DOI:
10.1039/C1RA00023C
(Paper)
RSC Adv., 2011,
1, 238-246
The flow of magnetic nanoparticles in magnetic drug targeting
Received
8th April 2011
, Accepted 19th April 2011
First published on 1st August 2011
Abstract
Magnetic drug targeting has been explored by an in vitro study of the deposition of polyvinyl alcohol (PVA) coated magnetic carrier nanoparticles (MCNPs) in a tube under the influence of an externally applied magnetic field. Experiments and simulations show a steady decrease in the retention of MCNPs with increasing flow rate and weaker magnetic field strength. The retention of MCNPs has been significantly influenced by the fluid flow behaviour resulting from the position and shape of the magnet, magnetic properties and size of the MCNPs, and the magnetic field strength. Under strong magnetic fields, the MCNPs tend to creep along the wall of the tube and undergo high shear before reaching the targeted region. These results highlight the importance of choosing the region of MCNP injection, magnetic field strength and, the magnetic properties and size of the MCNPs to minimize the loss of the drug.
1. Introduction
Recent technological advances in the synthesis and characterization of magnetic nanoparticles has opened up a host of applications in bioengineering.1,2 In particular, magnetic nanoparticles are of immense importance in the context of the targeted delivery of drugs because the movement of these particles can be controlled by an external magnetic field. In cancer treatments, surgery results in numerous complications, radiotherapy destroys healthy as well as cancerous cells, and chemotherapy is limited by systemic toxicity due to the relatively nonspecific action of cytotoxic drugs.3,4 Targeted drug delivery at the tumor by an external magnetic field shows great promise to maximize the concentration of therapeutic drugs at the desired location and minimize the side effect.5–9
Recent studies show that an externally applied magnetic field can trap drug loaded polymer coated magnetic carrier nanoparticles (MCNPs) at targeted locations.10–14 In this method, a suspension of MCNPs is injected into a blood vessel near the tumor.15 An external magnetic field is applied to localize the MCNPs at the targeted region, maximizing drug concentration at the targeted region and minimizing systemic toxicity and wastage of drugs.16 The capture of MCNPs loaded with anti-cancer drug has been primarily investigated for surface tumors7,16 and in animal models.10,17–33 Related magnetic hyperthermia,34–38 and radiation therapies39,40 have also been studied. Clinical trials have shown encouraging results, e.g., remission of squamous cell carcinoma in white rabbits.16 A phase-I human clinical trial with 4-epidoxorubicin has shown encouraging results of the physiological tolerance of magnetic drug targeting in patients.15
The effectiveness of this technique is critically dependent on MCNPs with superior properties.41–43 The flow behaviour of such MCNPs in the blood stream is the key to effective magnetic drug targeting.44 Micron sized magnetic particles have been deposited in a capillary bed of a blood vessel inside a tumor by placing an external magnetic field ∼10 cm away from the tumor location.45,46 Other techniques such as implant assisted magnetic drug targeting (IA-MDT) employing wires,47–51 seeds,52,53 and stents54–56 inside the body to enhance magnetic field strength have also been reported. Parametric analyses of the transport and deposition of magnetic carriers in the microvasculature have been performed.57–58 The flow rate of the blood, the magnetic and hydrodynamic properties of the MCNPs are very important parameters, which can significantly influence MCNP deposition at the targeted region.
Hence, we investigate the influence of flow behaviour on the deposition of MCNP at the targeted location. We emphasise the importance of choosing the optimal magnetic field strength, shape of the magnet, size and magnetic properties of the MCNPs, and location of MCNP injection needed to improve the efficiency of the magnetic drug targeting. A cylindrical tube is employed to mimic a blood vessel and MCNPs are injected as a suspension in buffer solution flowing inside the tube. Fig. 1 shows the schematic diagram of the experimental set-up. An external magnetic field oriented perpendicular to the flow direction is applied and the deposition efficiency of MCNPs at the targeted region is determined. The retention of MCNPs is found to depend on the magnetic field strength, size and magnetic properties of the MCNPs, and the associated flow characteristics of the fluid. To reveal the salient features of the MCNP deposition mechanism, time dependent computational fluid dynamic (CFD) simulations are performed. The simulations suggest interesting mechanisms of magnetic trapping of the MCNPs. For example, (i) for a strong magnetic field, MCNPs can undergo high shear forces as they tend to creep along the tube wall; (ii) the deposition pattern of the MCNPs at the targeted region varies with magnet shape; (iii) the MCNP deposition is found to be less at the targeted location and an increased loss is observed when the flow rate is high or under a weaker magnetic field; (iv) the flow behaviour of the MCNP containing fluid is significantly influenced by the size and magnetic properties of the MCNPs. It is important to note that the magnet shape, the magnetic field strength, and the size and magnetic properties of the MCNPs in the fluid have different temporal responses to the applied magnetic field. This study demonstrates a collection of consequences for a range of process parameters, which essentially highlights the importance of quantity of MCNP loading, optimal tuning of magnetic field strength, location of MCNP injection, flow rate of carrier fluid, size and magnetic properties of the MCNPs. The fluid dynamic aspects of the magnetic drug delivery discussed in this work can be used to improve the efficiency of drug targeting by increasing the MCNP concentration at the targeted region.
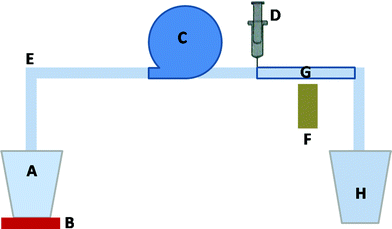 |
| Fig. 1 The schematic of in vitro experiment for magnetic drug targeting: A—intake beaker, B—vibrating plate, C—pump, D—syringe, E—silicone tube, F—permanent magnet, H—outlet beaker, G—region of targeting. | |
2. Experimental procedure
Fig. 1 shows a schematic diagram of the in vitro experimental set-up to mimic blood circulation through a single blood vessel. It consists of an intake beaker (A), pump (C), vibrating plate (B), MCNP suspension dosing unit (D), permanent magnet (F) and the targeted region (G).
The MCNPs were synthesized and then deposition efficiency of the MCNPs was determined. To synthesize iron oxide nanoparticles,41 a mixture of Fe2+ and Fe3+ ions (molar ratio 1
:
2) was prepared by dissolving 0.15 mol of FeCl2·4H2O and 0.3 mol of FeCl3·6H2O in 30 cc deionized water. This mixture was stirred at 600 rpm, mixed with 100 cc of 0.4 M NaOH (pH ∼ 13) to precipitate iron oxide nanoparticles. These nanoparticles were then added to polyvinyl alcohol (PVA) solution and stirred for 12 h. This PVA solution was prepared by adding dry PVA powder in deionized water at 55 °C. Finally, PVA coated iron oxide MCNPs were separated by centrifugation and dried in a vacuum oven.
In the in vitro set-up, (Fig. 1), PBS buffer, which has physiological properties similar to blood, was driven by a pump (C) through a tube (E) with an inner diameter of 1 mm and length of 100 mm. We term the buffer solution as the carrier fluid. The flow rate was altered by adjusting the pump throughput. A suspension of PVA coated iron oxide MCNPs (0.33 mg cc−1) was injected at the tube inlet. A Nd–Fe–B rectangular permanent magnet (F) (100 mm × 50 mm × 20 mm, energy product: 40 MG Oe) was placed laterally outside the tube and the magnetic field strength was changed by adjusting the vertical distance (d) of the magnet from the tube. The MCNPs deposited on the inner wall of the tube were collected in acetone and dried in an oven to remove the acetone. The percentage retention of MCNPs is calculated from the ratio of the mass of the deposited MCNPs relative to the mass of the MCNPs injected into the inlet.
3. Modeling
The high aspect ratio cylinder employed in the experiments was approximated as a two-dimensional (2-D) rectangular channel in the CFD simulations. The simplified geometry helps in quick implementation of the magneto-hydrodynamic models in the formulation and also provides us with a first order estimate on the mechanism of deposition in the initial stages. Fig. 2 shows the geometry for the simulations, x and y denote horizontal and vertical coordinates, the magnet is placed midway along the x-axis. We assumed the liquid medium to be Newtonian and incompressible because PBS buffer (a water based chloride, phosphate salt solution of Na and K) shows characteristics similar to water at room temperature and atmospheric pressure. Gravity was neglected due to small size of the MCNP and the effect of gravity is negligible compared to the stronger body force from the external magnetic field. The low flow rates ensured low Reynolds number laminar flow. The dimensions of the magnet as well as the flow properties of the fluid and nanoparticles used for the simulations are provided in Table 1.
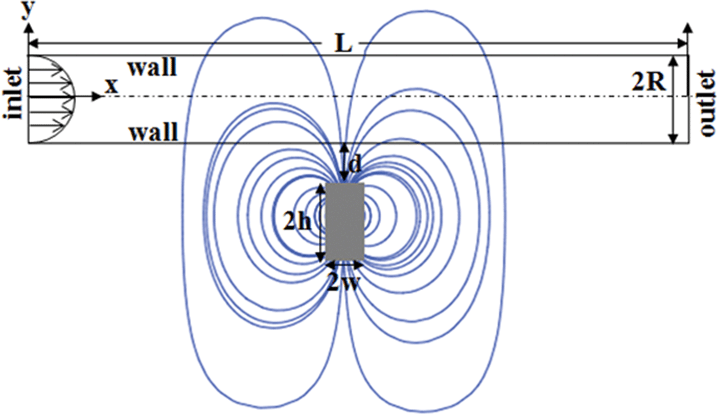 |
| Fig. 2 The 2-D rectangular channel which mimics the experimental cylindrical tube with the frame of reference and variables used for the numerical analysis. | |
Table 1 Parameters used in the modeling
Properties |
Symbol |
Units |
Value |
Width of rectangular magnet |
2w |
mm |
50 |
Height of rectangular magnet |
2h |
mm |
100 |
Diameter of cylindrical magnet |
2r |
mm |
60 |
Magnetization of Nd-Fe-B magnet |
M
s
|
A m−1 |
106 |
Length of the channel |
L
|
mm |
100 |
Height of the channel |
2R |
mm |
1 |
Particle size |
r
p
|
nm |
100, 200 |
Susceptibility of magnetic particle |
x
|
|
0.4, 0.9 |
Flow rate of fluid |
v
|
mm s−1 |
1.5, 5, 10, 40 |
Density of ferrofluid |
ρ
|
kg m−3 |
800 |
Viscosity of ferrofluid |
μ
|
N s m−2 |
1.0 × 10−3 |
Permeability of vacuum |
μ
0
|
T m A−1 |
4π × 10−7 |
A. Governing equations
The Navier–Stokes equations of motion with the equation of continuity were employed to describe the motion of the buffer flowing inside the channel (Fig. 2):59 | 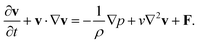 | (2) |
Here v, p, μ, ρ, v (= μ/ρ) and F denote the velocity vector, pressure, viscosity, density, kinematic viscosity and the external body force due to the applied magnetic field, respectively. We assumed the continuum approximation to be valid because the sparse suspension of MCNPs can undergo continuous motion within the length and time scales of the flow. Thus, the buffer solution with MCNP suspension was modeled by a multiple species balance convection–diffusion equation across the control volume:59 |  | (3) |
Here Y, vp and D are the mass fraction of MCNPs, the velocity of MCNPs and mass diffusivity, respectively. The equations of motion, continuity equation and species balance equation were solved using the software ANSYS FLUENT™. To calculate the μ and ρ of the mixture, the mass and volume weighted mixing law was used. For the mass diffusivity constant, the dilute-approximation with constant diffusion coefficient was employed. The properties of each species used for the simulations are supplied in Table 1. The velocity of MCNPs (vmag) in the buffer solution was obtained by balancing the hydrodynamic drag forces and magnetic forces, vmag = Fmag/6πμrp, where rp is the radius of an MCNP. In this analysis, the hydrodynamic diameter of the cluster of particles in suspension was obtained from dynamic light scattering (DLS) (Table 1). However, we reported the individual particle size (10 to 15 nm) which exhibited superparamagnetic character.60 The total velocity vp of MCNPs in the species balance (eqn (3)) is vp = v + vmag.44
B. Magnetic force field
The magnetic field of the permanent magnet was modeled as a source term to the momentum equation. In this study, magnets with rectangular and cylindrical cross section were studied to evaluate the influence of magnet shape on the deposition of MCNPs. The magnetic force per unit volume was assumed to be F = nFmag, where n is the number of MCNPs in the buffer solution. The magnetic force acting on each particle is given by:44Here μ0 is the magnetic permeability of free space, V is the volume of magnetic particle, χ is the magnetic susceptibility, and H is the magnetic field strength. The analytical expressions obtained for the x- and y-components of the magnetic field strength for a rectangular magnet are:61 |  | (5) |
|  | (6) |
The width and the height of the rectangular magnet are denoted by 2w and 2h, respectively, d is the distance between the surface of the magnet and the bottom wall of tube, R is the half-width of the channel (Fig. 2). The analytical expressions for the x- and y-components of the magnetic field strength for a cylindrical magnet with radius Rmag is given by:61 | 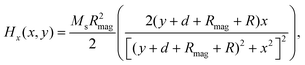 | (7) |
| 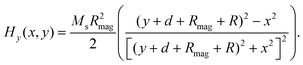 | (8) |
Fig. 3 shows the variation in ∇Hx2 and ∇Hy2 across the dimensionless length (x/L) of the tube. The curves in Fig. 3a and 3b are the profiles for rectangular and cylindrical magnets, respectively. The ∇Hx2 plots [curves (i)–(iii)] suggest that upstream the magnitude of the force field (|∇Hx2|) gradually increases but downstream it progressively decreases. The y-component field ∇Hy2 [curves (iv)–(vi)] creates a gradient of magnetic force in the −y direction, which moves the particles towards the magnet. These curves also show that ∇Hy2 decays sharply away from the magnet towards the inlet and outlet of the tube. For the rectangular magnet [curves (iv)–(vi) in Fig. 3a] the force component is maximum near the corners of the rectangular magnet. In contrast, ∇Hy2 is maximum at the centre of the cylindrical magnet [curves (iv)–(vi) in Fig. 3b] and decays sharply away from the magnet. The plots also show that the magnitude of ∇Hx2 and ∇Hy2 decreases if the distance between the magnet and tube increases. The results obtained from the analytical expressions (eqn (5)–(8)) were also validated with the components of the magnetic field strength (H) of the corresponding permanent magnet, by numerically solving the magnetostatic equations in COMSOL multiphysics™ commercial software, | 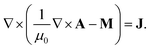 | (9) |
Eqn (9) is obtained by combining the Maxwell–Ampere equation (∇ × H = J), the Gauss equation (∇·B = 0), the constitutive equation [B = μ0(M + H)], and the equation for magnetic vector potential (B = ∇ × A and ∇·A = 0). Here J, B, M and A denote current density, magnetic flux density, magnetization, and magnetic vector potential, respectively. The magnetic insulation condition Az = 0 is employed at the boundaries to solve eqn (9).
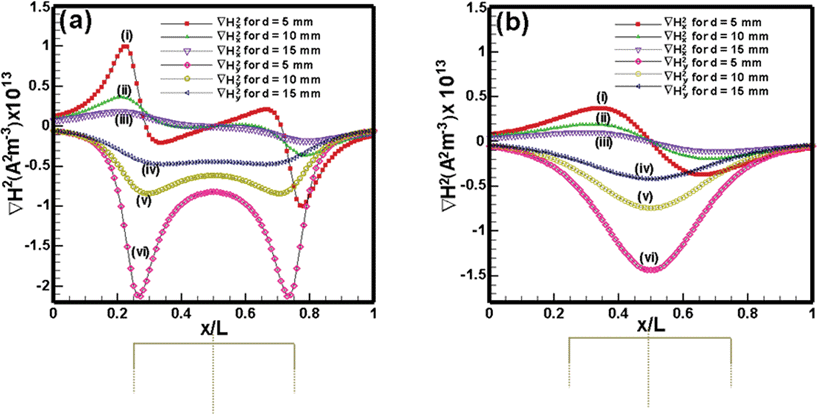 |
| Fig. 3 Variation of ∇H2 components along the x direction (x/L): (a) Nd–Fe–B rectangular permanent magnet and (b) Nd–Fe–B cylindrical permanent magnet along the axis of the tube, when the tube is at a distance of 5 mm, 10 mm and 15 mm from the surface of the magnet. | |
C. Boundary conditions
The walls in the geometries were assumed to be no-slip and impermeable:The inlet flow was modelled as a fully developed parabolic profile [vx = 2
(1 − (x/R)2)] where the average velocity (
) was incorporated from the range of flow rates used in the experiments (1.5 mm s−1 to 40 mm s−1). The typical inlet MCNP concentration (0.33 mg cc−1) was also obtained from experiments. At the channel exit, the pressure outlet boundary condition was enforced.
D. Grid generation and solution methodology
The grid was prepared using the commercial software GAMBIT. The typical grid size is around 105cells. The equations of motion, continuity equation, and species balance equation together with the boundary conditions were solved employing the commercial software ANSYS FLUENT™. Fluent uses a control volume based technique to convert the governing equations to algebraic form, which are then solved numerically. The typical time steps were 10−5 to 10−4 s. This control volume technique consists of integrating the governing equations about each control volume, yielding discrete equations that conserve each quantity on a control-volume basis. The co-located scheme used by the ANSYS FLUENT solver stores both pressure and velocity at cell centers. In this study, we used the default ‘standard’ interpolation scheme which interpolates the pressure values at the faces using momentum equation coefficients. The momentum and species equations were solved employing a ‘first order upwind’ discretization scheme. We employed the unsteady solver for time marching over a period and the results obtained were found to be grid independent. Selected simulations reported earlier44 were reproduced to validate the computational protocol.
4. Results and discussion
The success of magnetic field assisted drug delivery depends on the extent to which MCNPs can be deposited at the targeted region. The following factors can significantly influence the efficiency: (i) distance of the injection point of the MCNPs from the targeted region, (ii) size and magnetic properties of the MCNPs, (iii) magnetic field strength, and (iv) flow rates of the fluid carrying the MCNPs. We first discuss experimental results of in vitro deposition of MCNPs for various flow parameters and magnetic field strength values. Thereafter, we explore the finer flow features of MCNP deposition using nonlinear CFD simulations.
A.
In vitro experimental deposition of PVA coated iron oxide MCNPs
Fig. 4 shows the variation of percentage retention of MCNP with changing flow rates of the carrier fluid. The figure also depicts the influence of the size of MCNP and magnetic field gradient on the percentage retention. The range of typical flow rates inside a capillary bed of a tumor is 1.5 mm s−1 to 10 mm s−1.62 Plots (a) to (d) show that the deposition of MCNPs at the targeted region reduces as the flow rate of fluid increases. Increasing the flow rate of the carrier fluid induces more convective force and carries the MCNPs away from the target zone. Plots (a) and (c) show that when the size of the MCNPs is larger a stronger body force is experienced by the MCNP leading to higher retention of the MCNPs. Plots (b) to (d) indicate that a larger magnetic field gradient for MCNPs with similar size leads to higher deposition. Interestingly, plots (a) and (b) demonstrate that even when the magnetic field gradient is high, the percentage retention can be low if the size of the MCNPs is small. The experiments confirm that the size of the MCNPs, the magnetic field and the hydrodynamics of the carrier fluid are very important parameters for magnetic drug targeting.
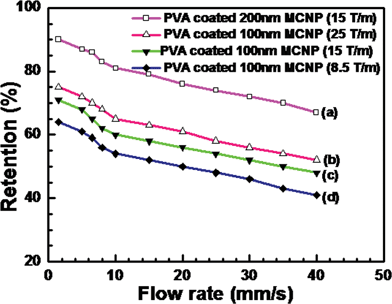 |
| Fig. 4
Retention of PVA coated Fe3O4 MCNPs at various flow rates of fluid with different magnetic field gradients. | |
B. Nonlinear CFD simulations of MCNP deposition
We carried out a series of nonlinear simulations to model the flow features of the MCNP loaded carrier fluid under various conditions. The inlet flow is on the left hand side and the outlet is on the right hand side. The magnetic field is applied at the bottom of the channel similar to the experimental set-up. For the sake of brevity, we term the carrier fluid loaded with MCNPs as the ferrofluid in the following section.
The contours in Fig. 5 show the spatiotemporal evolution of ferrofluid inside the tube when the inlet average velocity of fluid (
) is 10 mm s−1 and the magnet is placed d = 10 mm away from the tube wall. Frame (i) shows that the ferrofluid enters through the inlet and the MCNPs move towards the lower wall under the influence of the y-component of the applied magnetic force. Thereafter, the x-component of the magnetic field forces the MCNP to creep along the bottom wall of the tube towards the targeted region [Frames (i) and (ii)]. As the MCNP reach the centre of the tube, they are exposed to the weakest x-component and strongest y-component of the magnetic force gradient. Frame (iii) shows that with time the MCNPs gradually start depositing near the targeted zone. Frames (iv) to (vii) show that as more MCNPs are deposited near the magnet, diffusive and convective forces spread the MCNP in the vertical and lateral directions of the tube, respectively. With increasing time, convective forces play an important role in reducing the deposition of MCNPs at the targeted region by causing them to flow downstream. Thus, the time of application of the magnetic field must be carefully chosen to maximize deposition of MCNPs. Frames (iv) to (vii) also show that maximum MCNP deposition takes place near the corners of the magnet where the y-component of the magnetic force is strongest. The MCNPs are subjected to high wall shear upstream, which can lead to erosion of the PVA coating of the MCNPs before it reaches the targeted region. Hence special care is necessary to protect drugs from friction/adsorption with the walls before they reach the targeted location. Fig. 5 also shows that the quantity of MCNPs is crucial because after a certain exposure time of the magnetic field diffusive and convective losses can reduce the quantity of MCNPs.
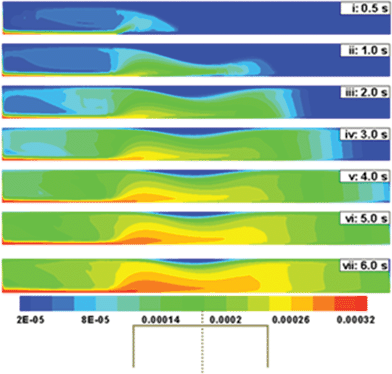 |
| Fig. 5 Temporal evolution of the concentration profile when a ferrofluid (rp = 100 nm and x = 0.4) is continuously injected at the inlet of a 2-D channel. Initially the tube is assumed to be filled with a PBS buffer flowing at 10 mm s−1. The centre of the magnet is placed at the midpoint of the lateral coordinate. In this simulation d is kept constant at 10 mm. | |
Fig. 6 shows a comparison of the deposition when
is varied from 1.5 mm s−1 to 40 mm s−1 and d is kept constant at 10 mm. Fig. 6a to 6c show that the mechanism of deposition is similar to that in Fig. 5, however, the time required to deposit the MCNPs changes as
is varied. For example, Fig. 6a shows that when
= 1.5 mm s−1, deposition near the magnet at 15 s is similar to the amount of deposition after 3 s when
= 10 mm s−1 [Fig. 5, frame (iv)] and deposition after 5 s when
= 5 mm s−1 [Fig. 6b, frame (vi)]. As the flow rate is reduced, the deposition increases, due to reduced loss of MCNPs through the outlet. These observations from the simulations are in accordance with the experimental results (Fig. 4). Fig. 5 and 6 suggest that the time of exposure to the magnetic field can be optimized once the diameter of the blood vessel and blood flow rate are known. For example, Fig. 6c shows that when
= 40 mm s−1 and d = 10 mm, 0.5 s to 1.0 s exposure leads to significant deposition. Thereafter, dispersion of particles along and across the vessel leads to loss of MCNPs from the targeted region.
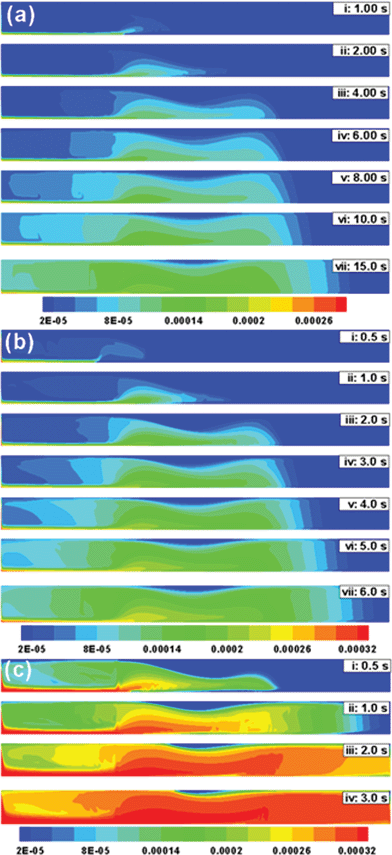 |
| Fig. 6 Temporal evolution of the concentration profile when a ferrofluid (rp = 100 nm and x = 0.4) is continuously injected at the inlet of a 2-D channel. In this simulation d is kept constant at 10 mm. The contour plots represent: (a) = 1.5 mm s−1, (b) = 5 mm s−1 and (c) = 40 mm s−1. | |
Other than the flow rate, the magnetic field is an important parameter to optimize MCNP deposition at the target zone. Interestingly, the flow behaviour of the MCNPs changes if the particle size (rp), magnetic susceptibility (x), and the magnet distance (d) from the tube are changed. Fig. 7 summarizes the effect of these parameters in magnetic drug targeting. When
= 10 mm s−1 and d = 5 mm, the rectangular magnet results in higher deposition of MCNPs near the corners (Fig. 7a), since the y-component of the magnetic field is highest at the corners for a rectangular magnet (Fig. 3a). However, a weaker magnetic field (e.g.,
= 10 mm s−1 and d = 15 mm) leads to early dispersion of MCNPs and their loss from the outlet since convective forces dominate over the weaker magnetic forces (Fig. 7b). Fig. 7c shows high deposition near the magnet within 1.0 s, for larger MCNPs (200 nm) when
= 10 mm s−1 and d = 10 mm. When the particle size is smaller (100 nm), similar deposition occurs after 2 s (Fig. 5). Fig. 7d shows that by increasing the magnetic susceptibility (x = 0.9), faster deposition can be achieved as the magnetic force is increased. Thus the spatiotemporal deposition pattern varies significantly as the size and magnetic properties of MCNPs as well as the magnetic field strength are changed.
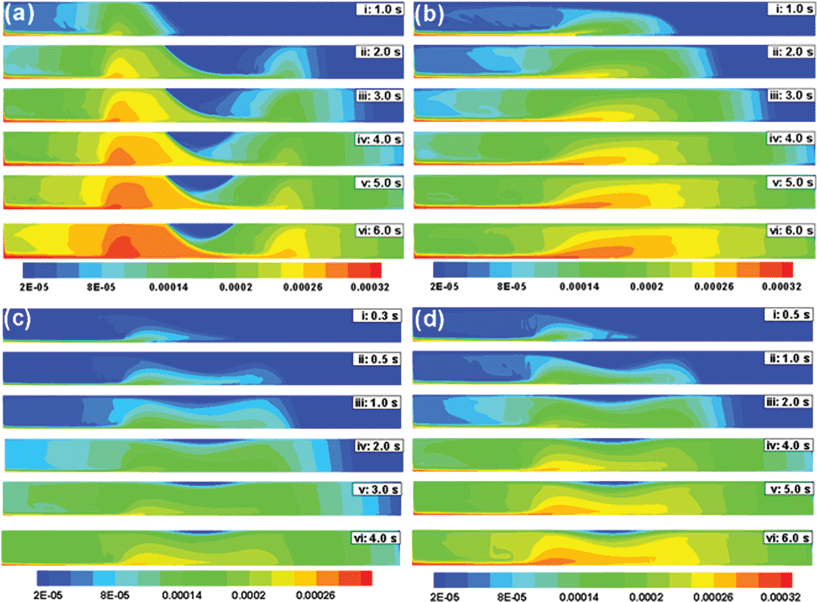 |
| Fig. 7 Temporal evolution of the concentration profile when a ferrofluid ( = 10 mm s−1) is continuously injected at the inlet of a 2-D channel. In this contour plots: (a) d = 5 mm, rp = 100 nm and x = 0.4; (b) d = 15 mm, rp = 100 nm and x = 0.4; (c) d = 10 mm, rp = 200 nm and x = 0.4; (d) d = 10 mm, rp = 100 nm and x = 0.9. | |
Interestingly, lower magnetic field strength can also lead to changes in the flow mechanism (Fig. 8). Fig. 8a shows the temporal evolution of ferrofluid when
= 40 mm s−1 and d = 50 mm. Frame (i) shows that initially the MCNPs move towards the bottom wall. However, convective forces take over quickly and the MCNPs dislodge from the bottom wall to flow through the central portion of the tube. The streamlines in the magnified view (Fig. 8b) indicate that competition between the magnetic and convective forces leads to the formation of vortices near the wall, which impede the movement of the MCNPs towards the wall. Frames (ii) to (vi) (Fig. 8a) show that after longer time although the weak magnetic field directs some fraction of the MCNPs towards the lower wall upstream, most of the MCNPs pass through the central part of the tube and go downstream. The flow downstream is similar to the pipe-flow in a 2-D channel in the absence of a magnetic field.
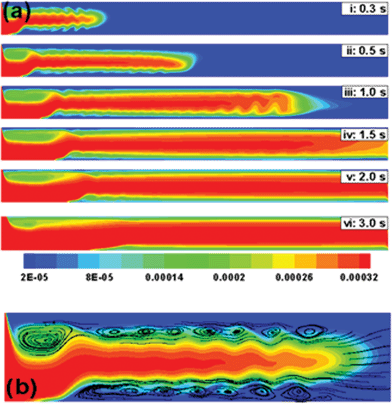 |
| Fig. 8 (a) Temporal evolution of the concentration profile when a ferrofluid (rp = 100 nm and x = 0.4) is continuously injected at the inlet of a 2-D channel at flow rate ( ) of 40 mm s−1 under weak magnetic field (d = 50 mm). (b) The streamline contour plot of frame (i) in magnified view shows the trade off between the magnetic and convective effects leading to the formation of vortices near the wall. | |
Fig. 9 shows the effect of magnet shape, the magnet has a cylindrical cross section and the deposition is concentrated near the centre of the magnet. Fig. 9a to 9c show the spatiotemporal evolution of ferrofluid in the tube when d = 10 mm and flow rate is varied (
= 1.5 mm s−1, 10 mm s−1, and 40 mm s−1, respectively). Deposition is concentrated near the centre of the magnet (Fig. 9a) since the magnetic force is at a maximum at the centre of a cylindrical magnet (Fig. 3b). This is in contrast to a rectangular magnet, where the magnetic force is at a maximum at the corners of the magnet. However, changes of deposition with changes in flow rate are similar to the case of a rectangular magnet.
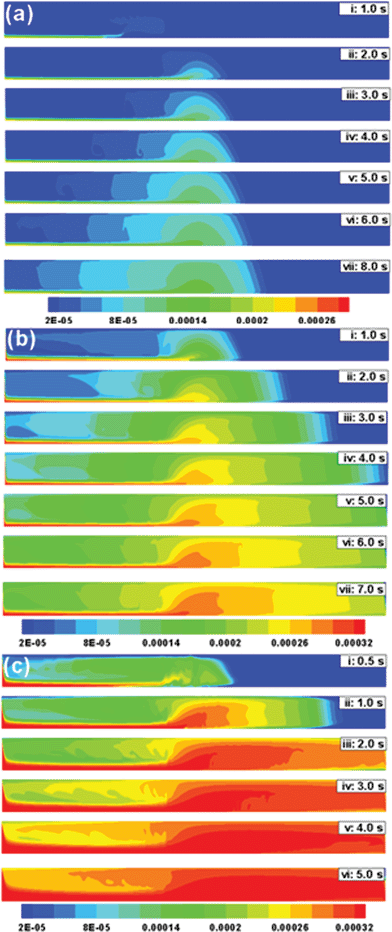 |
| Fig. 9 Temporal evolution of the concentration profile of a ferrofluid (rp = 100 nm and x = 0.4) in a 2-D channel under the influence of a cylindrical magnet (d = 10 mm). The contour plots represent: (a) = 1.5 mm s−1, (b) = 10 mm s−1 and (c) = 40 mm s−1. | |
5. Conclusions
A combined experimental and theoretical study of magnetically targeted drug delivery was conducted. The major conclusions are:
(i) Experimentally obtained deposition efficiency and its variation with the flow rate of the fluid are qualitatively consistent with results obtained from the CFD simulations. The retention of MCNPs reduces with an increase in the flow rate of the carrier fluid, a reduction in magnetic field strength, and a reduction in the size and magnetic susceptibility of the MCNPs. For a given magnetic field strength, the time of exposure to the magnetic field can be optimized once the diameter of the tube and flow rate of carrier fluid is known. A lower flow rate increases the time required for magnetic field exposure, a higher flow rate can lead to a larger loss of MCNPs by diffusion and convection losses. The amount of drug needs to be optimized to account for these losses.
(ii) The magnetic field strength, magnet shape, size and magnetic properties of the MCNPs significantly influence the deposition of MCNPs. Weaker magnetic fields lead to early dispersion of MCNPs and their loss from the outlet since the convective effect dominates over the magnetic effect. High deposition is found near the magnet within short times for larger MCNPs and higher magnetic susceptibility. Comparison between cylindrical and rectangular magnets infers that cylindrical magnets lead to smoother deposition at the targeted zone.
(iii) The MCNPs creep along the bottom wall of the tube under the influence of a strong magnetic field. The resulting shear forces can erode the polymer shell resulting in drug loss. Thus, MCNP injection in close proximity to the tumor is preferable.
(iv) For very weak magnetic fields, creep flow is absent and the MCNPs flow through the central portion of the tube. When the MCNPs loaded in the ferrofluid dislodge from the lower wall, the vortices generated near the wall boundary hinder the movement of MCNPs back to the targeted region.
In summary, this study demonstrates the effect of blood flow rate, magnet shape, size and magnetic properties of MCNPs and magnetic field strength on the deposition efficiency, which is a critical factor in optimizing magnetically targeted drug delivery.
References
- R. Jurgons, C. Seliger, A. Hilpert, L. Trahms, S. Odenbach and C. Alexiou, J. Phys.: Condens. Matter, 2006, 18, S2893 CrossRef CAS.
- V. P. Torchilin, Eur. J. Pharm. Sci., 2000, 11, S81 CrossRef CAS.
- A. S. Lubbe, C. Alexiou and C. Bergemann, J. Surg. Res., 2001, 95, 200 CrossRef CAS.
- J. M. Llovet and J. Bruix, J. Hepatol., 2008, 48, S20 CrossRef CAS.
- U. O. Hafeli, Int. J. Pharm., 2004, 277, 19 CrossRef CAS.
- M. Shinkai, J. Biosci. Bioeng., 2002, 94, 606 CAS.
- C. C. Berry and A. S. G. Curtis, J. Phys. D: Appl. Phys., 2003, 36, R198 CrossRef CAS.
- T. Neuberger, B. Schopf, H. Hofmann, M. Hofmann and B. Von Rechenberg, J. Magn. Magn. Mater., 2005, 293, 483 CrossRef CAS.
- J. Dobson, Drug Dev. Res., 2006, 67, 55 CrossRef CAS.
- C. Alexiou, W. Arnold, P. Hulin, R. J. Klein, H. Renz, F. G. Parak, C. Bergemann and A. S. Lubbe, J. Magn. Magn. Mater., 2001, 225, 187 CrossRef CAS.
- C. Alexiou, W. Arnold, R. J. Klein, F. G. Parak, P. Hulin, C. Bergemann, W. Erhardt, S. Wagenpfeil and A. S. Lubbe, Cancer Res., 2000, 60, 6641 CAS.
- J. M. Gallo, U. Hafeli and A. S. Lubbe, Cancer Res., 1997, 57, 3063 CAS.
- A. S. Lubbe, C. Alexiou and C. Bergemann, J. Surg. Res., 2001, 95, 200 CrossRef CAS.
- S. Rudge, C. Peterson, C. Vessely, J. Koda, S. Stevens and L. Catterall, J. Controlled Release, 2001, 74, 335 CrossRef CAS.
- A. S. Lubbe, C. Bergemann, H. Riess, F. Schriever, P. Reichardt, K. Possinger, M. Matthias, B. Dorken, F. Herrmann, R. Gurtler, P. Hohenberger, N. Haas, R. Sohr, B. Sander, A. J. Lemke, D. Ohlendorf, W. Huhnt and D. Huhn, Cancer Res., 1996, 56, 4686 CAS.
- C. Alexiou, W. Arnold, R. J. Klein, F. G. Parak, P. Hulin, C. Bergemann, W. Erhardt, S. Wagenpfeil and A. S. Lubbe, Cancer Res., 2000, 60, 6641 CAS.
- S. C. Goodwin, C. A. Bittner, C. L. Peterson and G. Wong, Toxicol. Sci., 2001, 60, 177 CrossRef CAS.
- S. Goodwin, C. Peterson, C. Hoh and C. Bittner, J. Magn. Magn. Mater., 1998, 194, 132 CrossRef.
- S. K. Pulfer and J. M. Gallo, J. Drug Targeting, 1998, 6, 215 CrossRef CAS.
- S. K. Pulfer, S. L. Ciccotto and J. M. Gallo, J. Neuro-Oncol., 1999, 41, 99 CrossRef CAS.
- C. Alexiou, R. Jurgons, R. J. Schmid, C. Bergemann, J. Henke, W. Erhardt, E. Huenges and F. Parak, J. Drug Targeting, 2003, 11, 139 CrossRef CAS.
- C. Alexiou, R. Jurgons, R. Schmid, A. Hilpert, C. Bergemann, F. Parak and H. Iro, J. Magn. Magn. Mater., 2005, 293, 389 CrossRef CAS.
- S. Goodwin, C. Peterson, C. Hoh and C. Bittner, J. Magn. Magn. Mater., 1999, 194, 132 CrossRef CAS.
- O. Mykhaylyk, N. Dudchenko and A. Dudchenko, J. Magn. Magn. Mater., 2005, 293, 473 CrossRef CAS.
- B. Chertok, B. A. Moffat, A. E. David, F. Yu, C. Bergemann, B. D. Ross and V. C. Yang, Biomaterials, 2008, 29, 487 CrossRef CAS.
- Y. H. Ma, Y. W. Hsu, Y. J. Chang, M. Y. Hua, J. P. Chen and T. Wu, J. Magn. Magn. Mater., 2007, 311, 342 CrossRef CAS.
- K. Schulze, A. Koch, B. Schopf, A. Petri, B. Steitz, M. Chastellain, M. Hofmann, H. Hofmann and B. Von Rechenberg, J. Magn. Magn. Mater., 2005, 293, 419 CrossRef CAS.
- H. Xu, T. Song, X. Bao and L. Hu, J. Magn. Magn. Mater., 2005, 293, 514 CrossRef CAS.
- K. J. Widder, R. M. Morris and G. A. Poore, Eur. J. Cancer Clin. Oncol., 1983, 19, 135 CrossRef CAS.
- K. J. Widder, P. A. Marino and R. M. Morris, Eur. J. Cancer Clin. Oncol., 1983, 19, 141 CrossRef CAS.
- A. S. Lubbe, C. Bergemann, J. Brock and D. G. McClure, J. Magn. Magn. Mater., 1999, 194, 149 CrossRef CAS.
- S. K. Pulfer and J. M. Gallo, J. Drug Targeting, 1998, 6, 215 CrossRef CAS.
- T. Kubo, T. Sugita, S. Shimose, Y. Nitta, Y. Ikuta and T. Murakami, Int. J. Oncol., 2000, 17, 309 CAS.
- H. P. Kok, P. M. A. Van Haaren, J. B. Van De Kamer, J. Wiersma, J. D. P. Van Dijk and J. Crezee, Phys. Med. Biol., 2005, 50, 3127 CrossRef CAS.
- A. Jordan, R. Scholz, K. Maier-Hauff, M. Johannsen, P. Wust, J. Nadobny, H. Schirra, H. Schmidt, S. Deger, S. Loening, W. Lanksch and R. Felix, J. Magn. Magn. Mater., 2001, 225, 118 CrossRef CAS.
- A. Jordan, R. Scholz, K. Maier-Hauff, F. K. van Landeghem, N. Waldoefner, U. Teichgraeber, J. Pinkernelle, H. Bruhn, F. Neumann, B. Thiesen, A. von Deimling and R. Felix, J. Neuro-Oncol., 2005, 29, 1 Search PubMed.
- A. Jordan, R. Scholz, P. Wust, H. Fahling and R. Felix, J. Magn. Magn. Mater., 1999, 201, 413 CrossRef CAS.
- I. Hilger, R. Hiergeist, R. Hergt, K. Winnefeld, H. Schubert and W. A. Kaiser, Invest. Radiol., 2002, 37, 580 CrossRef CAS.
- U. Hafeli, G. Pauer, S. Failing and G. Tapolsky, J. Magn. Magn. Mater., 2001, 225, 73 CrossRef CAS.
- J. Cao, Y. Wang, J. Yu, J. Xia, C. Zhang, D. Yin and U. O. Hafeli, J. Magn. Magn. Mater., 2004, 277, 165 CrossRef CAS.
- J. Lee, T. Isobe and M. Senna, J. Colloid Interface Sci., 1996, 177, 490 CrossRef CAS.
- S. Kayal and R. V. Ramanujan, J. Nanosci. Nanotechnol., 2010, 10, 5527 CrossRef CAS.
- M. Mahmoudi, A. Simchi, M. Imani, A. S. Milani and P. Stroeve, J. Phys. Chem. B, 2008, 112, 14470 CrossRef CAS.
- X. L. Li, K. L. Yao and Z. L. Liu, J. Magn. Magn. Mater., 2008, 320, 1753 CrossRef CAS.
- O. Rotariu and N. J. C. Strachan, J. Magn. Magn. Mater., 2005, 293, 639 CrossRef CAS.
- A. D. Grief and G. Richardson, J. Magn. Magn. Mater., 2005, 293, 455 CrossRef CAS.
- M. O. Aviles, A. D. Ebner, H. Chen, A. J. Rosengart, M. D. Kaminski and J. A. Ritter, J. Magn. Magn. Mater., 2005, 293, 605 CrossRef CAS.
- J. A. Ritter, A. D. Ebner, K. D. Daniel and K. L. Stewart, J. Magn. Magn. Mater., 2004, 280, 184 CrossRef CAS.
- O. Rotariu and N. J. C. Strachan, J. Magn. Magn. Mater., 2005, 293, 639 CrossRef CAS.
- G. Iacob, O. Rotariu, N. J. C. Strachan and U. O. Hafeli, Biorheology, 2004, 41, 599 CAS.
- G. Iacob, O. Rotariu and H. Chiriac, J. Optoelectron. Adv. Mater., 2004, 6, 713 Search PubMed.
- M. O. Aviles, A. D. Ebner and J. A. Ritter, J. Magn. Magn. Mater., 2007, 310, 131 CrossRef CAS.
- Z. G. Forbes, B. B. Yellen, K. A. Barbee and G. Friedman, IEEE Trans. Magn., 2003, 39, 3372 CrossRef CAS.
- H. Chen, A. D. Ebner, A. J. Rosengart, M. D. Kaminski and J. A. Ritter, J. Magn. Magn. Mater., 2004, 284, 181 CrossRef CAS.
- H. Chen, A. D. Ebner, M. D. Kaminski, A. J. Rosengart and J. A. Ritter, J. Magn. Magn. Mater., 2005, 293, 616 CrossRef CAS.
- B. B. Yellen, Z. G. Forbes, D. S. Halverson, G. Fridman, K. A. Barbee, M. Chorny, R. Levy and G. Friedman, J. Magn. Magn. Mater., 2005, 293, 647 CrossRef CAS.
- E. P. Furlani and K. C. Ng, Phys. Rev. E: Stat., Nonlinear, Soft Matter Phys., 2006, 73, 061919 CrossRef CAS.
- E. J. Furlani and E. P. Furlani, J. Magn. Magn. Mater., 2007, 312, 187 CrossRef CAS.
-
R. B. Bird, W. E. Stewart, E. N. Lightfoot, Transport Phenomena, John Wiley & Sons., 2007 Search PubMed.
- S. Kayal and R. V. Ramanujan, Mater. Sci. Eng., C, 2010, 30, 484 CrossRef CAS.
-
E. P. Furlani, Permanent magnet and electromechanical device: materials,analysis and aplications, Academic, New York, 2001 Search PubMed.
- L. E. Udrea, N. J. C. Strachan, V. Badescu and O. Rotariu, Phys. Med. Biol., 2006, 51, 4869 CrossRef CAS.
|
This journal is © The Royal Society of Chemistry 2011 |
Click here to see how this site uses Cookies. View our privacy policy here.