DOI:
10.1039/C1PY00187F
(Paper)
Polym. Chem., 2011,
2, 2239-2248
Synthesis of glycosylated peptides by NCA polymerization for recognition of human T-cells†
Received
29th April 2011
, Accepted 3rd July 2011
First published on 28th July 2011
Abstract
Peracetylated sugars (glucose, mannose, galactose) were attached via a thiourea linker to the ε-amino group of α-BOC-lysine and α-Z-lysine. After transformation to the N-carboxyanhydride (NCA), the product was copolymerized with both PEGylated lysine-NCA and ε-TFA-lysine-NCA by both tertiary amine and nickel complex catalysts. The resulting statistical copolypeptides are water-soluble after global deprotection and show an α-helical secondary structure. Fluorescein isothiocyanate (FITC) was then coupled to the free ε-NH2 groups of the lysine repeating units. The galactosylated fluorescent peptides are specifically incorporated in human T lymphocytes at 37 °C, as shown by flow cytometry and fluorescence microscopy. Therefore they are potentially useful for selective staining of cells or targeted drug delivery.
Introduction
The recognitions between different cells, between viruses and cells, and between tissues and cells are controlled by the cooperativity of rather weak ligand–receptor interactions. Among others, carbohydrates like glucose, mannose, galactose, and lactose as well as complex oligosaccharides are well-known ligands for biological receptors based within cell membranes.1,2 Binding interactions between carbohydrate ligands and receptor proteins are indeed highly specific, but generally rather weak compared to other biological interactions.3 Dissociation constants range mostly between 50 μM and 1 mM. Therefore, molecular recognition between biological entities requires the interplay of more than one receptor–ligand interaction.
Cooperativity of binding can also be used in artificial systems to improve binding affinities. In the so-called ‘multivalency approach’, many ligands are connected to an inert polymer backbone to reach manifold binding.4 Therefore, many carbohydrate ligands have been attached to polymers,5,6carbon nanotubes,7 and cyclic scaffolds, e.g., cyclodextrins8–10 or spherical molecules such as dendrimers.11 The resulting so-called ‘glycoclusters’ or ‘neoglycoconjugates’ indeed showed improved binding to multivalent receptors, e.g., lectins,12–15in vitro and to cellsin vivo,16 and are potentially useful for anti-adhesion therapy17,18 or targeted delivery.19 Despite the successes of the multivalency approach, some drawbacks still obstruct broad application. Firstly, glycoclusters are often not biodegradable because mostly synthetic scaffolds are used in their synthesis. Secondly, polymers and high molecular weight glycoclusters tend to bind nonspecifically to biological surfaces, i.e., by Coulomb interactions between oppositely charged species.
In the following, we describe a new approach using a helical synthetic polypeptide as the framework for the attachment of carbohydrate ligands. Polypeptides are biodegradable by proteolytic enzymes. Also, the α-helix has already proven its use as a rigid scaffold for the investigation of molecular recognition between attached complementary binding sites.20 Homopolyamino as well as copolyamino acids are readily available through the ring-opening polymerization of N-carboxyanhydrides (NCAs).21,22 Our approach is based on previous work about living coordinative polymerization of NCAs with Ni0 catalysts,23,24 and synthesis of water-soluble monoethylene and diethylene glycol-functionalized polylysines that form α-helices.25Glycopeptides had been already synthesized recently by polymer-analogous modification of polylysine with glycosyl-isothiocyanates26 or by polymerization of C-glycosylated-L-lysine NCAs.27 PEGylation of polymers is known to prevent phagocytosis by the immune system.28 We found a straightforward method for the synthesis of neutral O-glycosylated polylysine derivatives with attached diethylene glycol chains to avoid unspecific binding onto cell surfaces. The specific interactions of these glycosylated peptides were investigated with T lymphocytes because they are very promising therapeutic drug carriers for the treatment of cancer.29
Experimental section
Instrumentation
Nuclear magnetic resonance.
1H and 13C NMR spectra were recorded on a Bruker AVANCE 500 (1H: 500.27 MHz, 13C: 125.79 MHz) or a Bruker Magnet System 400 MHz Ultra shield plus (1H: 400.00 MHz) instrument using CDCl3 and DMSO-d6 at 25 °C. The solvent signals were used as internal standards. The following abbreviations were used for multiplicities: s for singlet, d for duplet, dd for double duplet, t for triplet, q for quartet and quin for quintet. Overlapping signals and multiplets were labeled with m, broad signals with br.
Fluorescence spectrometry
.
Fluorescence intensities of the polymers 6a–c and 6u, c = 20 mg L−1, in RPMI 1640 culture medium with 10% FBS and 1% penicillin/streptomycin at pH 7.5 were measured in a black 96-well microplate, Art.-Nr.: 655900 Greiner Bio-One (Germany) with a Modulus™ Microplate Multimode Reader (Turner BioSystems, Sunnyvale, CA 94085, USA), excitation wavelength 490 nm, emission wavelength 510–570 nm at 25 °C.
Size exclusion chromatography
.
SEC was performed at 25 °C using two MCX columns with pore sizes of 103 and 106 Å (Polymer Standard Service GmbH), a reflective index (RI), and a UV (315 nm) detector. THF or N,N-dimethylacetamide were used as eluents and the elution times were converted to molecular weights with a calibration curve constructed from narrow polystyrene standards.
Materials and synthetic procedures
N
ε-Trifluoroacetyl-L-lysine-N-carboxyanhydride
30 and Nε-(3,6,9-trioxa-decanoyl)-L-lysine-N-carboxyanhydride25 (both isolated according to Poché et al.31) as well as 2,3,4,6-tetra-O-acetyl-1-O-trichloracetimidato-α-D-mannopyranose,322,3,4,6-tetra-O-acetyl-1-O-trichloracetimidato-β-D-glucopyranose,322,3,4,6-tetra-O-acetyl-1-O-trichloracetimidato-β-D-galactopyranose,332-(2-isothiocyanatoethoxy)ethanol,34 and (2,2′-bipyridine)(1,5-cyclooctadien)nickel(0) (bpyNi(COD))35 were prepared according to literature procedures. N,N′-Dimethylformamide (DMF) was distilled from P2O5 under reduced pressure and stored over molecular sieves 4 Å under Ar. Tetrahydrofuran (THF) was distilled from sodium and subsequently stored over molecular sieves 4 Å under Ar. Triethylamine was freshly distilled from CaH2. All other reactants and solvents were purchased from commercial suppliers and used without further purification. Water was doubly distilled in a glass apparatus.
5-Isothiocyanato-3-oxapentyl 2,3,4,6-tetra-O-acetyl-1-α-D-mannopyranoside (1a).
2,3,4,6-Tetra-O-acetyl-1-O-trichloracetimidato-α-D-mannopyranose (8.0 g, 16.2 mmol), 4 Å molecular sieves (1.0 g) and 2-(2-isothiocyanatoethoxy)ethanol (4.8 g, 32.6 mmol) were dissolved in anhydrous dichloromethane (150 mL) under an N2 atmosphere. After stirring for 1 h, the reaction mixture was cooled to −78 °C. Trimethylsilyl trifluoromethanesulfonate (1.2 mL, 6.5 mmol) was added and the mixture was allowed to warm to 25 °C over 5 h. The mixture was filtered over Celite and washed successively with sodium hydrogen carbonate and sodium chloride solutions. The organic layer was separated and dried over anhydrous MgSO4. The solvent was evaporated and the crude residue was purified by column chromatography (chloroform/ethyl acetate 85
:
15) to yield 1a as a white solid (5.44 g, 70%). 1H NMR (CDCl3): δ/ppm = 1.96, 2.01, 2.07, 2.12 (4 s, 12H, –(C
O)OCH3), 3.63–3.85 (m, 8H, –OCH2CH2OCH2CH2NCS), 4.01–4.06 (m, 1H, H-5), 4.07–4.11 (m, 1H, H-6), 4.26 (dd, 1H, H-6′), 4.86 (d, 3JHH = 1.5 Hz, 1H, H-1), 5.24 (dd, 1H, H-2), 5.25 (t, 1H, H-4), 5.33 (dd, 1H, H-3); 13C NMR (CDCl3): δ/ppm = 20.62, 20.66, 20.70, 20.83, 45.26, 62.47, 66.07, 67.30, 68.47, 69.02, 69.37, 69.46, 70.15, 97.68, 133.01, 169.68, 169.85, 169.97, 170.57; FTIR (cm−1) = 1043, 1217, 1738, 2106, 2938, 3241, 3360; ESI-MS (m/z) [M + Na]+ calcd for C19H27NNaO11S, 500.12; found, 500.36.
5-Isothiocyanato-3-oxapentyl 2,3,4,6-tetra-O-acetyl-β-D-glucopyranoside (1b).
1b was synthesized with a 67% yield according to the procedure described for 1a. 1H NMR (CDCl3): δ/ppm = 1.98, 2.02, 2.04, 2.07 (4 s, 12H, –(C
O)OCH3), 3.61–3.67 (m, 6H, –OCH2CH2OCH2CH2NCS), 3.69–3.97 (m, 3H, –OCH2CH2O, H-5), 4.13 (dd, 1H, H-6), 4.25 (dd, 1H, H-6′), 4.59 (d, 3JHH = 8.0 Hz, 1H, H-1), 4.97 (dd, 1H, H-2), 5.06 (t, 1H, H-4), 5.21 (t, 1H, H-3); 13C NMR (CDCl3): δ/ppm = 20.57, 20.58, 20.67, 20.73, 45.21, 61.86, 68.30, 69.10, 69.31, 70.35, 71.23, 71.76, 72.72, 100.73, 132.71, 169.42, 169.44, 170.23, 170.68; FTIR (cm−1) = 1032, 1208, 1745, 2140, 2201, 2884, 2955, 3238, 3356; ESI-MS (m/z) [M + Na]+ calcd for C19H27NNaO11S, 500.12; found, 500.36.
5-Isothiocyanato-3-oxapentyl 2,3,4,6-tetra-O-acetyl-β-D-galactopyranoside (1c).
1c was synthesized according to the procedure described for 1a in 74% yield. 1H NMR (CDCl3): δ/ppm = 1.96, 2.03, 2.04, 2.12 (4 s, 12H, –(C
O)OCH3), 3.62–3.67 (m, 6H, –OCH2CH2OCH2CH2NCS), 3.71–3.97 (m, 3H, –OCH2CH2O, H-5), 4.06–4.18 (m, 2H, H-6), 4.55 (d, 3JHH = 8.0 Hz, 1H, H-1), 5.02 (dd, 1H, H-3), 5.18 (dd, 1H, H-2), 5.37 (dd, 1H, H-4); 13C NMR (CDCl3): δ/ppm = 20.53, 20.62, 20.64, 20.76, 45.26, 61.21, 66.97, 68.75, 69.06, 69.31, 70.37, 70.64, 70.81, 101.24, 132.69, 169.96, 170.07, 170.20, 170.36; FTIR (cm−1) = 1042, 1214, 1741, 2113, 2200, 2875, 2941, 3183, 3357; ESI-MS (m/z) [M + Na]+ calcd for C19H27NNaO11S, 500.12; found, 500.36.
N
ε-(8-(2,3,4,6-Tetra-O-acetyl-α-D-mannopyranosyl)-1-thioxo-2-aza-5,8-dioxa-octyl)-Nα-(benzyloxycarbonyl)-L-lysine (2a).
1a (5.22 g, 10.9 mmol) and Nα-benzyloxycarbonyl-L-lysine (4.6 g, 16.4 mmol) were suspended in DMF (100 mL). DIPEA (6 mL) was added and the suspension was stirred at 25 °C for 30 min. Distilled water was added until a clear solution was obtained and the reaction mixture was stirred for 3 d at 25 °C. The solution was acidified to pH 5 with acetic acid and concentrated in vacuum to a residual volume of about 20 mL. Distilled water (100 mL) was added and the product was extracted three times with ethyl acetate (100 mL). The organic fractions were combined, washed with sodium hydrogen carbonate and sodium chloride, and dried over anhydrous MgSO4. The solvent was removed under vacuum to leave a white solid (6.7 g, 8.8 mmol). The crude product was purified by column chromatography (ethyl acetate/ethanol/acetic acid 100
:
2
:
0.6, v/v/v). The combined organic fractions were concentrated in vacuum to a residual volume of about 10 mL, diluted with 150 mL ethyl acetate and washed several times with sodium hydrogen carbonate and sodium chloride to remove the acetic acid. Finally, the organic fraction was dried over anhydrous MgSO4 and the solvent was evaporated under vacuum. The resulting white solid was received in 43% yield. 1H NMR (CDCl3): δ/ppm = 1.39 (m, 2H, –NHCHCH2CH2CH2CH2–), 1.55 (m, 2H, –NHCHCH2CH2CH2CH2–), 1.72 (m, 1H, –NHCHCH2CH2CH2CH2–), 1.82 (m, 1H, –NHCHCH2CH2CH2CH2–), 1.96, 2.02, 2.07, 2.12 (4 s, 12H, –(C
O)OCH3), 3.40 (m, 2H, –NHCHCH2CH2CH2CH2–), 3.57–3.76 (m, 8H, –OCH2CH2OCH2CH2NH–), 4.00 (m, 1H, H-5), 4.10 (m, 1H, H-6), 4.24 (m, 2H, H-6′, –NHCH(R)C(O)OH), 4.88 (br, 1H, H-1), 5.06 (m, 2H, –CH2C6H5), 5.20–5.32 (m, 3H, H-2, H-3, H-4), 5.77 (br, 1H, NHCH(R)C(O)OH), 6.40/6.74 (2 br, 2H, –NHC(S)NH–), 7.30 (m, 5H, –CH2C6H5); 13C NMR (CDCl3): δ/ppm = 20.67, 20.72, 20.90, 22.42, 28.31, 31.69, 43.92, 44.33, 53.93, 62.52, 66.11, 66.85, 67.00, 68.47, 69.10, 69.70, 69.86, 70.15, 97.48, 128.01, 128.14, 128.48, 136.15, 156.34, 169.73, 170.41, 170.66, 170.79, 171.17, 211.62; FTIR (cm−1) = 977, 1042, 1135, 1216, 1367, 1436, 1454, 1536, 1739, 2867, 2935, 3344; ESI-MS (m/z) [M + H]+ calcd for C33H47N3O15S, 757.80; found, 757.31.
N
ε-(8-(2,3,4,6-Tetra-O-acetyl-β-D-glucopyranosyl)-1-thioxo-2-aza-5,8-dioxa-octyl)-Nα-(benzyloxycarbonyl)-L-lysine (2b).
2b was synthesized according to the procedure described for 2a in 36% yield. 1H NMR (CDCl3): δ/ppm = 1.39–1.50 (m, 2H, –NHCHCH2CH2CH2CH2–), 1.62 (m, 2H, –NHCHCH2CH2CH2CH2–), 1.72–1.92 (m, 2H, –NHCHCH2CH2CH2CH2–), 1.99, 2.01, 2.03, 2.06 (4 s, 12H, –(C
O)OCH3), 3.46 (m, 2H, –NHCHCH2CH2CH2CH2–), 3.55–3.96 (m, 9H, –OCH2CH2OCH2CH2NH–, H-5), 4.14 (dd, 1H, H-6), 4.26 (m, 2H, H-6′ –NHCH(R)C(O)OH), 4.54 (br, 1H, H-1), 4.97 (m, 2H, H-2), 5.09 (m, 3H, –CH2C6H5, H-4), 5.22 (d, 3H, H-3), 5.60 (d, 1H, –NHCH(R)C(O)OH), 6.33/6.72 (2 br, 2H, –NHC(S)NH–), 7.27–34 (m, 5H, –CH2C6H5); 13C NMR (CDCl3): δ/ppm = 20.57, 20.70, 20.78, 22.26, 28.30, 31.78, 43.82, 44.36, 53.49, 61.86, 67.04, 68.37, 68.91, 69.61, 69.88, 71.58, 71.88, 72.45, 100.83, 128.07, 128.18, 128.52, 136.19, 156.12, 169.61, 170.34, 170.79, 174.81, 211.63; FTIR (cm−1) = 909, 1031, 1118, 1213, 1366, 1455, 1531, 1738, 2871, 2938, 3356; ESI-MS (m/z) [M + H]+, calcd for C33H47N3O15S, 757.80; found, 757.31.
N
ε-(8-(2,3,4,6-Tetra-O-acetyl-β-D-galactopyranosyl)-1-thioxo-2-aza-5,8-dioxa-octyl)-Nα-(benzyloxycarbonyl)-L-lysine (2c).
2c was synthesized according to the procedure described for 2a in 20% yield. 1H NMR (CDCl3): δ/ppm = 1.42 (m, 2H, –NHCHCH2CH2CH2CH2–), 1.60 (m, 2H, –NHCHCH2CH2CH2CH2–), 1.71–1.92 (m, 2H, –NHCHCH2CH2CH2CH2–), 1.98, 2.03, 2.04, 2.13 (4 s, 12H, –(C
O)OCH3), 3.46 (m, 2H, –NHCHCH2CH2CH2CH2–), 3.54–3.97 (m, 9H, –OCH2CH2OCH2CH2NH–, H-5), 4.10 (dd, 1H, H-6), 4.16 (dd, 1H, H-6′), 4.35 (m, 1H, –NHCH(R)C(O)OH), 4.48 (d, 1H, H-1), 5.04 (dd, 2H, H-3), 5.08 (br, 2H, –CH2C6H5), 5.15 (dd, 1H, H-2), 5.38 (dd, 3H, H-4), 5.65 (d, 1H, – NHCH(R)C(O)OH), 6.37/6.83 (2 br, 2H, –NHC(S)NH–), 7.27–7.33 (m, 5H, –CH2C6H5); 13C NMR (CDCl3): δ/ppm = 20.57, 20.62, 20.67, 20.88, 22.29, 28.32, 31.74, 43.77, 44.23, 53.54, 61.19, 66.91, 67.02, 68.93, 69.17, 69.54, 71.53, 70.69, 101.30, 128.06, 128.18, 128.51, 136.10, 156.13, 170.18, 170.22, 170.56, 175.12, 211.60; FTIR (cm−1) = 955, 1040, 1132, 1175, 1215, 1367, 1436, 1455, 1532, 1738, 2870, 2935, 3353; ESI-MS (m/z) [M + H]+ calcd for C33H47N3O15S, 757.80; found, 757.31.
N
ε-(8-(2,3,4,6-Tetra-O-acetyl-α-D-mannopyranosyl)-1-thioxo-2-aza-5,8-dioxa-octyl)-L-lysine-N-carboxyanhydride (3a).
2a (2.0 g, 2.6 mmol) was dissolved in anhydrous dichloromethane (100 mL) under nitrogen. After the addition of dichloromethyl methyl ether (0.6 mL, 6.6 mmol), the solution was heated to reflux for 24 h. The solvent was evaporated and the residue was dissolved in ethyl acetate (150 mL). The resulting solution was washed rapidly with pre-chilled water and sodium hydrogen carbonate. The organic layer was dried over anhydrous MgSO4 and the solvent was removed under vacuum. The residue was dissolved in anhydrous THF and precipitated in n-hexane (200 mL). The mixture was placed in the refrigerator for 18 h to complete the precipitation. The product 3a was obtained as a white solid (1.7 g, 76% yield). 1H NMR (CDCl3): δ/ppm = 1.32–1.70 (m, 4H, –NHCHCH2CH2CH2CH2–), 1.89 (m, 2H, –NHCHCH2CH2CH2CH2–), 1.95, 2.02, 2.07, 2.12 (4 s, 12H, –(C
O)OCH3), 3.18 (m, 2H, –NHCHCH2CH2CH2CH2–), 3.35 (m, 2H, OCH2CH2NH–), 3.45–3.86 (m, 6H, –OCH2CH2OCH2CH2NH–), 4.02–4.07 (m, 3H, H-5, H-6, –NHCH–), 4.25 (dd, 1H, H-6′), 4.84 (m, 1H, H-1), 5.22–5.26 (m, 3H, H-2, H-3, H-4); 13C NMR (CDCl3): δ/ppm = 20.68, 20.85, 23.59, 30.73, 31.12, 37.85, 46.21, 62.37, 66.06, 67.29, 68.40, 69.05, 69.44, 69.80, 71.06, 97.68, 150.90, 169.69, 169.92, 170.65, 211.45; FTIR (cm−1) = 973, 1043, 1134, 1217, 1367, 1432, 1532, 1657, 1740, 1783, 1852, 2934.
N
ε-(8-(2,3,4,6-Tetra-O-acetyl-β-D-glucopyranosyl)-1-thioxo-2-aza-5,8-dioxa-octyl)-L-lysine-N-carboxyanhydride (3b).
3b was synthesized according to the procedure described for 3a in 80% yield. 1H NMR (CDCl3): δ/ppm = 1.27–1.91 (m, 6H, –NHCHCH2CH2CH2CH2–), 1.98, 2.00, 2.05, 2.06 (4 s, 12H, –(C
O)OCH3), 3.12–3.89 (m, 12H, –NHCHCH2CH2CH2CH2–, –OCH2CH2OCH2CH2NH–, –NHCH–, H-5), 4.07–4.13 (m, 1H, H-6), 4.12–4.25 (m, 1H, H-6′), 4.56 (m, 1H, H-1), 4.94 (m, 1H, H-2), 5.06 (t, 1H, H-4), 5.19 (m, 1H, H-3); 13C NMR (CDCl3): δ/ppm = 20.57, 20.72, 21.00, 23.69, 31.19, 31.44, 36.39, 46.31, 61.93, 68.41, 69.02, 69.93, 71.22, 71.77, 72.78, 100.86, 151.28, 169.27, 169.37, 170.16, 170.23, 170.62, 211.63; FTIR (cm−1) = 907, 1032, 1120, 1213, 1365, 1433, 1454, 1532, 1651, 1742, 1783, 1852, 2867, 2939, 3306.
N
ε-(8-(2,3,4,6-Tetra-O-acetyl-β-D-galactopyranosyl)-1-thioxo-2-aza-5,8-dioxa-octyl)-L-lysine-N-carboxyanhydride (3c).
3c was synthesized according to the procedure described for 3a in 82% yield. 1H NMR (CDCl3): δ/ppm = 1.09–1.86 (m, 6H, –NHCHCH2CH2CH2CH2–), 1.96, 2.03, 2.13 (3 s, 12H, –(C
O)OCH3), 3.27–4.38 (m, 12H, –NHCHCH2CH2CH2CH2–, –OCH2CH2OCH2CH2NH–, –NHCH–, H-5, H-6), 4.53 (m, 1H, H-1), 5.03 (m, 1H, H-3), 5.13 (m, 1H, H-2), 5.37 (m, 1H, H-4); 13C NMR (CDCl3): δ/ppm = 20.54, 20.63, 20.76, 24.04, 31.16, 31.25, 38.74, 46.33, 61.21, 67.01, 68.77, 68.91, 69.96, 70.05, 70.67, 70.84, 101.32, 151.68, 170.09, 170.18, 170.32, 211.62; FTIR (cm−1) = 955, 1043, 1126, 1173, 1215, 1366, 1432, 1531, 1658, 1742, 1783, 1852, 2870, 2936, 3295.
NCA-polymerization in DMF using triethylamine.
N
ε-(3,6,9-trioxa-decanoyl)-L-lysine-N-carboxyanhydride (1.23 g, 3.70 mmol), and one of the glycosylated L-lysine-NCAs 3a–c (0.30 g, 0.46 mmol) were dissolved in freshly distilled DMF (30 mL) under an N2 atmosphere. Distilled triethyl amine (14 mg, 19 μL, 0.14 mmol) was added to this 5 wt% NCA solution and the reaction mixture was stirred at 25 °C. After 2 d, the reaction mixture was added to a 20-fold excess of diethyl ether. The precipitated polymer was isolated and dried. The crude product was dissolved in methanol and purified by continuous ultrafiltration through a cellulose membrane with a 5 kDa molecular weight cut-off against a 10-fold amount of methanol. Lyophilization afforded the products 4a–c as white, cotton-like solids.
For the synthesis of the terpolymer 5c, Nε-trifluoroacetyl-L-lysine-N-carboxyanhydride (0.062 g, 0.23 mmol), Nε-(3,6,9-trioxa-decanoyl)-L-lysine-N-carboxyanhydride (1.23 g, 3.70 mmol), and the glycosylated L-lysine-NCA 3c (0.30 g, 0.46 mmol) were polymerized with triethyl amine (15 mg, 20 μL, 0.15 mmol) according to the same procedure. The copolymer 5u was synthesized accordingly using triethyl amine (6 mg, 8 μL, 0.06 mmol), Nε-(3,6,9-trioxa-decanoyl)-L-lysine-N-carboxyanhydride (0.92 g, 2.75 mmol) and Nε-trifluoroacetyl-L-lysine-N-carboxyanhydride (0.041 g, 0.15 mmol).
NCA-polymerization in DMF using bpyNi(COD).
A Schlenk flask sealed with a rubber septum was charged with a solution of Nε-(3,6,9-trioxa-decanoyl)-L-lysine-N-carboxyanhydride (1.23 g, 3.70 mmol), and one of the glycosylated L-lysine-NCAs 3a–c (0.27 g, 0.41 mmol) in 30 mL anhydrous DMF. The solution was deoxygenated three times by vacuum and ventilation with Ar. Afterwards, bpyNi(COD) (32 mg, 0.1 mmol for 4a′, 26 mg, 0.08 mmol for 4b′ and 16 mg, 0.05 mmol for 4c′, synthesized from Ni0(COD)2 by ligand exchange according to the literature35) was added as a solution in 0.5 mL DMF and stirred at 25 °C for 2 d. The polymer was precipitated by adding the reaction mixture to 600 mL diethyl ether. The crude product was dissolved in 50 mL methanol and purified by continuous ultrafiltration through a cellulose membrane with a 5 kDa molecular weight cut-off with 500 mL methanol. After lyophilization, the polypeptides were obtained as white, cotton-like solids.
For the synthesis of the terpolymers 5a and 5b, Nε-trifluoroacetyl-L-lysine-N-carboxyanhydride (0.1 g, 0.38 mmol), Nε-(3,6,9-trioxa-decanoyl)-L-lysine-N-carboxyanhydride (2.0 g, 6.16 mmol), and the glycosylated L-lysine-NCA 3a respectively 3b (0.50 g, 0.77 mmol) were polymerized with bpyNi(COD) (30 mg, 0.09 mmol) according to the same procedure.
Poly([Nε-(3,6,9-trioxa-decanoyl)-L-lysine]-co-[Nε-trifluoroacetyl-L-lysine]) (5u).
1H NMR (CDCl3): δ/ppm = 1.14–1.90 (m, –NHCHCH2CH2CH2CH2–), 3.05 (m, –NHCHCH2CH2CH2CH2–), 3.22 (s, CH3O–), 3.42 (m, CH3OCH2CH2O–), 3.53 (m, –OCH2CH2OCH2CH2O–, –NHCH(R)C(O)–), 3.84 (m, –OCH2C(O)NH–), 7.66 (m, NH), 9.37 (m, NH); FTIR (cm−1) = 3287, 3066, 2923, 2865, 1717, 1650, 1535, 1440, 1339, 1283, 1247, 1199, 1100, 1028, 934, 850.
Poly([Nε-(3,6,9-trioxa-decanoyl)-L-lysine]-co-[Nε-(8-(2,3,4,6-tetra-O-acetyl-α-D-mannopyranosyl)-1-thioxo-2-aza-5,8-dioxa-octyl)-L-lysine]-co-[Nε-trifluoroacetyl-L-lysine]) (5a).
1H NMR (CDCl3): δ/ppm = 1.23–2.19 (m, –NHCHCH2CH2CH2CH2–, –(C
O)OCH3), 3.21–3.34 (m, –NHCHCH2CH2CH2CH2–, CH3OCH2–), 3.52–4.25 (m, –NHCH(R)C(O)–, –OCH2CH2OCH2CH2OCH2C(O)NH–, –OCH2CH2OCH2CH2NH–, H-5, H-6), 4.84 (m, H-1), 5.25 (m, H-2, H-3, H-4), 7.17 (br, NH), 8.19 (s, NH), 8.80 (s, NH); FTIR (cm−1) = 3292, 3066, 2921, 2867, 1747, 1719, 1650, 1536, 1440, 1369, 1338, 1283, 1224, 1200, 1100, 1047, 981, 933, 849.
Poly([Nε-(3,6,9-Trioxa-decanoyl)-L-lysine]-co-[Nε-(8-(2,3,4,6-tetra-O-acetyl-β-D-glucopyranosyl)-1-thioxo-2-aza-5,8-dioxa-octyl)-L-lysine]-co-[Nε-trifluoroacetyl-L-lysine]) (5b).
1H NMR (CDCl3): δ/ppm = 1.23–2.06 (m, –NHCHCH2CH2CH2CH2–, –(C
O)OCH3), 3.21–3.34 (m, –NHCHCH2CH2CH2CH2–, CH3OCH2–), 3.51–4.26 (m, –NHCH(R)C(O)–, –OCH2CH2OCH2CH2OCH2C(O)NH–, –OCH2CH2OCH2CH2NH–, H-5, H-6), 4.57 (m, H-1), 4.95 (m, H-2), 5.05 (t, H-4), 5.19 (m, H-3), 7.17 (br, NH), 8.20 (s, NH), 8.80 (s, NH); FTIR (cm−1) = 3292, 3067, 2923, 2866, 1754, 1719, 1650, 1537, 1440, 1365, 1339, 1281 1223, 1099, 1034, 931, 849.
Poly([Nε-(3,6,9-trioxa-decanoyl)-L-lysine]-co-[Nε-(8-(2,3,4,6-tetra-O-acetyl-β-D-galactopyranosyl)-1-thioxo-2-aza-5,8-dioxa-octyl)-L-lysine]-co-[Nε-trifluoroacetyl-L-lysine]) (5c).
1H NMR (CDCl3): δ/ppm = 1.40–2.13 (m, –NHCHCH2CH2CH2CH2–, –(C
O)OCH3), 3.22–3.34 (m, –NHCHCH2CH2CH2CH2–, CH3OCH2–), 3.52–4.12 (m, –NHCH(R)C(O)–, –OCH2CH2OCH2CH2OCH2C(O)NH–, –OCH2CH2OCH2CH2NH–, H-5, H-6), 4.54 (m, H-1), 5.02 (m, H-3), 5.15 (t, H-2), 5.37 (m, H-4), 7.14 (br, NH), 8.19 (s, NH), 8.79 (s, NH); FTIR (cm−1) = 3287, 3067, 2921, 2866, 1749, 1719, 1650, 1534, 1439, 1369, 1339, 1221, 1100, 928, 849.
General procedure for global deprotection.
The polypeptide was dissolved in dry methanol (100 mL methanol per gram of polypeptide), 50 mg sodium methanolate was then added and the reaction mixture was stirred at 25 °C. After 2 d, the solution was neutralized with acetic acid. The crude product was dissolved in methanol and purified by continuous ultrafiltration through a cellulose membrane with a 5 kDa molecular weight cut-off against a 10-fold amount of methanol. After evaporation of the solventin vacuo, the residue was redissolved in water and lyophilized yielding the unprotected polypeptide as a white, cotton-like solid.
Poly([Nε-(3,6,9-trioxa-decanoyl)-L-lysine]-co-[Nε-(8-(2,3,4,6-tetra-O-acetyl-α-D-mannopyranosyl)-1-thioxo-2-aza-5,8-dioxa-octyl)-L-lysine]-co-lysine).
1H NMR (CDCl3): δ/ppm = 1.08–1.98 (m, –NHCHCH2CH2CH2CH2–), 3.05 (m, –NHCHCH2CH2CH2CH2–), 3.22–5.92 (m, –NHCH(R)C(O)–, CH3OCH2CH2OCH2CH2OCH2C(O)NH–, –OCH2CH2O–CH2CH2NH–, H-1, H-2, H-3, H-4, H-5, H-6), 7.69 (br, NH), 8.53 (s, NH); FTIR (cm−1) = 3284, 3067, 2920, 2865, 1649, 1537, 1439, 1375, 1339, 1283, 1247, 1198, 1099, 934, 848. The 1H NMR and IR spectra of the corresponding glucose and galactose derivatives are very similar to those of the mannose derivatives due to poor resolution of the signals.
Labeling of the polymers with fluorescein-5-isothiocyanate.
In a typical experiment, the deprotected polypeptide (200 mg) and DIPEA (100 μL) were dissolved in anhydrous DMF (30 mL) under an N2 atmosphere. Fluorescein-5-isothiocyanate (60 mg, 0.15 mmol) was added and the reaction mixture was stirred protected from light for 2 d at 25 °C. After 1 d, more fluorescein-isothiocyanate (60 mg, 0.15 mmol) was added to complete the reaction. The DMF was removed under vacuum and the crude residue was redissolved in methanol (80 mL). Purification was carried out by ultrafiltration through a cellulose membrane (cut-off molecular weight 5 kDa) with 250 mL of a 1
:
1 mixture of 0.25 M aqueous sodium chloride and methanol, 250 mL of a 1
:
1 mixture of distilled water and methanol, and finally, 1 L of methanol. After evaporation of the solvent and dissolution of the residue in water the product was obtained after lyophilization as an orange, cotton-like solid.
Poly([Nε-(3,6,9-trioxa-decanoyl)-L-lysine]-co-[Nε-(8-α-D-mannopyranosyl)-1-thioxo-2-aza-5,8-dioxa-octyl)-L-lysine]-co-[Nε-(fluorescein-5-imino-thiocarbonyl)lysine]) (6a).
1H NMR (CDCl3): δ/ppm = 1.01–2.06 (m, –NHCHCH2CH2CH2CH2–), 3.22–5.33 (m, –NHCHCH2CH2CH2CH2–, –NHCH(R)C(O)–, CH3OCH2CH2OCH2CH2OCH2C(O)NH–, –CH2CH2OCH2CH2NH–, H-1, H-2, H-3, H-4, H-5, H-6), 7.15 (br, NH), 8.22 (br, NH); FTIR (cm−1) = 3284, 3068, 2921, 2865, 1647, 1537, 1454, 1338, 1284, 1259, 1199, 1095, 1024, 935, 849, 803. The 1H NMR and IR spectra of the corresponding glucose and galactose derivatives, 6b and 6c, were very similar to those of 6a.
Biochemical procedures
Isolation and activation of human T lymphocytes.
Buffy coat blood was obtained from a local blood center (Blutspendezentrale Saar-Pfalz gGmbH, Saarbrücken, Germany) and CD3+ T cells were enriched by using a RosetteSep Kit (StemCell Technologies, Grenoble, France) according to the manufacturer's instructions. Briefly, 0.5 mL RosetteSep antibody cocktail was added to 20 mL buffy coat blood and incubated at 25 °C for 20 min while gently shaking. Cells were isolated by centrifugation using a Pancoll human density gradient (PAN Biotech, Aidenbach, Germany). Remaining erythrocytes were lysed with 2 mL of an ammonium chloride-based lysing reagent (BD Pharm Lyse, BD Biosciences, Heidelberg, Germany). Afterward, enriched cells were resuspended in culture medium (RPMI 1640, PAN Biotech, supplemented with 10 wt% heat-inactivated FBS, PAN Biotech, and 1 wt% penicillin–streptomycin, Sigma-Aldrich, Steinheim, Germany). The purity of isolated T lymphocytes was consistently higher than 90% when analyzed by flow cytometry (FACSCalibur, BD Biosciences) with antibodies against human CD3 (AbD Serotec, Düsseldorf, Germany). Prior to experimental use, T lymphocytes were stimulated for at least 3 d using particles functionalized with antibodies against human CD2, CD3, and CD28 (MACS T Cell Activation/Expansion Kit, Miltenyi Biotec, Bergisch Gladbach, Germany). Expansion of T cells was achieved by adding 20 U mL−1 of recombinant human Interleukin-2 (PAN Biotech) and fresh culture medium every 3 to 4 d.
In vitro toxicology assay.
T lymphocytes were harvested from cultures and counted by using a cell counter (CASY Model TT, Innovatis, Mannheim, Germany). Subsequently, cells were inoculated at a concentration of 2.5 × 105cells per well in 100 μL fresh culture medium (supplemented RPMI 1640 without phenol red, PAN Biotech) into 96-well microtiter plates. Polypeptides were dissolved in pure water at a concentration of 2 mg mL−1. Triplicates of culture wells were subjected to 20 μL portions of solutions of polypeptides 6a, 6b or 6c and incubated at 37 °C in a humidified atmosphere containing 5 vol% CO2. Likewise, control cells were treated with 20 μL PBS (Gibco, Invitrogen, Darmstadt, Germany). Additionally, triplicate blanks contained only culture medium with polypeptides or PBS, respectively. Prior to use, 5 mg of a mixture of 2,3-bis(2-methoxy-4-nitro-5-sulfophenyl)-5-[(phenylamino)carbonyl]-2H-tetrazolium hydroxide, XTT, and 1 wt% phenazine methosulfate (PMS) (TOX-2, Sigma-Aldrich) were reconstituted with 5 mL RPMI 1640 without phenol red. After 18, 42 and 66 h, 24 μL of XTT–PMS solution was applied to the wells and cultures were incubated for 6 h. Subsequently, cultures were homogenized to completely disperse the XTT formazan. Finally, the absorbance was measured at 450 nm with a reference wavelength of 690 nm using a microplate reader (Spectra Rainbow Thermo, Tecan, Crailsheim, Germany).
Cell interaction test by flow cytometry.
T lymphocytes were inoculated at a concentration of 5 × 105cells per tube in 100 μL of fresh culture medium. Duplicates of T cells were subjected to 20 μg (10 μL solution) of polypeptides 6a, 6b or 6c and incubated at 4 °C or 37 °C for 2, 4 or 8 h. Likewise, control cells were treated with 10 μL PBS. Cells were washed twice with 1 mL ice-cold PBS, resuspended in ice-cold 500 μL PBS and kept on ice until analysis by flow cytometry. Fluorescence intensity of 10
000 events per sample was measured by using a FACSCalibur cytometer (BD Biosciences) and CellQuest Pro software (version 5.1.1, BD Biosciences). Fluorescence intensities of gated T cell populations are represented by histograms and geometric means calculated by CellQuest Pro.
Microscopy
.
For fluorescence microscopy, samples containing 2 × 106T cells in 800 μL culture medium were subjected to 80 μg (40 μL solution) of polypeptides and incubated at 4 °C or 37 °C for 6 h. Subsequently, T cells were washed twice with 1 mL ice-cold PBS. For fixation, sedimented cells were resuspended in 400 μL 4 wt% paraformaldehyde in PBS and incubated protected from light at 25 °C for 10 min. Afterward, cells were washed twice with 1 mL ice-cold PBS. Washing buffer was discarded and T cells were resuspended in 20 μL polyvinyl alcohol-based anti-fading mounting medium consisting of 2.5 wt% 1,4-diazabicyclo-[2,2,2]-octane (DABCO, Roth, Karlsruhe, Germany), 22.7 wt% Mowiol 4-88 (Roth), 9.1 wt% glycerol (Merck, Darmstadt, Germany), 1 μg mL−14′,6-diamidino-2-phenylindole dihydrochloride (DAPI, Sigma-Aldrich) and 0.1 M Tris–HCl pH 8.5 (Fluka Sigma-Aldrich). The mounting medium was allowed to harden overnight and protected from light at 4 °C. Fluorescence microscopy was performed using an IX71 inverted microscope (Olympus, Hamburg, Germany) equipped with U-MNU2 and U-MWIBA2 filters. DNA-intercalating DAPI was excited at wavelengths of 360–370 nm and polypeptides were excited at wavelengths of 460–490 nm. Fluorescence, emitted by DAPI–DNA complexes at wavelengths above 420 nm or by polypeptides at 515–550 nm, was recorded through a 60× PlanApo N oil-immersion objective (Olympus) with a numerical aperture of 1.42 by a digital camera (F-View II, Olympus) attached to the microscope. The camera was controlled by cellp imaging software (version 2.8, Olympus). All images were digitalized using the same conditions (automatic gain disabled, exposure time = 200 ms) to compare the fluorescence intensities, whereas the recording of DAPI-stained cell nuclei was automatically adjusted by cellp (Olympus soft imaging solutions). Image overlays illustrating blue and green fluorescence were created via cellp imaging software.
Results and discussion
Our intention was to synthesize glycosylated polypeptides soluble in water. They should be neutral to avoid unspecific interactions with cells. Because polyglutamine of a low degree of polymerization (DP < 20) and copolymers with polyglutamine blocks not longer than twenty are known to be water-soluble,36,37 we synthesized a statistical copolymer poly(glutamine-co-lysine) by copolymerization of a mixture of Nε-trityl-glutamine-NCA and TFA-lysine-NCA (molar ratio 5
:
1) using NEt3 as the catalyst.21 The lysine moieties were set aside for the attachment of carbohydrate moieties at the ε-amino groups by thiourea linkages. After deprotection of the copolymer, the compound was found to be totally insoluble in water as well as in other solvents. Therefore, all attempts to attach carbohydrate moieties were not successful.
Because we were not aware of any other neutral water-soluble homopolypeptides consisting of natural amino acids, we chose non-natural poly(Nε-(3,6,9-trioxo-decanoyl)-L-lysine) as the backbone, which is known to be soluble in water.25Statistical copolymers 4 of Nε-(3,6,9-trioxo-decanoyl)-L-lysine and a sugar-appended lysine were synthesized by NCA copolymerization of the corresponding NCAs. The sugar-appended lysine-NCA 3 was synthesized in three steps according to Fig. 1 with glycosylation of 2-(2-isothiocyanatoethoxy)ethanol by 2,3,4,6-tetra-O-acetylglycopyranosyl-1-trichloroacetimidate 1 and subsequent coupling of the isothiocyanate group of the products to the ε-amino group of Z-lysine. In all three cases, we observed stereoselective glycosylationtrans to the 2-O-acetyl groups according to the well-known 1,2-trans directing effect of acetyl neighbour groups leading to the α-mannoside, the β-glucoside and β-galactoside, respectively.32 The 1H NMR spectra were consistent with those of related glycosides38,39 and show large coupling constants between H-1 and H-2, 3JHH = 8.0 Hz, characteristic of both, the β-glucoside and the β-galactoside. Finally, direct conversion of the Z-protected glycosylated amino acid 2 to the NCA with dichloromethyl methyl ether was performed.40 The results of the NCA copolymerizations are summarized in Table 1. 1H NMR spectra of both the monomers 3a–c and the corresponding copolymers 4a–c are shown in the ESI†.
Table 1
Copolymerization of Nε-(3,6,9-trioxo-decanoyl)-L-lysine-NCA with sugar-appended L-lysine-NCAs 3a, 3b, or 3c (molar ratio 9
:
1) in DMF solution leading to polymers 4a, 4b and 4c, respectively
Both employed catalysts, NEt3 and the Ni0 2,2′-bipyridine 1,5-cyclooctadiene complex, furnished the polypeptides in high yields and molecular weights of 30
000 to 50
000 g mol−1. Higher molecular weights were obtained if THF was used as the reaction medium instead of DMF. The low polydispersity indices (PDIs) of around 1.2 obtained with the Ni0 catalysts are typical for living polymerizations. Therefore, they are well-suited for the synthesis of glycopeptides. These PDIs were significantly lower than the ones obtained with NEt3.
Furthermore, it was desirable to attach fluorescent labels, such as fluorescein, to the glycopolypeptides to allow the detection of any interaction with biological systems. Because synthesis and polymerization of lysine–NCA conjugates with fluorescein were rather difficult, it was decided to synthesize terpolymers with a small amount of TFA protected lysine, which would allow the subsequent attachment of fluorescein in a polymer-analogous reaction. Terpolymerizations of Nε-(3,6,9-trioxo-decanoyl)-L-lysine-NCA with glycosylated L-lysine-NCAs and TFA-lysine-NCA performed well giving rise to soluble polymers in good yields and high molecular weights and narrow PDIs, listed in Table 2. The Ni0 catalysts were superior to NEt3 in terms of the yields, but not in terms of the PDIs. Again, the compositions of the synthesized polymers were identical with the employed feed ratios of the three monomers, allowing for the predictable synthesis of well-defined polymers.
Table 2 Terpolymerization of Nε-(3,6,9-trioxo-decanoyl)-L-lysine-NCA with sugar-appended L-lysine-NCAs 3a, 3b, or 3c (sugar content 10 mol%) and TFA-lysine-NCA (molar ratio 16
:
2
:
1) in DMF solution leading to polymers 5a, 5b, and 5c, and to final polymers 6a, 6b, 6c, respectively
After deprotection of both the sugar residues and the lysine units with NaOCH3, fluorescein isothiocyanate (FITC) was coupled almost quantitatively to the ε-amino groups of the lysine units. The resulting labeled glycopolypeptides 6a–c as well as 6u were soluble in water and showed a bright fluorescence at λ = 520 nm in FBS buffer solution at pH = 7.5. The fluorescence intensities are given in Table 2. According to their IR spectra with typical bands at 1535 and 1650 cm−1 they have α-helical structures.25
The in vitro toxicities of polymers 6a–c as well as 6u were tested with human T lymphocytes using the XTT cell viability assay.41 It was found that natural cell viability remains preserved for polymer concentrations of up to 0.33 mg mL−1, shown in Fig. 2. Also, microscopic investigations revealed that T cells did not change their appearances within 3 d after exposure to solutions of these polymers. From these observations, we conclude that polymers 6a–c are sufficiently biocompatible.
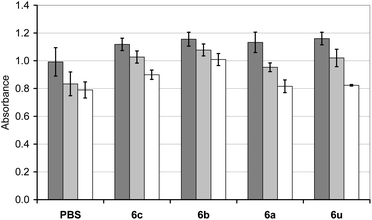 |
| Fig. 2 Viabilities of human T lymphocytes (concentration 0.33 mg mL−1) after a 24 h (dark grey bar), 48 h (pale grey bar) and 72 h (white bar) incubation with polymer 6c. | |
The interactions of polymers 6a–c with human T lymphocytes were investigated by flow cytometry (FCM). The fluorescence intensity distributions only showed a pronounced shift to higher fluorescence intensities for the galactosylated polymer 6c, especially for the incubations at 37 °C, shown in Fig. 3. The uptakes of the fluorescent polymers (Fig. 4) were quantified by calculating the geometric mean of fluorescence intensity over the cell populations and normalized on their different fluorescence intensities taken from Table 2. While there was only little uptake of the fluorescent unsubstituted polymer 6u and the glucose polymer 6b, the mannose polymer 6a and the galactose polymer 6c were readily taken up, especially at 37 °C. Interestingly, this uptake was only observed with activated T lymphocytes, which means that activation of the cells should lead to expression of galactose receptors incorporated in the cell walls. These receptors might be related to L-selectin, which is known to bind the galactose-containing tetrasaccharide sialyl Lewis X and galactose clusters.42,43 The glycoprotein L-selectin (CD62L) is expressed on the surface of T lymphocytes and plays a crucial role during T cell recirculation.44 By binding to its ligands, CD62L initiates the extravasation of T lymphocytes into peripheral lymphoid tissues.
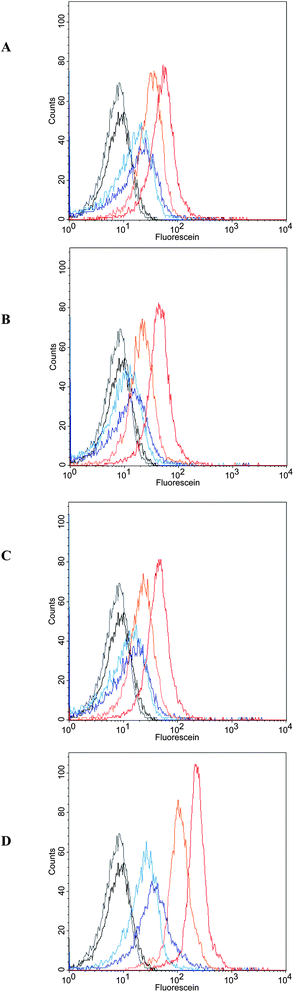 |
| Fig. 3
FCM fluorescence intensity distributions of T lymphocytes at 4 °C (black) and at 37 °C (grey), after incubation at 4 °C (pale blue, 2h; dark blue, 8h) and 37 °C (pale orange, 2h; dark orange, 8h) with polypeptides (A) unsubstituted 6u, (B) mannosylated 6a, (C) glucosylated 6b, and (D) galactosylated 6c. | |
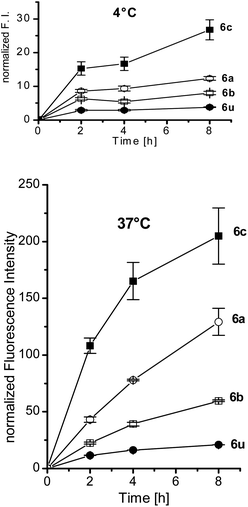 |
| Fig. 4 Uptake of the glycopolymers 6a–c and the unsubstituted polymer 6u by T cells at 4 °C and 37 °C, as determined from the geometric mean of FCM fluorescence intensities, normalized by the fluorescence intensity of the polymer. | |
However, during activation of T lymphocytes, CD62L is down-regulated,45 which is in contrast to our finding of solely activated T lymphocytes taking up polymer 6c significantly. Consequently, L-selectin might not be the desired receptor. Another receptor candidate might be among the members of the galectin family. These mammal lectins exhibit affinity for β-galactosides.46 Galectins can exist as dimers or even pentamers.47 Therefore, extracellularly secreted galectins are able to form lattices with glycoprotein receptors on the cell surface. This process enables the cell to modulate various receptor functions.48 Our galactosylated polymer 6c might bind multivalently to these extracellular galectin lattices. This hypothesis is supported by the experimental results of both galectin-1 and galectin-3 being up-regulated upon T cell activation.49,50
The pronounced temperature dependence of the interaction of the galactosylated polymer with the T lymphocytes might be rationalized by the higher cell membrane mobility at the elevated temperature (37 °C) which should allow receptor-mediated endocytosis, which should lead to an enrichment of the fluorescent polymer 6c within the cells. Fluorescence microscopy studies of the T cells incubated with solutions (conc. 0.10 mg mL−1) of polymers 6a, 6b as well as 6c for 6 h, shown in Fig. 5, indeed demonstrated that only the galactosylated polymer 6c showed significant interactions with the T cells. Most cells took over the green fluorescence of fluorescein. The picture also provides evidence for the fact that the polymer 6c is not only adsorbed at the cell wall but internalized into the cytoplasm of the cell, while the nuclei of the cells stained in blue remained unaffected. This finding is clearly indicative for the uptake of galactosylated polymer 6c within the T lymphocytes by endocytosis. Addition of both galactose and lactose (concentrations up to 0.1 mol L−1) could not inhibit the uptake of polymer 6c in these cells.
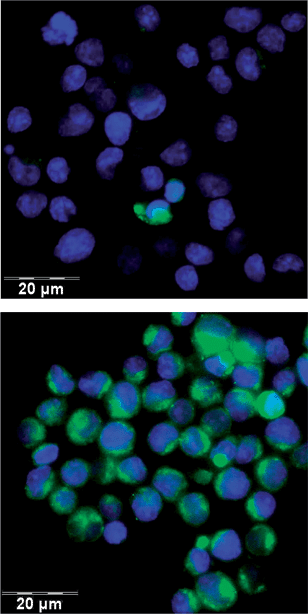 |
| Fig. 5 Imaging of fluorescein (green) in the T lymphocytes by fluorescence microscopy after 6 h incubation at 4 °C (upper image) and 37 °C (lower image) with the galactosylated polymer 6c. The cell nuclei were stained blue by DAPI. | |
Conclusions
PEGylated polylysine is a readily available and water-soluble scaffold for the design of polymeric neoglycoconjugates. PEGylated polylysine with conjugated galactose residues allows the recognition of activated T lymphocytes. It is potentially useful for targeting covalently attached active substances, e.g., drugs, into lymphocytes.
Acknowledgements
This work was funded by the project, “Polymere für die zeitkontrollierte Medikamentenfreisetzung in der Immuntherapie” Saarbridge by Saarland Ministerium für Wirtschaft und Wissenschaft.
Notes and references
- H. J. Gabius, H. C. Siebert, S. Andre, J. Jimenez-Barbero and H. Rudiger, ChemBioChem, 2004, 5, 741–764 CrossRef.
- C. R. Bertozzi and L. L. Kiessling, Science, 2001, 291, 2357–2364 CrossRef CAS.
- K. N. Houk, A. G. Leach, S. P. Kim and X. Y. Zhang, Angew. Chem., Int. Ed., 2003, 42, 4872–4897 CrossRef CAS.
- M. Mammen, S.-K. Chio and G. M. Whitesides, Angew. Chem., Int. Ed., 1998, 37, 2755–2794 CrossRef CAS.
- D. Rabuka, R. Parthasarathy, G. S. Lee, X. Chen, J. T. Groves and C. R. Bertozzi, J. Am. Chem. Soc., 2007, 129, 5462–5471 CrossRef CAS.
- D. Rabuka, M. B. Forstner, J. T. Groves and C. R. Bertozzi, J. Am. Chem. Soc., 2008, 130, 5947–5953 CrossRef CAS.
- X. Chen, U. C. Tam, J. L. Czlapinski, G. S. Lee, D. Rabuka, A. Zettl and C. R. Bertozzi, J. Am. Chem. Soc., 2006, 128, 6292–6293 CrossRef CAS.
- S. Andre, H. Kaltner, T. Furuike, S. I. Nishimura and H. J. Gabius, Bioconjugate Chem., 2004, 15, 87–98 CrossRef CAS.
- M. Gómez-García, J. M. Benito, D. Rodríguez-Lucena, J.-X. Yu, K. Chmurski, C. Ortiz-Mellet, R. G. Gallego, A. Maestre, J. Defaye and J. M. G. Fernández, J. Am. Chem. Soc., 2005, 7970–7971 CrossRef.
- F. Ortega-Caballero, J. J. Gimenez-Martinez, L. Garcia-Fuentes, E. Ortiz-Salmeron, F. Santoyo-Gonzalez and A. Vargas-Berenguel, J. Org. Chem., 2001, 66, 7786–7795 CrossRef CAS.
- P. R. Ashton, S. E. Boyd, C. L. Brown, S. A. Nepogodiev, E. W. Meijer, H. W. I. Peerlings and J. F. Stoddart, Chem.–Eur. J., 1997, 3, 974–984 CrossRef CAS.
- C. M. Lehr, J. Controlled Release, 2000, 65, 19–29 CrossRef CAS.
- C. Bies, C. M. Lehr and J. F. Woodley, Adv. Drug Delivery Rev., 2004, 56, 425–435 CrossRef CAS.
- D. Neumann, C. M. Lehr, H. P. Lenhof and O. Kohlbacher, Adv. Drug Delivery Rev., 2004, 56, 437–457 CrossRef CAS.
- M. Gomez-Garcia, J. M. Benito, R. Gutierrez-Gallego, A. Maestre, C. O. Mellet, J. M. G. Fernandez and J. L. J. Blanco, Org. Biomol. Chem., 2010, 8, 1849–1860 CAS.
- A. Vargas-Berenguel, F. Ortega-Caballero and J. M. Casas-Solvas, Mini-Rev. Org. Chem., 2007, 4, 1–14 CrossRef CAS.
- J. Kim, Y. Ahn, K. M. Park, D.-W. Lee and K. Kim, Chem.–Eur. J., 2010, 16, 12168–12173 CrossRef CAS.
- M. Ortega-Munoz, J. Morales-Sanfrutos, F. Perez-Balderas, F. Hernandez-Mateo, M. D. Giron-Gonzalez, N. Sevillano-Tripero, R. Salto-Gonzalez and F. Santoyo-Gonzalez, Org. Biomol. Chem., 2007, 5, 2291–2301 CAS.
- Y. Oda, N. Kobayashi, T. Yamanoi, K. Katsuraya, K. Takahashi and K. Hattori, Med. Chem., 2008, 4, 244–255 CrossRef CAS.
- S. Matsumura, S. Sakamoto, A. Ueno and H. Mihara, Chem.–Eur.
J., 2000, 6, 1781–1788 CrossRef CAS.
- H. R. Kricheldorf, Angew. Chem., Int. Ed., 2006, 45, 5752–5784 CrossRef CAS.
- G. J. M. Habraken, K. H. R. M. Wilsens, C. E. Koning and A. Heise, Polym. Chem., 2011, 2, 1322–1330 RSC.
- J. N. Cha, G. D. Stucky, D. E. Morse and T. J. Deming, Nature, 2000, 403, 289–292 CrossRef CAS.
- T. J. Deming, Prog. Polym. Sci., 2007, 32, 858–875 CrossRef CAS.
- M. Yu, A. P. Nowak, T. J. Deming and D. J. Pochan, J. Am. Chem. Soc., 1999, 121, 12210–12211 CrossRef CAS.
- R. Wang, N. Xu, F.-S. Du and Z.-C. Li, Chem. Commun., 2010, 46, 3902–3904 RSC.
- J. R. Kramer and T. J. Deming, J. Am. Chem. Soc., 2010, 132, 15068–15071 CrossRef CAS.
- D. E. Owens and N. A. Peppas, Int. J. Pharm., 2006, 307, 93–102 CrossRef CAS.
- U. Steinfeld, C. Pauli, N. Kaltz, C. Bergemann and H.-H. Lee, Int. J. Pharm., 2006, 311, 229–236 CrossRef CAS.
- C. Guillermain and B. Gallot, Macromol. Chem. Phys., 2002, 203, 1346–1356 CrossRef CAS.
- D. S. Poché, M. J. Moore and J. L. Bowles, Synth. Commun., 1999, 29, 843–854 CrossRef.
- R. R. Schmidt and W. Kinzy, Adv. Carbohydr. Chem. Biochem., 1994, 50, 21–123 CrossRef CAS.
- J. M. de la Fuente and S. Penades, Tetrahedron: Asymmetry, 2002, 13, 1879–1888 CrossRef CAS.
- H. Tajima and G. Li, Synlett, 1997, 773–774 CrossRef CAS.
- J. J. Eisch, A. M. Piotrowski, K. I. Han, C. Kruger and Y. H. Tsay, Organometallics, 1985, 4, 224–231 CrossRef CAS.
- S. Chen and R. Wetzel, Protein Sci., 2001, 10, 887–891 CrossRef CAS.
- E. L. Altschuler, N. V. Hud, J. A. Mazrimas and B. Rupp, J. Pept. Res., 1997, 50, 73–75 CrossRef CAS.
- E. K. Woller and M. J. Cloninger, Org. Lett., 2002, 4, 7–10 CrossRef CAS.
- Z.-J. Yin, Q. Li, X.-B. Meng and Z.-J. Li, Carbohydr. Res., 2007, 342, 2729–2734 CrossRef CAS.
- K. Poduska and H. Gross, Chem. Ber., 1961, 49, 527–537 CrossRef CAS.
- D. Scudiero, R. Shoemaker, K. Paull, A. Monks, S. Tierney, T. Nofziger, M. Currens, D. Seniff and M. Boyd, Cancer Res., 1988, 48, 4827–4833 CAS.
- W. S. Somers, J. Tang, G. D. Shaw and R. T. Camphausen, Cell, 2000, 103, 467–479 CrossRef CAS.
- I. Papp, J. Dernedde, S. Enders and R. Haag, Chem. Commun., 2008, 5851–5853 RSC.
- T. A. Springer, Cell, 1994, 76, 301–314 CrossRef CAS.
- C. C. Chao, R. Jensen and M. O. Dailey, J. Immunol., 1997, 159, 1686–1694 CAS.
- S. H. Barondes, V. Castronovo, D. N. W. Cooper, R. D. Cummings, K. Drickamer, T. Feizi, M. A. Gitt, J. Hirabayashi, C. Hughes, K. Kasai, H. Leffler, F. T. Liu, R. Lotan, A. M. Mercurio, M. Monsigny, S. Pillai, F. Poirer, A. Raz, P. W. J. Rigby, J. M. Rini and J. L. Wang, Cell, 1994, 76, 597–598 CrossRef CAS.
-
F. T. Liu and G. A. Rabinovich, in Year in Immunology 2, 2010, pp. 158–182 Search PubMed.
- O. B. Garner and L. G. Baum, Biochem. Soc. Trans., 2008, 36, 1472–1477 CrossRef CAS.
- M. B. Fuertes, L. L. Molinero, M. A. Toscano, J. M. Ilarregui, N. Rubinstein, L. Fainboim, N. W. Zwirner and G. A. Rabinovich, Mol. Cell. Biochem., 2004, 267, 177–185 CrossRef CAS.
- H. G. Joo, P. S. Goedegebuure, N. Sadanaga, M. Nagoshi, W. von Bernstorff and T. J. Eberlein, J. Leukocyte Biol., 2001, 69, 555–564 CAS.
Footnote |
† Electronic supplementary information (ESI) available. See DOI: 10.1039/c1py00187f |
|
This journal is © The Royal Society of Chemistry 2011 |
Click here to see how this site uses Cookies. View our privacy policy here.