant after centrifugation, new absorption bands were observed in the absorption spectra (Figure S2 in the ESI†) as broad bands at 1010 and 1158 nm corresponding to those of SWNTs. After concentration of the supernatant, the residual complex was washed with THF, which solubilized 1-pyrene nanotweezers very well, but did not dissolve the complex, as mentioned above. As a result, 0.28, 0.25, and 0.09 mg of SWNTs were recovered from the extracts with 1-pyrene nanotweezers 3, 4, and 5, respectively. The difference in their extractability was attributed to the solubility in methanol; that is, a long alkyl chain in 5 may decrease the solubility of the SWNT complex of 5 in methanol, while appropriate chain length such as the octyl group and appropriate polarity in the tetraethylene glycol group helped solubilize the complexes of 3 and 4 in methanol. The substituent at N-position in the carbazole spacer turned out to be useful, because various groups could be readily introduced at the N-position and could serve as controllers in the extractability of the nanotweezers.
Diameter selectivity of SWNTs extracted with dipyrene nanotweezers
In order to determine the selectivity, we measured Raman spectra of the solid samples after washing, and absorption and photoluminescence (PL) spectra of the solution prepared by dissolving the solid samples in D2O with the aid of sodium dodecylbenzenesulfonate (SDBS). Since the amount of extracted SWNTs with 5 was not enough to obtain decent spectra after solubilization, only the Raman spectrum is shown here.
In the PL spectra shown in Fig. 1, (7,6)-, (8,4)-, (9,4)-, and (8,6)-SWNTs were enriched significantly in the extraction of 76-CoMoCAT with 1-pyrene nanotweezers 3 and 4. In contrast, (6,5)-, (7,5)-, and (8,3)-SWNTs decreased relatively. The enriched SWNTs, (7,6)-, (8,4)-, (9,4)-, and (8,6)-SWNTs have diameters ranging from 0.84 nm to 0.97 nm, while SWNTs having diameters less than 0.84 nm, (6,5)-, (7,5)-, and (8,3)-SWNTs, relatively decreased after the extraction. This clearly indicates that the 1-pyrene nanotweezers recognize the diameter of SWNTs. For the extraction of HiPco including larger diameters with 1-pyrene nanotweezers 3, the content of SWNTs with diameters larger than 0.97 nm, for example (8,7)- and (10,5)-SWNTs, decreased relatively as shown in the PL spectra (Fig. S3 in the ESI†). These results clearly show that 1-pyrene nanotweezers selectively extract the SWNTs with 0.84–0.97 nm in diameters.
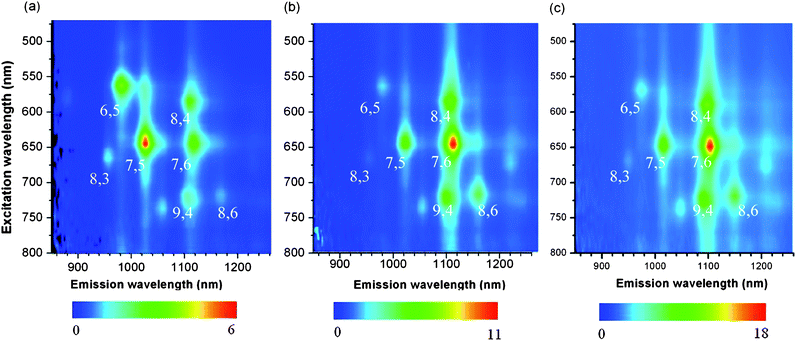 |
| Fig. 1 Photoluminescence (PL) spectra of 76-CoMoCAT before (a) and after extraction with 3 (b) and 4 (c). The scale intensity is shown below the spectra. | |
Absorption and Raman spectra shown in Fig. 2 and Fig. 3, respectively, support the above conclusion of the diameter selectivity of 1-pyrene nanotweezers. After the extraction of 76-CoMoCAT, the absorption bands for (8,4)-, (7,6)-, (9,4)-, and (8,6)-SWNTs increase relatively in the region of ES11, while the contents of the smaller diameter, (6,4), (6,5), (8,3), and (7,5)-SWNTs, are largely decreased. Raman spectra of as-obtained 76-CoMoCAT and the extracted solid samples were measured at the excitation wavelength of 633 nm. The peak intensity of (7,6), (9,4), and (10,3)-SWNTs, having diameter ranging from 0.90 nm to 0.94 nm, enhanced after the extraction, while those of (7,5), (8,3), (6,5), and (6,4)-SWNTs decreased relatively. Since photoluminescence of (10,3)-SWNTs is hardly observed in the PL spectra (excitation at 632 nm and emission at 1249 nm in Fig. 1), the actual content of (10,3)-SWNTs may be negligible. The Raman scattering from (10,3)-SWNTs is emphasized because of the strong resonance of (10,3)-SWNTs at the excitation wavelength of 633 nm.
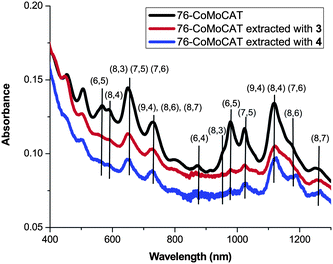 |
| Fig. 2 UV–vis–NIR spectra of D2O/SDBS solutions of 76-CoMoCAT and the one extracted with 3 and 4. | |
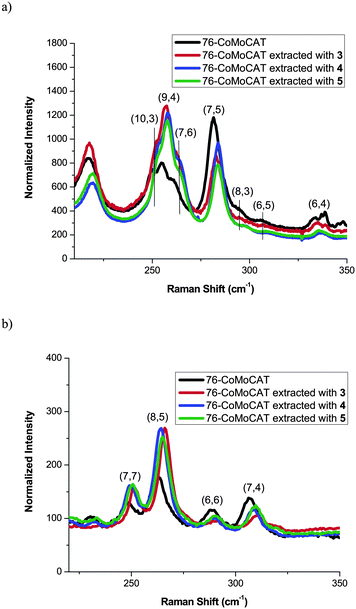 |
| Fig. 3
Raman spectra of 76-CoMoCAT before and after extraction with 3, 4, and 5 in the region of radial breathing mode at the excitation wavelength of 633 nm (a) and 488 nm (b). The intensity was normalized at G band. G and D band regions are shown in Fig. S4 in the ESI.† | |
In order to know the selectivity in the metallic SWNTs, the Raman spectra were also measured at an excitation of 488 nm as shown in Fig. 3b. (8,5)- and (7,7)-SWNTs having diameters of 0.85 nm and 0.95 nm, respectively, increase relatively, while (7,4)- and (6,6)-SWNTs having diameters of 0.75 nm and 0.81 nm, respectively, decreased. This is totally consistent with the above conclusion that SWNTs with diameters ranging from 0.84 nm to 0.97 nm are selectively extracted by the 1-pyrene nanotweezers 3–5. This result of the metallic SWNTs also indicates that the 1-pyrene nanotweezers 3–5 recognize only the diameter and not the electrical properties.
The results of the (n, m) enrichments mentioned above through the extraction with the 1-pyrene nanotweezers are summarized in the graphene map shown in Fig. 4. The map clearly shows that 1-pyrene nanotweezers discriminate diameters of SWNTs rather than roll-up angles. This is consistent with the case of the porphyrin nanotweezers possessing a carbazole as a spacer, where the porphyrin nanotweezers recognize diameter to accept SWNTs inside the cavity deeply. The relation between the complex structure and the diameter selectivity will be discussed in the following section.
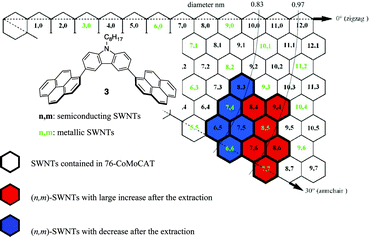 |
| Fig. 4 Summary of enrichment in (n, m) abundance in the extraction of 76-CoMoCAT with 3. The diameter corresponds to the length between the origin (the point where the two dotted lines crossed) and the (n, m) (left-up vertex in the (n, m) hexagon) in the map. | |
Theoretical calculation for complex structures
For theoretical support and interpretation of the above experimental results, we carried out theoretical calculations of the complexes of 1, meso-3, and dl-3 with (6,5)-, (8,3)-, (7,5)-, (8,4)-, (7,6)-, (9,4)-, (8,6)-, and (8,7)-SWNTs (the major components of 76-CoMoCAT). The typical complex structures of 1, meso-3, and dl-3 with (7,6)-SWNTs are illustrated in Fig. 5. The association enthalpies in the complex of SWNTs with meso- and dl-3 (Fig. 5b and 5c) are almost the same, indicating that the SWNT complexes with meso- and dl-3 may exist in almost equal amounts in the extract. The pyrene nanotweezers, 1, meso-3, and dl-3, have almost the same dihedral angles (86.7 – 86.9°) and the same distances between the two ipso-carbons connected to carbazole (0.813–0.814 nm) before complexation with SWNTs, as shown in Table 1. Upon complexation with the major components of 76-CoMoCAT, all the distances between the ipso-carbons at 2-position (C2–C2′) in 1 become smaller. The deformation of the structure of 1 destabilizes the complex and hence no SWNTs are considered to be extracted with 1. On the other hand, no (or only slight) change is observed in the distances between the carbons at 1-position (C1–C1′) in 3 upon complexation with (8,4)-, (10,2)-, (7,6)-, (9,4)- and (8,6)-SWNTs having diameters of 0.84–0.97 nm. This theoretical calculation supports the above conclusion of the diameter selectivity of 1-pyrene nanotweezers, which was derived from the experimental results. The (n, m)-SWNTs giving the least deformation of the 1-pyrene nanotweezers 3 upon complexation are concluded to be extracted selectively.
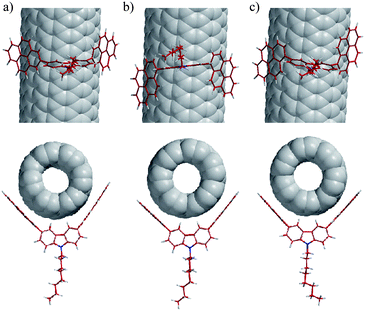 |
| Fig. 5 Computer-generated complex structures of 1 (a), meso-3 (b), and dl-3 (c) with (7,6)-SWNTs. Views of these complexes along the SWNT axis are shown below the models (a)–(c). | |
Table 1 Change in distances between two ipso-carbons of pyrenes in 1, meso-3, and dl-3 before and after complexation with SWNTsa
Major (n, m) components in 76-CoMoCAT |
Diameter (nm) |
Roll-up angle (deg.) |
Distance (nm) between two ipso-carbons of pyrenes |
C2–C2′ in 1b |
C1–C1′ in meso-3c |
C1–C1′ in dl-3c |
Molecular mechanics calculation.
C2 and C2′ are the carbons at 2-position in pyrenes.
C1 and C1′ are the carbons at 1-position in pyrenes.
|
— |
— |
— |
0.814 |
0.814 |
0.813 |
(6,5) |
0.76 |
27.0 |
0.802 |
0.806 |
0.809 |
(8,3) |
0.78 |
15.3 |
0.805 |
0.808 |
0.810 |
(7,5) |
0.83 |
24.5 |
0.808 |
0.809 |
0.810 |
(8,4) |
0.84 |
19.1 |
0.808 |
0.810 |
0.812 |
(7,6) |
0.90 |
27.5 |
0.809 |
0.813 |
0.812 |
(9,4) |
0.92 |
17.5 |
0.810 |
0.813 |
0.812 |
(8,6) |
0.97 |
25.3 |
0.811 |
0.813 |
0.813 |
(8,7) |
1.03 |
27.8 |
0.813 |
0.815 |
0.816 |
Conclusions
We have demonstrated the diameter selective separation of SWNTs with newly designed pyrene nanotweezers consisting of two pyrenes and a carbazolylene spacer in between. The extraction ability of the pyrene nanotweezers has been found to depend strongly on their structural features; 1-pyrene nanotweezers 3–5 successfully extracted SWNTs with diameters ranging from 0.84 nm to 0.97 nm, while 2-pyrene nanotweezers 1–2 did not show any extraction ability at all. The marked difference in the extraction ability may be ascribed to the difference in the solubility and the stability of the complexes, which is estimated from computer-generated complex structures. As compared with the porphyrin nanotweezers previously reported by us, the pyrene ones have advantages in practical separation by diameter of SWNTs because of their facile synthesis. We are convinced to improve the discrimination ability of the pyrene nanotweezers by changing the spacer, as in the case of the porphyrin nanotweezers, and accomplish more practical and precise diameter separation of SWNTs.
Acknowledgements
We thank Professor Hiroshi Imahori (Kyoto University) for allowing us to use a PL spectrophotometer, Professor Atsuhiro Osuka (Kyoto University) for encouraging us, Mr. Ryohei Okamoto (Shiga University of Medical Science) for helping with low temperature NMR experiments, and Mr. Takayoshi Irie (Osaka Electro-Communication University) for helping with MALDI-TOF MS measurement. A. F. M. M. R. acknowledges Japan Society for the Promotion of Science (JSPS) for providing his postdoctoral fellowship (No. 2008350). This work was financially supported by Grant-in-Aid for Scientific Research on Priority Areas (No. 20048003 and 20048004), Grant-in-Aid for Scientific Research (B) (No. 20350064), and the Industrial Technology Research Grant Program in 2005 from the New Energy and Industrial Technology Development Organisation (NEDO) of Japan.
Notes and references
- R. H. Baughman, A. A. Zakhidov and W. A.d. Heer, Science, 2002, 297, 787 CrossRef CAS.
- H. Kataura, Y. Kumazawa, Y. Maniwa, I. Umezu, S. Suzuki, Y. Ohtsuka and Y. Achiba, Synth. Met., 1999, 103, 2555 CrossRef CAS.
- A. R. Harutyunyan, G. Chen, T. M. Paronyan, E. M. Pigos, O. A. Kuznetsov, K. Hewaparakrama, S. M. Kim, D. Zakharov, E. A. Stach and G. U. Sumanasekera, Science, 2009, 326, 116 CrossRef CAS.
- G. Hong, B. Zhang, B. Peng, J. Zhang, W. M. Choi, J.-Y. Choi, J. M. Kim and Z. Liu, J. Am. Chem. Soc., 2009, 131, 14642 CrossRef CAS.
- Z. Ghorannevis, T. Kato, T. Kaneko and R. Hatakeyama, J. Am. Chem. Soc., 2010, 132, 9570 CrossRef CAS.
- N. Komatsu and F. Wang, Materials, 2010, 3, 3818 Search PubMed.
- C. N. R. Rao, R. Voggu and A. Govindaraj, Nanoscale, 2009, 1, 96 RSC.
- S. Ghosh, S. M. Bachilo and R. B. Weisman, Nat. Nanotechnol., 2010, 5, 443 CrossRef CAS.
- A. A. Green, M. C. Duch and M. C. Hersam, Nano Res., 2009, 2, 69 CrossRef CAS.
- A. A. Green and M. C. Hersam, Nano Lett., 2008, 8, 1417 CrossRef CAS.
- M. S. Arnold, S. I. Stupp and M. C. Hersam, Nano Lett., 2005, 5, 713 CrossRef CAS.
- M. S. Arnold, A. A. Green, J. F. Hulvat, S. I. Stupp and M. C. Hersam, Nat. Nanotechnol., 2006, 1, 60 CrossRef CAS.
- N. Stürtl, F. Hennrich, S. Lebedkin and M. M. Kappes, J. Phys. Chem. C, 2009, 113, 14628 CrossRef.
- R. Krupke, F. Hennrich, H.v. Löhneysen and M. M. Kappes, Science, 2003, 301, 344 CrossRef CAS.
- X. Tu, S. Manohar, A. Jagota and M. Zheng, Nature, 2009, 460, 250 CrossRef CAS.
- M. Zheng and E. D. Semke, J. Am. Chem. Soc., 2007, 129, 6084 CrossRef CAS.
- L. Zhang, S. Zaric, X. Tu, X. Wang, W. Zhao and H. Dai, J. Am. Chem. Soc., 2008, 130, 2686 CrossRef CAS.
- L. Zhang, X. Tu, K. Welsher, X. Wang, M. Zheng and H. Dai, J. Am. Chem. Soc., 2009, 131, 2454 CrossRef CAS.
- F. Chen, B. Wang, Y. Chen and L.-J. Li, Nano Lett., 2007, 7, 3013 CrossRef CAS.
- J.-Y. Hwang, A. Nish, J. Doig, S. Douven, C.-W. Chen, L.-C. Chen and R. J. Nicholas, J. Am. Chem. Soc., 2008, 130, 3543 CrossRef CAS.
- A. Nish, J.-Y. Hwang, J. Doig and R. J. Nicholas, Nat. Nanotechnol., 2007, 2, 640 CrossRef CAS.
- R. Marquis, K. Kulikiewicz, S. Lebedkin, M. M. Kappes, C. Mioskowski, S. Meunier and A. Wagner, Chem.–Eur. J., 2009, 15, 11187 CrossRef CAS.
- R. Marquis, C. Greco, I. Sadokierska, S. Lebedkin, M. M. Kappes, T. Michel, L. Alvarez, J.-L. Sauvajol, S. Meunier and C. Mioskowski, Nano Lett., 2008, 8, 1830 CrossRef CAS.
- S. Niyogi, S. Boukhalfa, S. B. Chikkannanavar, T. J. McDonald, M. J. Heben and S. K. Doorn, J. Am. Chem. Soc., 2007, 129, 1898 CrossRef CAS.
- M. Kalbác, L. Kavan and L. Dunsch, J. Am. Chem. Soc., 2009, 131, 4529 CrossRef CAS.
- Y. Kato, Y. Niidome and N. Nakashima, Angew. Chem., Int. Ed., 2009, 48, 5435 CrossRef CAS.
- O. O. Ogunro and X.-Q. Wang, Nano Lett., 2009, 9, 1034 CrossRef CAS.
- S.-Y. Ju, J. Doll, I. Sharma and F. Papadimitrakopoulos, Nat. Nanotechnol., 2008, 3, 356 CrossRef CAS.
- R. M. Tromp, A. Afzali, M. Freitag, D. B. Mitzi and Z. Chen, Nano Lett., 2008, 8, 469 CrossRef CAS.
- X. Peng, F. Wang, A. K. Bauri, A. F. M. M. Rahman and N. Komatsu, Chem. Lett. (Highlight Review), 2010, 39, 1022 Search PubMed.
- F. Wang, K. Matsuda, A. F. M. M. Rahman, X. Peng, T. Kimura and N. Komatsu, J. Am. Chem. Soc., 2010, 132, 10876 CrossRef CAS.
- X. Peng, N. Komatsu, T. Kimura and A. Osuka, ACS Nano, 2008, 2, 2045 CrossRef CAS.
- X. Peng, N. Komatsu, T. Kimura and A. Osuka, J. Am. Chem. Soc., 2007, 129, 15947 CrossRef CAS.
- X. Peng, N. Komatsu, S. Bhattacharya, T. Shimawaki, S. Aonuma, T. Kimura and A. Osuka, Nat. Nanotechnol., 2007, 2, 361 CrossRef CAS.
- C. Backes, U. Mundloch, A. Ebel, F. Hauke and A. Hirsch, Chem.–Eur. J., 2010, 16, 3314 CrossRef CAS.
- W. Wang, K. A. S. Fernando, Y. Lin, M. J. Meziani, L. M. Veca, L. Cao, P. Zhang, M. M. Kimani and Y.-P. Sun, J. Am. Chem. Soc., 2008, 130, 1415 CrossRef CAS.
- N. Nakashima, Y. Tomonari and H. Murakami, Chem. Lett., 2002, 31, 638 CrossRef.
- C. Backes, C. D. Schmidt, F. Hauke and A. Hirsch, Chem. Asian J., 2011, 6, 438 CrossRef CAS.
- D. N. Coventry, A. S. Batsanov, A. E. Goeta, J. A. K. Howard, T. B. Marder and R. N. Perutz, Chem. Commun., 2005, 2172 RSC.
- A. I. Meyers and R. J. Himmelsbach, J. Am. Chem. Soc., 1985, 107, 682 CrossRef CAS.
- S. M. Bachilo, L. Balzano, J. E. Herrera, F. Pompeo, D. E. Resasco and R. B. Weisman, J. Am. Chem. Soc., 2003, 125, 11186 CrossRef CAS.
- X. Peng, N. Komatsu, T. Kimura and A. Osuka, J. Phys. Chem. C, 2009, 113, 9108 CrossRef CAS.
- D. M. Guldi, E. Menna, M. Maggini, M. Marcaccio, D. Paolucci, D. Paolucci, S. Campidelli, M. Prato, G. M. A. Rahman and S. Schergna, Chem.–Eur. J., 2006, 12, 3975 CrossRef CAS.
- D. M. Guldi, G. M. A. Rahman, N. Jux, N. Tagmatarchis and M. Prato, Angew. Chem., Int. Ed., 2004, 43, 5526 CrossRef CAS.
- K. Datta, M. Banerjee, B. K. Seal and A. K. Mukherjee, J. Chem. Soc., Perkin Trans. 2, 2000, 2, 531 Search PubMed.
|
This journal is © The Royal Society of Chemistry 2011 |