DOI:
10.1039/C1RA00133G
(Paper)
RSC Adv., 2011,
1, 611-618
Photocatalytic degradation of linuron in aqueous suspensions of TiO2
Received
3rd May 2011
, Accepted 5th July 2011
First published on 23rd August 2011
Abstract
The photocatalytic degradation of linuron (3-(3,4-dichlorophenyl)-1-methoxy-1-methylurea), a widely used herbicide and potential environmental contaminant, in irradiated titanium dioxide aqueous suspensions is investigated. The photocatalytic performance of two nano-sized materials prepared by a sol–gel process, and the influence on the degradation rate of some operational parameters, such as catalyst amount, pH of the medium and hydrogen peroxide concentration, are evaluated. Within the range of the explored experimental conditions, degradation of linuron in an aqueous suspension of TiO2 at mg l−1 contamination levels is described by a pseudo-first-order kinetic model, the apparent half-life time of the herbicide being about 30 min in optimal conditions. Based on the evolution of total organic carbon and the fate of inorganic species, the disappearance of linuron seems to be accompanied by its almost quantitative mineralization.
Introduction
Heterogeneous photocatalysis based on semiconductors is extensively investigated in view of its applications in the detoxification of polluted water.1–3 In this context, titanium dioxide, owing to its remarkable activity together with low cost, chemical inertness, robustness to UV irradiation and non-toxicity, is a greatly promising material. The detailed mechanism of the TiO2-mediated photodegradation of organic compounds has been extensively described in the literature.3–5 Briefly, electron–hole pairs are generated when this material is irradiated with light energy greater than its band-gap energy (3.2 eV, corresponding to wavelengths below 380 nm), which results in the coexistence of an oxidative and reductive environment on the semiconductor surface. Oxidation, or, less frequently, reduction occurs when adsorbed molecules directly react with photogenerated holes or electrons, respectively. Alternatively, degradation of contaminants is promoted by highly reactive radical species resulting from the reaction of TiO2 with the chemical components of the aqueous medium. Typically, OH˙ radicals with very high oxidizing power are generated by the reaction of water or hydroxyl ions with the charges–holes, while superoxide anion O2−· or hydroperoxide radicals HO2˙ are generated by reaction of dissolved oxygen with the photogenerated electrons. A large variety of organic compounds, including many potential pollutants,6–11 are quickly degraded in irradiated TiO2 aqueous suspensions and mineralization of most of these chemicals has been observed, finally leading to harmless products (water, carbon dioxide and inorganic ionic species).
Improvement of the photodecontamination performance of irradiated TiO2 suspensions can be in principle based on the optimisation of several physico-chemical parameters related with both reaction conditions and morphological and electronic properties of the semiconductor.7,12 As to the first aspect, pH of the medium, concentration of naturally occurring or added chemical species can be tuned in order to prevent electron–hole recombination or to exalt the oxidative (or reductive) character of the photocatalytic system.6,10,13 On the other side, several modifications of TiO2, for instance doping with transition metal ions or nonmetals, aimed at decreasing the band gap energy are attempted to make use of the full spectrum of solar light.12,14,15 Further, as typical in heterogeneous catalysis, in which the working arena for the reaction is the medium–catalyst interphase, catalytic efficiency is expected to improve by increasing the surface area.12 In this perspective, a remarkable surface-to-volume ratio becomes available when TiO2 size is decreased down to the nanometre scale.
In this paper, the photocatalytic ability of nano-sized TiO2 prepared in our laboratory by means of two different sol–gel methodologies are tested on linuron, 3-(3,4-dichlorophenyl)-1-methoxy-1-methylurea, a widely used phenylurea herbicide. Phenylureas, extensively employed to contrast weed growth in many crops, are persistent pesticides mainly exhibiting a low microbial degradation in the environment, that, as the consequence of massive use, can contaminate surface and ground water.16 Linuron, which has an observed half-life in soil from 38 to 67 days,17 deserves particular attention because of its potential carcinogenic effect on humans18 and, as well as its terminal metabolite 3,4-dichloroaniline, documented endocrine disrupting action.19 Previously, photodegradation of linuron under UV irradiation combined with hydrogen peroxide20 or Fenton's reagent21–23 was investigated, but, to the best of our knowledge, photodegradation potentiality of TiO2 has not been tested yet on this pesticide, despite a great attention being devoted to TiO2-mediated photodegradation of several phenylurea herbicides.24–31
Experimental
Chemicals and reagents
Reagents, solvents, and inorganic acids and bases used to prepare the photocatalysts, all of analytical grade, were obtained from Sigma-Aldrich (St. Louis, MO, USA). Linuronstandard (purity 99.9%) was obtained from Labor Dr Ehrenstorfer-Schäfers (Augsburg, Germany) and was used without further purification. A stock solution (1.00 g l−1) of linuron was prepared by dissolving accurately-weighed 10 mg of pesticide in 10 ml of acetonitrile (chromatographic grade, Carlo Erba Reagenti, Milano, Italy), from which, after appropriate dilution a working aqueous solution (1 mg l−1) was finally obtained and stored at 4 °C. Distilled water obtained by a milli-Q water filtration/purification system (Millipore, Bedford, MA, USA) was used.
A sol–gel process under two different experimental conditions (methods A and B) was used to prepare the photocatalyst. According to protocol A, 150 ml of titanium butoxide was dissolved in ethanol along with 15 ml of acetyl-acetone acting as a complexing agent. In order to control the final grain size of TiO2 crystallites, 12 g of the surfactant cetyl trimethyl ammonium bromide was dissolved in 50 ml of ethanol and 5 ml of concentrated HCl aqueous solution (37%) and this mixture was added drop wise to the titanium butoxide sol under vigorous stirring. After refluxing at 60 °C for 3 h to complete the reaction, the homogeneous solution was cooled to room temperature and filtered using a 45 μm Whatman filter paper in order to remove any particulate matter possibly formed during the reaction. The solvent was evaporated at room temperature and, after complete dryness, the precipitate was finely ground in agate mortar and finally annealed at 600 °C in air for 3 h after temperature had been increased at a rate of 3 °C min−1. Photocatalyst B was prepared according to the following protocol. 34 ml of titanium butoxide was added to 30 ml of dry ethanol under stirring until a homogeneous solution was obtained. 10.5 ml of a 3% w/w ammonia aqueous solution was added drop wise to the solution of titanium butoxide and successively stirred overnight. The obtained precipitate was filtered through a 45 μm Whatman filter paper, dried in an oven at 120 °C for 3 h and finally annealed at 600 °C for 3 h after the temperature had been increased at a rate of 3 °C min−1.
Photocatalyst characterisation
The X-ray diffraction (XRD) patterns of the photocatalysts were collected on a Bruker D5000 model operating in the grazing angle mode using a Cu Kα radiation (λ = 1.5406 Å).
SEM images of the synthesised materials were acquired with a FEG-SEM (LEO 1530) at 5–10 kV beam energy.
The photodegradation of linuron in aqueous TiO2 suspensions was investigated by means of high-performance liquid chromatography (HPLC). The HPLC system was equipped with a reversed-phase column Spherisorb ODS2 (5 μm, 250 × 4.6 mm, Waters, Mildford, MA, USA), a precolumn Spherisorb ODS2 (5 μm, 10 × 4,6 mm), a 515 pump and a 996 Photodiode Array Detector (Waters). The chromatographic apparatus was controlled by Millennium32 software (Waters). Photocatalytic experiments were carried out by adding different amounts of TiO2 nanomaterials (from 5 to 40 mg) into a 100 ml pyrex glass beaker containing 30 ml of 1.0 mg l−1 aqueous solution of the pesticide. The suspension was illuminated with a couple of fluorescent lamps (Philips TL 20W/05) emitting in the wavelength range 300–400 nm with a maximum emission at 350 nm placed at a fixed distance of 20 cm from the suspension surface and was continuously stirred by means of a sonicator, Branson Model 1510 (Branson Ultrasonics Corp., Danbury, CT, USA), thermostated at 25 °C. Before introducing UV irradiation, the suspension was sonicated for 30 min in the dark in order to obtain the adsorption equilibrium. During the photodegradation experiment, 200 μl aliquots of suspension were collected at regular time intervals, filtered through a 0.45 μm Millipore filter and 20 μl of the clear solution was introduced into the chromatograph by means of a 7125 Rheodyne injector. The HPLC analysis was performed under isocratic conditions using a 30/70 v/v water-acetonitrile mobile phase flowing at 1 ml min−1, the detection wavelength being 248 nm.
Total organic carbon (TOC) was measured with a Sievers 2244 AP analytical TOC analyser (Sievers Instruments Inc., Boulder CO, USA). Anions were analysed by a Dionex ion chromatography (Dionex Corporation, Sunnyvale, CA, USA) equipped with an ED 50 conductivity detector, an anion self-generating suppressor ASRS Ultra II 2 mm, a column Ion Pac AS18 (2 mm × 250 mm), a precolumn Ion Pac AG18 (2mm × 50 mm) using a solution of NaOH (23.0 nM) flowing at 0.25 ml min−1 as the mobile phase. The fate of TOC and ionic species was investigated in an irradiated TiO2 aqueous suspension initially containing 20 mg l−1 of linuron. Solution aliquots, collected at regular time intervals after filtration, were directly analysed by ion chromatography, while TOC was analysed after a 20-fold dilution. Contribution of TiO2 impurities to TOC and ion chromatography results were evaluated by blank analyses on irradiated TiO2 suspensions in the absence of linuron and subtracted.
Results and discussion
TiO2 characterisation
The XRD patterns of TiO2 prepared following the two sol–gel methodologies are qualitatively similar and display relatively sharp diffraction peaks of the tetragonal body centered phase of anatase (Fig. 1), which is far more efficient as a photocatalyst than other natural allotropic forms (rutile and brookite).3
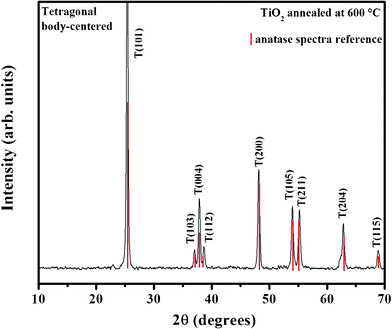 |
| Fig. 1 XRD spectrum of TiO2 prepared through protocol A and reference diffraction peaks of the anatase crystal phase. (Inserted numbers are Miller indices). | |
The SEM images at micrometre-scale magnification of the material prepared through the procedure A reveal that TiO2 consists of fragments with irregular shapes (Fig. 2a) whereas protocol B produces approximately spherical particles (Fig. 3a). However, at greater magnification both materials appear as agglomerates of crystallites with similar shape and comparable size (Fig. 2b and 3b). Based on the apparent crystallite size (within 20–40 nm), the surface area of TiO2, according to simple geometrical arguments32 is expected to fall within 30–60 m2/g. This means that the materials prepared in this work can exhibit a surface area comparable or slightly lower than that of the commercial product Degussa P-25 (50 m2 g−1)28 which is one of the most extensively investigated TiO2-based photocatalysts. Some preliminary photodegradation cycles were carried out with a catalyst to assess its stability and durability in the photocatalytic process. The catalyst was recycled after a filtration step and has been reused in five consecutive runs to determine its stability and durability. The collected data, reported in our previous report,33 show that the catalyst is stable for long time.
Blank preliminarily experiments were performed on TiO2 suspensions without UV-irradiation and on irradiated solutions but in the absence of TiO2. Both experiments were conducted under sonication in order to evaluate the potential sonolysis of the organic compound. Within the time range explored in the next photodegradation investigation, no evident change of linuron concentration was observed in either condition, which indicates that TiO2 is not able to promote linuron transformation in the dark, and the contribution of direct photolysis to degradation can be neglected.
It is established that photodegradation of organic substances in TiO2 aqueous suspensions can be described by a Langmuir–Hinshelwood kinetics model:6,34
where c is the concentration of the reactant, k the reaction rate constant, K an equilibrium adsorption constant and t, the irradiation time. For low reactant concentrations (millimolar or below) the above equation can be simplified to a pseudo-first-order kinetic equation that in the integrated form gives:
| ln (c/c0) = Kkt = kappt | (2) |
where c
0 is the starting concentration and k
app an apparent pseudo-first-order kinetic constant.
Eqn (2) accurately describes photodegradation of linuron under the various experimental conditions explored in this study, the observed correlation coefficient of ln(c/c0) data vs. irradiation time being at least 0.95.
For each photodegradation experiment kapp was evaluated by means of least-square regression as the slope of the straight line interpolating the experimental data. Typically, the observed kapp range is between 0.005 and 0.025 min−1, the related standard deviation, evaluated in duplicate experiments, being less than 0.001 min−1.
Effect of TiO2 content
Fig. 4 shows the kapp values for different amounts of TiO2 in the reaction medium. It can be observed that the phodegradation rate of linuron in both cases markedly increases with the TiO2 content in the range 0.1–0.7 g l−1, while it is almost constant or slightly decreases at higher concentrations.
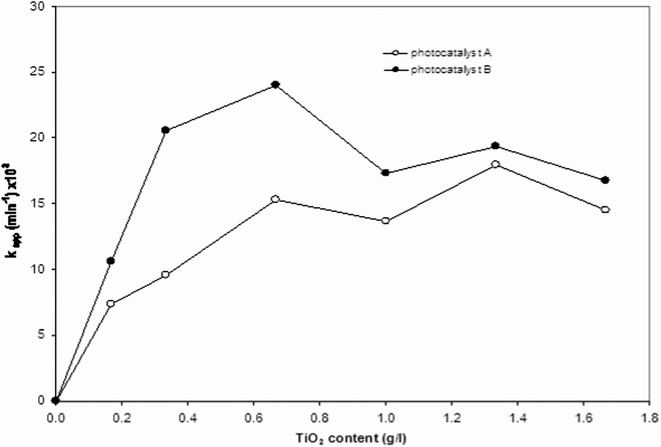 |
| Fig. 4 Observed values of the pseudo-first order kinetic constant (kapp) of linuron degradation photocatalysed by TiO2 prepared by means of procedure A (open circles) or B (full circles) as a function of the photocatalyst content. Initial linuron concentration: 1.0 mg l−1; neutral pH. | |
Typically, the increase of catalytic efficiency in dilute TiO2 suspensions is related with the increase of available reactive surface, but at higher TiO2 content further addition of photocatalyst does not result in a proportional growth of activity because of increasing opacity of the suspension and/or agglomeration phenomena.6,7 As the result of a combination of the above effects, photocatalytic activity reaches an asymptotic value or starts decreasing above the optimal TiO2 content. Observed behaviour of both materials qualitatively agrees with the expected trend, but the catalytic efficiency of material B is systematically better than that of the photocatalyst obtained with the method A within the explored range of TiO2 content, this behavior is probably due to its better dispersion in aqueous solution under sonication.
Accordingly, at the optimal TiO2 content (approximately 1.3 g l−1 and 0.7 g l−1 for materials A and B, respectively) the apparent half-life of linuron (initial concentration: 1 mg l−1) is about 40 and 30 min, respectively. Based on this evidence, further investigation was focused on the material synthesised through the procedure B.
Effect of pH
Fig. 5 displays the effect of pH on the photodegradation rate. These results reveal that photodegradation of linuron is the fastest at neutral pH (close to 6), while the disappearance rate of the herbicide decreases both at higher and lower pHs. The above evidence qualitatively agrees with the results of similar investigations carried out on other phenylureas25,31 under acidic conditions. For instance, inhibition of the photodegradation ability of TiO2 when pH is decreased in the range 2–7 was observed in the case of the parent compound fenuron.25 Decrease of pH from 2.7 to 1.6 also produced a decrease in the degradation rate of diuron.31
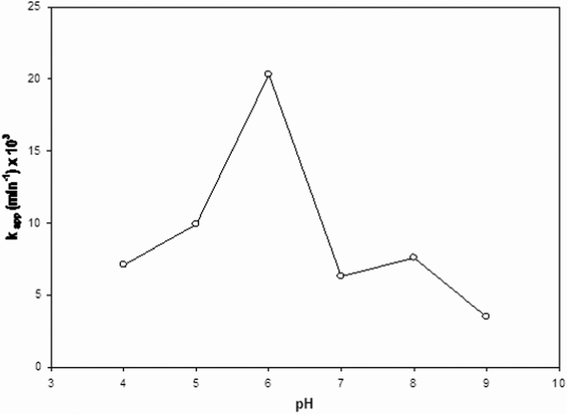 |
| Fig. 5 Observed values of the pseudo-first order kinetic constant (kapp) of linuron degradation as a function of pH. Initial linuron concentration: 1.0 mg l−1; TiO2 content: 0.3 g l−1. | |
The influence of pH on TiO2-mediated photodegradation of organic substances is a quite complex issue, since acidity of the medium influences several aspects, such as the charge state of both TiO2 and the reactant, redox properties of the photocatalyst, and agglomeration phenomena,6 these being in general interacting factors. It is widely accepted that, as a consequence of its amphoteric character, the TiO2 surface is positively or negatively charged when the pH of the medium is above or below the isoelectric pH of the photocatalyst (around 6.5), 28 respectively, which makes adsorption of ionisable reactants dependent from pH.1,6 In this connection, despite the fact that phenylurea herbicides are expected to be neutral compounds, protonation of linuron in acidic media has been experimentally observed.35 As a consequence, adsorption of linuron under very acidic conditions should be inhibited by the fact that both TiO2 and the herbicide are positively charged, which could be responsible for the lower degradation rate in acidic media. However, the above effect cannot be invoked to explain the decrease of activity when pH is decreased just below the isoelectric pH. Moreover, differences in adsorption related with pH must be excluded above the isoelectric pH, since linuron does not contain dissociable groups. It must be remarked that eqn (1) resulting from the Langmuir–Hinshelwood kinetics model, which implies an adsorption equilibrium followed by a slower single reaction step on the photocatalyst surface (the rate determining step), is actually compatible with alternative degradation mechanisms. In particular, some authors36 have shown that both steps assumed in the Langmuir–Hinshelwood mechanisms are dependent on the concentration of hydroxyl radicals. Therefore, the observed effect of pH on the degradation rate of linuron has to be ascribed to the influence of medium acidity on the formation of hydroxyl radicals rather than to its effect on the adsorption equilibrium of linuron on the photocatalyst surface.
Hydrogen peroxide is an oxidant largely used in homogeneous photodegradation processes or added to irradiated TiO2 suspensions with the aim of increasing its catalytic performance.6,8
Added to TiO2 aqueous suspensions, H2O2 can react with photogenerated electrons (eCB−) or O2−, which inhibits electron–hole recombination and, at the same time, produces ˙OH radicals, according to the following equations:
| H2O2 + eCB− → ˙OH + OH− | (3) |
| H2O2 + O2˙−→ ˙OH + OH− + O2 | (4) |
Both effects are expected to enhance the degradation rate. On the other hand, inhibition can be observed, since H2O2 acts as a scavenger of the valence band hole (hVB+) and ˙OH according to the following processes:
| H2O2 + hVB+ → O2 + 2H+ | (5) |
| H2O2 + ˙OH → H2O + HO2˙ | (6) |
Fig. 6 displays the effect of H2O2 addition up to 100 mM on the photodegradation rate of linuron, clearly revealing an inhibitory action that grows with the H2O2 content. In apparent contrast with this result, investigation of TiO2-mediated photodegradation of the phenylurea herbicides monuron16 and metobromuron26 showed that addition of H2O2 (around 10 mM) accelerates the decay of total organic carbon (TOC) content, while it did not produce an appreciable effect on TOC evolution in the case of isoproturon.26 However, it must be noted that TOC quantifies the degree of mineralization of all organic species (reactant and intermediates) while kapp is related to the disappearance rate of the reactant.
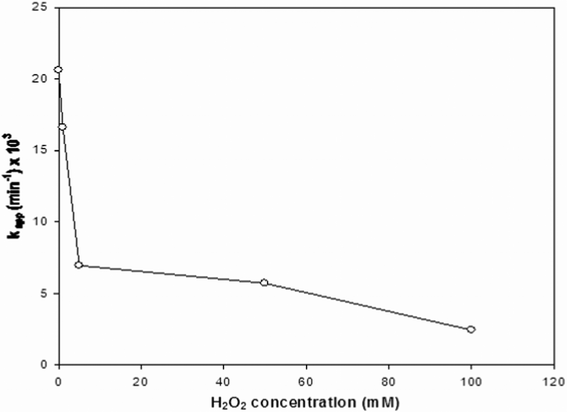 |
| Fig. 6 Observed values of the pseudo-first order kinetic constant (kapp) of linuron degradation as a function of concentration of added H2O2. Initial linuron concentration: 1.0 mg l−1; neutral pH; TiO2 content: 0.3 g l−1 | |
At this connection, several investigations suggest that rate enhancement (through eqn (3)–(4)) prevails at low H2O2 concentrations, while rate inhibition (processes 5–7) is dominant at higher H2O2 contents.6 In the presence of a higher concentration of 1.0 × 10−3 M hydrogen peroxide, it may be suggested that the hydroperoxyl radical (HO2˙) produced by the reaction between H2O2 and the OH˙ radical (6), as well as the neutral species generated between two radicals, HO2˙ and ˙OH (7), will contribute to the decrease in degradation rate. Some authors13,16 remarked on the direct role of H2O2 that when irradiated at wavelengths shorter than 300 nm generates hydroxyl radicals, widely thought as the chemical species able to initiate the degradation process, according to the equation:
As a matter of fact, homogeneous photodegradation based on the photolytical process (eqn 8), may compete with heterogeneous photocatalysis producing an apparent enhancement of catalytic efficiency in the UV/TiO2/H2O2 system.13 Since emission of the lamp used in this work at λ < 300 nm is negligible, the observed behaviour suggests that ˙OH radicals and hole quenching (processes 5–7) is the dominant effect even at low H2O2 concentration.
In order to evaluate whether the observed disappearance of linuron, as seen by HPLC analysis, is actually followed by its quantitative mineralization, TOC content was determined at different irradiation times. Moreover, since linuron contains chlorine and nitrogen atoms, Cl−, NH4+, NO2−, and NO3− are potential ionic by-products of degradation: all these inorganic species, except NH4+, were monitored by means of ion chromatography. In this regard, it is expected that NH4+ released at the beginning of the photocatalytic process is successively oxidised.29 Thus, nitrogen recovery based on observed NO2− and NO3− concentrations may be underestimated, especially at low irradiation times. The results of TOC measurements and ion chromatography analyses are collected in Fig. 7 and 8, respectively. Fig. 7 shows that TOC content decreases more slowly compared with the decreasing trend of linuron concentration.
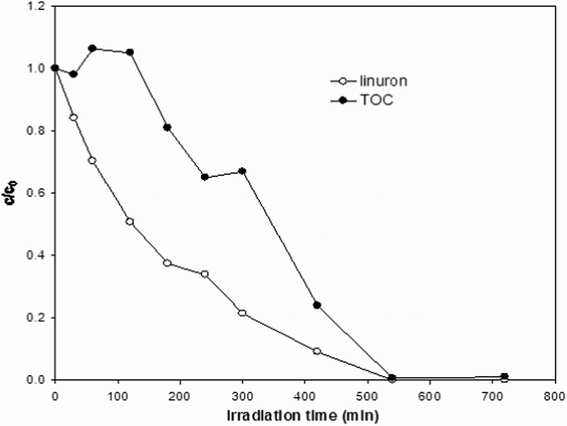 |
| Fig. 7 Kinetics of disappearance of linuron and evolution of total organic carbon (TOC) content; initial linuron concentration: 20 mg l−1; natural pH, TiO2 concentration: 1.0 g l−1. | |
Accordingly, half-life of linuron is about 150 min in these conditions, whereas within the same time range TOC is comparable to the initial value. This implies that linuron is transformed into organic intermediates that are only later mineralised, as previously shown for other phenylurea herbicides.16,24,25,28,29,31
As to the release of ionic species, it can be observed that both chloride and nitrate concentration increase monotonically, whereas nitrite concentration increases at the beginning and successively decreases, because of oxidation to nitrate. Again, the release of these anions is noticeably slower than the observed decrease of linuron concentration. According to data collected after 12 h of irradiation, recovery of nitrogen and chlorine in the ionic form is approximately 78% and 82%, respectively. Taking also into account the possible incomplete oxidation of ammonium and adsorption of ions on TiO2 that may lead to underestimation of nitrogen and chlorine recovery, the above evidence, together with the observed decrease of TOC content, suggests that TiO2-mediated photodegradation can be a suitable method for mineralization of linuron in contaminated water.
Conclusions
Although there are numerous examples of applications of photocatalysts for water treatment, there is very little information about degradation in TiO2 suspensions of the pesticide linuron, which represent one of the most important phenyl-urea herbicides. In this study are reported the syntheses of two new sol–gel TiO2 materials used for the photodegradation of a solution of linuron. We evaluate the effect of some operational parameters (TiO2 morphology, catalyst concentration, pH and H2O2 addition) on the degradation rate in aqueous solutions at mg l−1 contamination levels. The observed half-life of linuron is about 30 min in the best conditions. The almost complete mineralization of this herbicide is demonstrated by TOC measurements and high recovery of nitrogen and chlorine in the ionic form. However, the decrease of initial TOC and formation of ionic end-products is noticeably slower than the apparent disappearance of linuron, which suggests, in agreement with documented behaviour of parent compounds, a quite complex degradation mechanism involving a number of intermediates, but these are expected to eventually evolve into harmful end-products.
Acknowledgements
The authors are grateful to Mrs. Antonella Falgiani (Istituto Nazionale di Fisica Nucleare, Laboratori Nazionali del Gran Sasso) for her help in performing TOC and ion chromatography analyses and Prof. Enrico Bellotti and Dr Raffaele Adinolfi Falcone for helpful discussion. This article is dedicated to the memory of the University of L'Aquila students that died during the 6th April 2009 earthquake.
References
- M. R. Hoffmann, S. T. Martin, W. Choi and D. W. Bahnemann, Chem. Rev., 1995, 95, 69 CrossRef CAS.
-
N. Serpone, E. Pelizzetti, Photocatalysis-Fundamentals and Applications, Wiley-Interscience, New York, 1989 Search PubMed.
- A. Mills, R. H. Davies and D. Worsley, Chem. Soc. Rev., 1993, 22, 417 RSC.
- A. L. Linsebigler, G. Lu and J. T. Yates Jr., Chem. Rev., 1995, 95, 735 CrossRef CAS.
- N. Serpone, D. Lawless, R. Khairutdinov and E. Pelizzetti, J. Phys. Chem., 1995, 99, 16655 CrossRef CAS.
- E. Evgenidou, I. Konstantinou, F. Konstantinos, I. Poulios and T. A. Albanis, Catal. Today, 2007, 124, 156 CrossRef CAS.
- J.-M. Herrmann, Catal. Today, 1999, 53, 115 CrossRef CAS.
- D. F. Ollis, E. Pelizzetti and N. Serpone, Environ. Sci. Technol., 1991, 25, 1523 CrossRef.
- I. K. Konstantinou and T. A. Albanis, Appl. Catal., B, 2003, 42, 319 CrossRef CAS.
- C. Aprile, A. Corma and H. Garcia, Phys. Chem. Chem. Phys., 2008, 10, 769 RSC.
- J. Cunningham, G. Al-Sayyed, P. Sedlak and J. Caffrey, Catal. Today, 1999, 53, 145 CrossRef CAS.
- X. Chen and S. S. Mao, Chem. Rev., 2007, 107, 2891 CrossRef CAS.
- Y. Wang and C.-S. Hong, Water Res., 1999, 33, 2031 CrossRef CAS.
- B. Sun, E. P. Reddy and P. G. Smirniotis, J. Catal., 2006, 237, 314 CrossRef CAS.
- C. Chen, X. Li, W. Ma, J. Zhao, H. Hidaka and N. Serpone, J. Phys. Chem. B, 2002, 106, 5022 CrossRef.
- M. Bobu, S. Wilson, T. Greibrokk, E. Lundanes and I. Siminiceanu, Chemosphere, 2006, 63, 1718 CrossRef CAS.
-
C. D. S. Tomlin, The Pesticide Manual, 11th ed., The British Crop Protection Council, Surrey, U.K., 1997 Search PubMed.
-
N. N. Ragsdale, R. E. Menzer, Carcinogenicity and Pesticides: Principles, Issues, and Relationships. ACS, Washington, DC, 1989 Search PubMed.
- V. I. Boti, V. A. Sakkas and T. A. Albanis, J. Chromatogr., A, 2007, 1146, 139 CrossRef CAS.
- F. J. Benitez, F. J. Real, J.L. Acero and C. Garcia, J. Hazard. Mater., 2006, 138, 278 CrossRef CAS.
- V. H. Katsumata, S. Kaneco, T. Suzuki, K. Ohta and Y. Yobiko, Chem. Eng. J., 2005, 108, 269 CrossRef.
- R. C. Martins, A. F. Rossi and R. M. Quinta-Ferreira, J. Hazard. Mater., 2010, 180, 716 CrossRef CAS.
- M. J. Farré, S. Brosillon, X. Doménech and J. Peral, J. Photochem. Photobiol., A, 2007, 189, 364 CrossRef.
- L. Lhomme, S. Brosillon, D. Wolbert and J. Dussaud, Appl. Catal., B, 2005, 61, 227 CrossRef CAS.
- C. Richard and S. Bengana, Chemosphere, 1996, 33, 635 CrossRef CAS.
- S. Parra, J. Olivero and C. Pulgarin, Appl. Catal., B, 2002, 36, 75 CrossRef CAS.
- A. E. Kinkennon, D. B. Green and B. Hutchinson, Chemosphere, 1995, 31, 3663 CrossRef CAS.
- A. Amine-Khodja, A. Boulkamh and C. Richard, Appl. Catal., B, 2005, 59, 147 CrossRef CAS.
- E. Pramauro, M. Vincenti, V. Augugliaro and L. Palmisano, Environ. Sci. Technol., 1993, 27, 1790 CrossRef CAS.
- S. Parra, V. Sarria, S. Malato, P. Péringer and C. Pulgarin, Appl. Catal., B, 2000, 27, 153 CrossRef CAS.
- K. Macounová, H. Krýsová, J. Ludvík and J. Jirkovský, J. Photochem. Photobiol., A, 2003, 156, 273 CrossRef.
- D. W. Bahnemann, S. N. Kholuiskaya, R. Dillert, A. I. Kulak and A. I. Kokorin, Appl. Catal., B, 2002, 36, 161 CrossRef CAS.
-
F. Ruggieri, A.A. D'Archivio, M. Fanelli, P. Mazzeo, A.R. Phani, S. Santucci, Degradation of organic molecules by photocatalytic action of TiO2. Presented at the 25th conference TUMA, Camerino, Italy, 29 June–1 July, 2006; Search PubMed.
- H. Al-Ekabi and N. Serpone, J. Phys. Chem., 1988, 92, 5726 CrossRef CAS.
- M. J. González de la Huebra, P. Hernández, Y. Ballesteros and L. Hernández, Talanta, 2001, 54, 1077 CrossRef.
- D. F. Ollis, J. Phys. Chem. B, 2005, 109, 2439 CrossRef CAS.
|
This journal is © The Royal Society of Chemistry 2011 |
Click here to see how this site uses Cookies. View our privacy policy here.