DOI:
10.1039/C1PY00102G
(Paper)
Polym. Chem., 2011,
2, 1671-1677
Optimizing the generation of narrow polydispersity ‘arm-first’ star polymers made using RAFT polymerization†‡
Received
8th March 2011
, Accepted 30th March 2011
First published on 20th April 2011
Abstract
In this article, the synthesis of well-defined, narrow polydispersity (PDI < 1.2) star polymersviaRAFT polymerisation is detailed. An ‘arm-first’ method is described using a crosslinked nanogel core. The synthetic conditions and variables were thoroughly investigated and optimised so that nearly quantitative arm incorporation was found to occur. The parameters studied included polymerization time, the arm molecular weight, and the nature of the solvent and cross-linker.
Introduction
Star polymers have attracted a lot of research interest, since they have unique properties in terms of the relationship between arm number, arm molecular weight and solvent viscosity.1–4 In addition, star polymers can be seen as well-defined nanoparticles for applications in nanomedicine,5–8catalyst9 and in photonics,10,11 for example. Well-defined star polymers can be made using ionic polymerisation techniques that are ideally suited to precision syntheses as bimolecular termination (especially coupling) is not present and therefore star–star coupling is negated.12–14
Since the discovery of living radical techniques, all three main synthetic methods, viz., ATRP/metal mediated,15–21 RAFT/MADIX22–29 and NMP,30 have been exploited to make star shaped polymers. In all syntheses involving radical intermediates termination reactions inevitably occur and therefore optimising reaction conditions to minimize coupling becomes an important consideration for generating narrow polydispersities (an issue of particular importance in nanomedicine applications).5,31,32 This is an unavoidable problem when a core-first approach to star synthesis is adopted. To obviate this problem then an ‘arm-first’ method can be implemented, however, this approach can be beset with problems, exacerbated at higher arm molecular weights and arm numbers, as steric effects come into play, hindering quantitative yields.33
In this current work, we focus on RAFT polymerisation, where radical coupling is known to be a potentially serious problem. This star–star coupling problem can be minimized using a Z-approach rather than a R-approach to arm growth.24 However, the Z-approach has its own drawbacks as the RAFT chain growth process is vulnerable to solvent effects, as was shown in theoretical and experimental work.34–36
The synthesis of star polymers has featured as a major research effort in both of our groups based at UNSW37–44 and Warwick University,45–48 with substantial work on both metal mediated and RAFT approaches to unique macromolecules with functional cores and biodegradability with particular focus on stars for biotechnological applications.
In this paper, we explore the ‘arm-first’ approach as an alternative to ‘core-first’ for the synthesis of star polymers. The ‘arms first’ route to stars is becoming more widely used with ‘click chemistry’ increasing in popularity, and both Huisgen cycloaddition and thiol–ene have been extensively explored for this purpose.49–53 In principle, a simpler approach to ‘arm-first’ stars is to use a microgel or nanogel core built up using a cross-linker with residual vinyl groups for subsequent arm attachment, an approach that dates back to an early anionic polymerisation age. This approach has since been applied to living radical polymerisation, and has been optimised for ATRP by Gao and Matyjaszewski17 and in recent work by Qiao and co-workers who report very narrow polydispersities using ATRP.54–56 In RAFT polymerisation, however, whilst the arm-first, crosslinked core, approach has been reported, narrow polydispersities have remained elusive as exemplified in a recent paper we published on biodegradable, functional stars.57
We have optimized a simple RAFT approach to stars to yield narrow polydispersity stars—we believe to be the most uniform products made yet, reported for RAFT without recourse to ‘click’ chemistry. The versatility of our approach is demonstrated using a variety of polymers, viz. poly(oligoethylene glycol methyl ether acrylate), poly(OEG-A), poly(tert-butyl acrylate), poly(tert-Bu A), and poly(N-isopropyl acrylamide), poly(NIPAAm).
Experimental
Materials
Oligo(ethylene glycol) methyl ether acrylate (OEG-A, Mw = 480 g mol−1, 99%, Sigma-Aldrich), diethylene glycol acrylate (DEG-A) and tert-butyl acrylate (tert-Bu A) were de-inhibited via a column of activated basic alumina. De-inhibited OEG-A, tert-Bu A and DEG-A were stored at −18 °C. N-Isopropyl acrylamide (NIPAAm) was recrystallised from hexane. The initiator, 2,2′-azobisisobutyronitrile (AIBN), was crystallized twice from methanol. High purity N2 (Linde gases) was used for reaction solution purging. All the other chemical reactants were purchased from Sigma-Aldrich, supplied at the highest purity available.
Analyses
Gel permeation chromatography (GPC) measurements.
DMAc
GPC analyses of the polymers were performed in N,N-dimethylacetamide [DMAc; 0.03% w/v LiBr, 0.05% 2,6-di-butyl-4-methylphenol (BHT)] at 50 °C (flow rate = 1 mL min−1) using a Shimadzu modular system comprised of an SIL-10AD auto-injector, a PL 5.0 mm bead-size guard column (50 × 7.8 mm) followed by four linear PL (Styragel) columns (105, 104, 103, and 500 Å) and an RID-10A differential refractive-index detector. Calibration was achieved with commercial polystyrene standards ranging from 500 to 106 g mol−1. THF GPC analyses were performed on a Shimadzu modular system, comprising an auto-injector and a Polymer Laboratories 5.0 mm bead size guard column (50 × 7.5 mm), followed by three linear PL columns and a differential refractive index detector using THF as the eluent at 40 °C with a flow rate of 1 mL min−1. The GPC system was calibrated using linear polystyrene standards.
Nuclear Magnetic Resonance (NMR).
Structures of the synthesized compounds were analysed by 1H NMR spectroscopy using a Bruker DPX 300 spectrometer at 300 MHz for hydrogen nuclei and 75 MHz for carbon nuclei.
Dynamic light scattering (DLS).
DLS measurements were performed using a Malvern Zetasizer Nano Series running DTS software and using a 4 mW He–Ne laser operating at a wavelength of 633 nm and an avalanche photodiode (APD) detector. The scattered light was detected at an angle of 173°. The temperature was stabilized to ±0.1 °C of the set temperature. To reduce the influence of larger aggregates the number-average hydrodynamic particle size is reported. The polydispersity index (PDI) is used to describe the width of the particle size distribution, as calculated from the DTS software using a cumulants analysis of the measured intensity autocorrelation function; it is related to the standard deviation of the hypothetical Gaussian distribution (i.e. PDI = s2/ZD2, where s is the standard deviation and ZD is the Z average mean size).
Transmission electron microscopy (TEM).
The sizes and morphologies of the star polymers were observed using a transmission electron microscopy JEOL1400 TEM at an accelerating voltage of 100 kV. The particles were dispersed in water (5 mg mL−1) and deposited onto 200 mesh, holey film, copper grid (ProSciTech).
Syntheses
Synthesis of RAFT agents.
The synthesis of 3-(benzylsulfanylthiocarbonylsulfanyl)-propionic acid (BSTP, 1, Scheme 1) has been described earlier.58
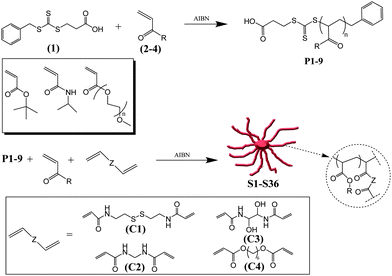 |
| Scheme 1 Schematic representation for the synthesis of star polymersvia arm first approach using different cross-linkers (C1–C4). | |
Synthesis of arm homopolymer: oligoethylene glycol-acrylate (OEG-A) arm.
Three different molecular weights were targeted, and a summary of the reagents used is given in Table S1 in the ESI†. Briefly, OEG-A480, AIBN, 3-(benzylsulfanylthiocarbonylsulfanyl)-propionic acid and acetonitrile were placed into a 50 mL round bottom flask, equipped with a magnetic stirrer bar. The reaction mixture, maintained at 0 °C, was degassed by purging with nitrogen for 20 minutes. The degassed solution was stirred at 70 °C for 6 hours. At the end of the polymerization, the solution was placed in an ice bath and an aliquot was sampled for GPC and 1H NMR analyses. The remainder of the reaction mixture was purified; acetonitrile was removed using a rotary evaporator under vacuum, and the concentrated solution was dialyzed against methanol to remove any traces of monomer, using a membrane with a cut-off of 3500 Da.
A similar procedure was performed for the homopolymerization of tert-butyl acrylate (tert-Bu A) and N-isopropyl acrylamide (NIPAAm) and the copolymerization of DEG-A and OEG-A (see the ESI† for more details).
Synthesis of star polymersvia an arm first methodology.
A general procedure is provided, with quantities used given in Table S2 in the ESI†. Polymer, or copolymer, was introduced into a vial equipped with a magnetic stirrer, with AIBN and toluene. Cross-linkers (N,N′-bis(acryloyl)cystamine (C1), N,N-methylene bisacrylamide (C2), 1,2-dihydroxyethylene-bis-acrylamide (C3) and 1,6-hexanediol diacrylate (C4)) were added, and the vials were sealed and purged under nitrogen, at 0 °C, for 20 minutes. The reaction vials were placed in an oil bath at 70 °C for 24 hours. At the end of the polymerizations, the polymers were sampled for 1H NMR and GPC analyses.
Degradation of disulfide cores using tris(n-butyl phosphine) or dimethylphenyl phosphine.
The disulfide core was cleaved in the presence of dimethylphenyl phosphine (DMPP) or tris(n-butyl phosphine) at ambient temperature to yield arms. The reactions were performed using the following general procedure: star polymers (100 mg) obtained using N,N′-bis(acryloyl)cystamine as the cross-linker were mixed with DMAc (1 mL). The solution was purged using nitrogen. DMPP or tris(n-butyl phosphine) (25 microlitres) was added to the solution. The reaction occurred at room temperature for 6 hours, before analysis by DMAc GPC. DMPP and tris(n-butyl phosphine) give similar results.
Results and discussion
Poly(OEG-A) (2), poly(tert-Bu A) (3) and poly(NIPAAm) (4) arms were prepared viaRAFT polymerization in the presence of 3-(benzylsulfanylthiocarbonylsulfanyl)-propionic acid (BSPA) (1) as the RAFT agent and AIBN as the initiator at 70 °C. Three different polymer arms, with molecular weights from 5000 to 20
000 g mol−1 and PDIs < 1.20, were obtained for each polymer family (Table 1). After purification, the presence of RAFT end groups was confirmed by 1H NMR spectroscopy and by UV-visible spectroscopy. The RAFT end-group functionality for these polymers was determined to be greater than 95% by UV-visible spectroscopy (λ = 305 nm), using the following equation: RAFT end-group Functionality = (Abs305/ε305)/[polymer]0, where Abs305, ε305 and [polymer]0 correspond to the absorbance, extinction coefficient at 305 nm (ε305 = 15
700/mol cm−1 (ref. 58)) and polymer concentration, respectively. Subsequently, the linear polymers were chain extended in the presence of a cross-linking molecule, AIBN and a low concentration of monomer, with a [RAFT]
:
[AIBN]
:
[monomer]
:
[cross-linker] ratio = 1.0
:
0.3
:
10.0
:
X, with X = 2, 4, 6, 8, 10, 12 and 16 in different solvents (DMF, acetonitrile and toluene) for 6, 24 and 48 h (Scheme 1). After chain extension, the star polymers were analysed by GPC and by 1H NMR spectroscopy.
Table 1 Summary of the polymers used in this study
Polymers
|
M
n
a/g mol−1 (Theoretical) |
M
n/g mol−1 (1H NMR)b |
M
n (GPC)/g mol−1 |
PDI (GPC) |
RAFT functionalityc |
Theoretical molecular weights calculated by the following equation: Mn = ([M]0/[RAFT]0) × αM × MwM + MwRAFT, [M]0, [RAFT]0, αM, MwM and MwRAFT correspond to the initial monomer concentration, initial RAFT agent concentration, monomer conversion, molar mass of the monomer and RAFT agent, respectively.
Molecular weighs determined by 1H NMR using the aromatic group of RAFT agent as the reference.
RAFT functionality was calculated by the following equation: RAFT end-group Functionality = (Abs305/ε305)/[polymer]0, where Abs305, ε305 and [polymer]0 correspond to the absorbance, extinction coefficient at 305 nm (ε305 = 15 700/mol cm−1) and polymer concentration.
|
Poly(OEG-A)–P1 |
8500 |
8000 |
7000 |
1.12 |
>95% |
Poly(OEG-A)–P2 |
11 500 |
12 000 |
11 500 |
1.18 |
— |
Poly(OEG-A)–P3 |
21 000 |
20 000 |
18 500 |
1.18 |
— |
Poly(tert-Bu A)–P4 |
8500 |
7800 |
8000 |
1.12 |
>95% |
Poly(tert-Bu A)–P5 |
14 000 |
14 000 |
14 500 |
1.14 |
— |
Poly(tert-Bu A)–P6 |
18 000 |
17 500 |
18 000 |
1.08 |
— |
Poly(NIPAAm)–P7 |
7500 |
8200 |
8500 |
1.08 |
>95% |
Poly(NIPAAm)–P8 |
13 000 |
14 000 |
13 500 |
1.09 |
— |
Copoly(OEG-A-co-DEG-A)–P9 |
18 500 |
18 000 |
17 000 |
1.20 |
>95% |
Effect of the solvent on the formation of star formation
To investigate the influence of solvent type, we worked at a fixed optimal reactant ratio, as we determined and published previously,57 namely, a [RAFT]0
:
[monomer]0
:
[cross-linker]0 ratio equal to 1
:
10
:
8. Three different solvents (DMF, acetonitrile and toluene) were tested using poly(OEG-A) arms in the presence of N,N′-bis(acryloyl)cystamine (C1) and 1,6-hexanediol diacrylate (C4). 1,6-Hexanediol diacrylate (C4) is perfectly soluble in these three solvents, while N,N′-bis(acryloyl)cystamine is partially soluble in toluene (35 mg mL−1) but fully soluble in DMF and acetonitrile. Star formation was observed for all soluble systems with the product star displaying polydispersities greater than 1.5, consistent with previous published data using RAFT polymerization.57 In contrast, when the core crosslinking was achieved with N,N′-bis(acryloyl)cystamine in toluene, well-defined star polymers were obtained with very narrow molecular weight distributions (low PDI < 1.2) and an excellent incorporation of the arms in the star structures (greater than 90%, determined via deconvulation of the GPC traces, see Fig. 1A). We hypothesize that this high incorporation of arms into the star structures for N,N′-bis(acryloyl)cystamine can be attributed to the low solubility (poor swelling) of the nascent crosslinked core in the poor solvent medium leading to some nano-phase separation inducing compartmentalization of the polymerization. Higher molecular weight polymers poly(OEG-A)-P2 (11
500 g mol−1) and poly(OEG-A)-P3 (18
500 g mol−1) were also used for star syntheses, with similar excellent results. These promising initial results motivated us to probe the experimental conditions to find optimum conditions for the synthesis of star polymers, using this RAFT, arm-first approach.
![GPC traces of different star polymers obtained by reversible addition fragmentation transfer (RAFT). Star polymers obtained using N,N′-bis(acryloyl)cystamine as the cross-linker: (A) in toluene, (B) in DMF, (C) in acetonitrile and (D) arm polymer used in this study (Mn, arm = 7000 g mol−1) before crosslinking. Experimental condition: [RAFT-polymer]0 : [monomer]0 : [cross-linker]0 ratio equal to 1 : 10 : 8 for 24 h at 70 °C.](/image/article/2011/PY/c1py00102g/c1py00102g-f1.gif) |
| Fig. 1
GPC traces of different star polymers obtained by reversible addition fragmentation transfer (RAFT). Star polymers obtained using N,N′-bis(acryloyl)cystamine as the cross-linker: (A) in toluene, (B) in DMF, (C) in acetonitrile and (D) arm polymer used in this study (Mn, arm = 7000 g mol−1) before crosslinking. Experimental condition: [RAFT-polymer]0 : [monomer]0 : [cross-linker]0 ratio equal to 1 : 10 : 8 for 24 h at 70 °C. | |
Effect of the polymerization time
The polymerization time span for the cross-linking reactions was varied from 6 hours to 48 hours using cross-linker
:
RAFT ratios equal to 6 and 8. During the polymerizations, the incorporation of arms into the star structures increased concomitantly with time as confirmed by GPC traces (Fig. 2). After 24 hours, some star coupling was observed, as indicated by the formation of a shoulder on the GPC chromatograms at low retention times. This coupling therefore imposed an upper time limit (24 h) to successful star production.
![Evolution of GPC traces of star polymersversuspolymerization time, (A) 0 h, (B) 6 h, (C) 12 h, (D) 18 h, (E) 24 h, and (F) 48 h. Experimental condition: [RAFT-polymer]0 : [monomer]0 : [cross-linker]0 ratio equal to 1 : 10 : 8 for 24 h at 70 °C.](/image/article/2011/PY/c1py00102g/c1py00102g-f2.gif) |
| Fig. 2 Evolution of GPC traces of star polymersversuspolymerization time, (A) 0 h, (B) 6 h, (C) 12 h, (D) 18 h, (E) 24 h, and (F) 48 h. Experimental condition: [RAFT-polymer]0 : [monomer]0 : [cross-linker]0 ratio equal to 1 : 10 : 8 for 24 h at 70 °C. | |
Effect of the cross-linker/RAFT ratio
The initial cross-linker
:
RAFT ratio was chosen based on our previous experience. However, in the current work, following our successful attainment of narrow distributions, we decided to probe the ratio from 2 to 32. For ratios from 2 to 6, a low incorporation of arms was observed by GPC. Arm incorporation was found to increase as the cross-linker
:
RAFT ratio increased. However, for a ratio equal to 16, the solution became turbid. After filtration, the soluble polymer fraction was analysed by DMAc GPC, indicating a shoulder at high molecular weights (low retention time) that could be attributed to star coupling (Fig. 3A). Above a ratio of 16, we observed the formation of macro-gels (Fig. 3B). Fig. 4 exhibits the evolution of the molecular weight and PDI of star polymersversus cross-linker
:
RAFT ratio. The molecular weight of star polymer increases versus cross-linker
:
RAFT ratio for the three different linear arms. In addition, the lowest PDI of star polymer was obtained for a ratio of cross-linker/RAFT equal to 8. Therefore using our reaction conditions the optimal cross-linker
:
RAFT ratio is eight for the synthesis of star polymers when using N,N′-bis(acryloyl)cystamine as the cross-linker.
![Evolution of the incorporation of linear arm (poly(OEG-A)) in the star polymersversus [RAFT-end group] : [cross-linker] ratio: (A) GPC traces of star polymers with different amounts of N,N′-bis(acryloyl)cystamine used as the cross-linker and linear poly(OEG-A), Mn, arm = 7000 g mol−1: red, blue, green and dark lines correspond to [RAFT-end group] : [cross-linker] ratio equal to 1 : 2,1 : 4, 1 : 8 and 1 : 16, respectively; inset picture shows the solution for different ratios (or equivalent); (B) Evolution of unreacted arm versus [RAFT-end group] : [cross-linker] ratio and molecular weight. Experimental condition: reaction was performed for 24 h at 70 °C.](/image/article/2011/PY/c1py00102g/c1py00102g-f3.gif) |
| Fig. 3 Evolution of the incorporation of linear arm (poly(OEG-A)) in the star polymersversus [RAFT-end group] : [cross-linker] ratio: (A) GPC traces of star polymers with different amounts of N,N′-bis(acryloyl)cystamine used as the cross-linker and linear poly(OEG-A), Mn, arm = 7000 g mol−1: red, blue, green and dark lines correspond to [RAFT-end group] : [cross-linker] ratio equal to 1 : 2,1 : 4, 1 : 8 and 1 : 16, respectively; inset picture shows the solution for different ratios (or equivalent); (B) Evolution of unreacted arm versus [RAFT-end group] : [cross-linker] ratio and molecular weight. Experimental condition: reaction was performed for 24 h at 70 °C. | |
![Evolution of the molecular weight (Mn) and PDI of the star polymersversus [N,N′-bis(acryloyl)cystamine] : [RAFT-end group] ratio for poly(OEG-A) arm with Mn, arm = 7000 g mol−1 (red circle), with Mn, arm = 11 500 g mol−1 (blue triangle) and with Mn, arm = 18 500 g mol−1 (green square). Experimental condition: reaction was performed for 24 h at 70 °C.](/image/article/2011/PY/c1py00102g/c1py00102g-f4.gif) |
| Fig. 4 Evolution of the molecular weight (Mn) and PDI of the star polymersversus [N,N′-bis(acryloyl)cystamine] : [RAFT-end group] ratio for poly(OEG-A) arm with Mn, arm = 7000 g mol−1 (red circle), with Mn, arm = 11 500 g mol−1 (blue triangle) and with Mn, arm = 18 500 g mol−1 (green square). Experimental condition: reaction was performed for 24 h at 70 °C. | |
Effect of the nature of cross-linker
The optimal conditions for N,N′-bis(acryloyl)cystamine were then applied to other cross-linkers predicted to have a low solubility in toluene, such as N,N′-methylene bisacrylamide (C2) and 1,2-dihydroxyethylene-bis-acrylamide (C3). Both cross-linkers yielded stars with a very low PDI (<1.20), Table 2. In the case of N,N′-methylene bisacrylamide, a [cross-linker]0
:
[RAFT]0 ratio equal to 4 was found to give the best incorporation of arms into star structures and the lowest PDI (without star coupling). It is interest to note that N,N′-methylene bisacrylamide presents a lower solubility (20 mg mL−1) compared to N,N′-bis(acryloyl)cystamine (35 mg mL−1), whilst 1,2-dihydroxyethylene-bis-acrylamide has the same solubility as N,N′-bis(acryloyl)cystamine. The effect of the solubility appeared a key factor for the formation of star polymers.
Table 2 Summary of the optimal poly(OEG-A) star synthesized viaRAFT polymerization using different cross-linkers. Influence of the structure of cross-linkers
Stars |
Polymer
|
Cross-linkera |
Ratiob |
Arm Mnc/g mol−1 |
Arm incorporationd (%) |
Star Mne/g mol−1 |
Arm PDIe |
Sizef/nm |
Nature of the cross-linker used in this study, i.e. C1–C4 correspond to N,N′-bis(acryloyl)cystamine, N,N′-methylene bisacrylamide, 1,6-hexanediol diacrylate and 1,2-dihydroxyethylene-bis-acrylamide (see Scheme 1 for the structures).
Optimal ratio of [cross-linker]/[RAFT agent] for the maximum incorporation for the arm polymers in the star structure.
Arm molecular weight determined by DMAc GPC.
Arm incorporation in the star structure assessed by GPC deconvulation of the arm and star peak.
Star molecular weight and PDI obtained by DMAc GPC.
Determined by dynamic light scattering (DLS).
|
S1 |
Poly(OEG-A) |
C1 |
8 |
7000 |
93 |
98 000 |
1.15 |
— |
S2 |
Poly(OEG-A) |
C1 |
8 |
11 500 |
91 |
100 000 |
1.19 |
18 |
S3 |
Poly(OEG-A) |
C1 |
8 |
18 500 |
88 |
118 000 |
1.18 |
28 |
S4 |
Poly(OEG-A) |
C2 |
4 |
7000 |
92 |
62 000 |
1.13 |
— |
S5 |
Poly(OEG-A) |
C2 |
4 |
11 500 |
91 |
104 000 |
1.15 |
22 |
S6 |
Poly(OEG-A) |
C2 |
4 |
18 500 |
13 |
118 000 |
1.19 |
30 |
S7 |
Poly(OEG-A) |
C3 |
8 |
7000 |
95 |
122 000 |
1.17 |
— |
S8 |
Poly(OEG-A) |
C3 |
8 |
11 500 |
92 |
136 000 |
1.15 |
24 |
S9 |
Poly(OEG-A) |
C3 |
8 |
18 500 |
90 |
210 000 |
1.19 |
35 |
S10 |
Poly(OEG-A) |
C4 |
8 |
7000 |
60 |
38 200 |
2.6 |
— |
S11
|
Poly(OEG-A) |
C4 |
8 |
11 500 |
40 |
62 000 |
1.46 |
— |
S12
|
Poly(OEG-A) |
C4 |
8 |
18 500 |
30 |
58 000 |
1.95 |
22 |
Purified poly(OEG-A) based star polymers were then analyzed by dynamic light scattering (DLS) in water, Table 2. Stars with short arms are only partially soluble as they have large hydrophobic cross-linked cores, but as the poly(OEG-A) molecular weights increase, the arms solubilize the star structures yielding nanostructures with diameters close to 20 nm and a dispersity close to 0.1 in good agreement with TEM results (Fig. 5). 1H NMR analyses were performed, confirming the absence of residual vinyl groups after crosslinking (data not shown).
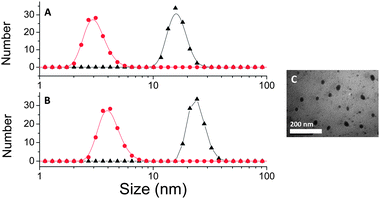 |
| Fig. 5
Dynamic light scattering (DLS) and TEM picture of star and arm polymers. (A) DLS of poly(OEG-A) arm with Mn, arm = 11 500 g mol−1 (red circle) and after formation of star polymers (black triangle) viaRAFT polymerization using N,N′-bis(acryloyl)cystamine as the cross-linker; (B) DLS of poly(OEG-A) arm with Mn, arm = 18 500 g mol−1 (red circle) and star polymer (black triangle) obtained viaRAFT polymerization using N,N′-bis(acryloyl)cystamine; (C) TEM picture of poly(OEG-A) star polymers with Mn, arm = 18 500 g mol−1. Experimental condition: reaction was performed for 24 h at 70 °C. | |
Nature of the polymer structure used for the arms
Initially, we optimized the synthesis of poly(OEG-A) based star polymers using partially soluble cross-linkers. Subsequently, alternative arm polymers poly(NIPAAm), poly(OEG-A-co-DEG-A) copolymer and poly(tert-Bu A) were investigated using N,N′-bis(acryloyl)cystamine as the cross-linker. For each arm polymer, different [cross-linker]0
:
[RAFT]0 ratios were studied (2
:
1, 4
:
1 and 8
:
1). Ratios 4
:
1 and 8
:
1 resulted in stars with more than 90% of the arm polymers incorporated and PDIs less than 1.2 (Table 3).
Table 3 Influence of the nature of arm polymers for the synthesis of star polymers using N,N′-bis(acryloyl)cystamine as the cross-linker
Stars |
Polymer
|
Ratioa |
Arm Mnb/g mol−1 |
Arm incorporationc (%) |
Star Mnd/g mol−1 |
Arm PDId |
Optimal [cross-linker] : [RAFT agent] ratio for the maximum incorporation for the arm polymers in the star structure.
Arm molecular weight determined by DMAc GPC.
Arm incorporation in the star structure assessed by GPC deconvulation of the arm and star peak.
Star molecular weight and PDI obtained by DMAc GPC.
|
S13
|
Poly(OEG-A) |
8 |
7000 |
93 |
98 000 |
1.15 |
S14
|
Poly(tert-Bu A) |
8 |
8000 |
96 |
180 000 |
1.08 |
S15
|
Poly(OEG-A-co-DEG-A) |
8 |
9500 |
86 |
135 000 |
1.19 |
S16
|
Poly(NIPAAm) |
4 |
8500 |
92 |
180 000 |
1.13 |
Disulfide bonds in the star cores (obtained by the polymerization of N,N′-bis(acryloyl)cystamine) were cleaved using a well established process,59,60i.e. by dimethylphenyl phosphine (DMPP) at ambient temperature overnight, and then, the products analysed by GPC. The star distribution disappeared (at low retention time) and a new distribution at high retention time appeared corresponding to the original population of linear arm polymers (Fig. 6).
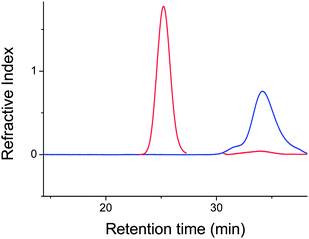 |
| Fig. 6
GPC traces of (red curve) before reduction of the disulfide of poly(tert-Bu A) star polymer and (blue) after degradation of the core using dimethylphenyl phosphine (DMPP). | |
Conclusion
In this article, we report for the first time, the synthesis of very well defined, narrow polydispersity, ‘arm-first’ star polymers made by RAFT polymerization using poorly soluble cross-linked cores for a range of polymers poly(OEG-A), poly(NIPAAm) and poly(tert-Bu A). The approach described in this paper involves a highly efficient incorporation of arms into the star polymer structures (close to quantitative yields for molecular weight arms less than 20
000 g mol−1). In contrast, when a fully solvent-compatible cross-linked core is used, a significantly reduced incorporation of arm polymers into the star structures and molecular weight broadening were observed. We are currently investigating these star polymers for drug delivery and imaging applications.
Acknowledgements
The authors acknowledge the Australian Research Council (ARC) for Discovery grant fundings (DP1092640 & DP110104251). In addition we would like to acknowledge significant research fellowship funding to TPD (Federation Fellowship) and CB (Australian Postdoctoral Fellowship).
References
- B. M. Erwin, M. Cloitre, M. Gauthier and D. Vlassopoulos, Soft Matter, 2010, 6, 2825–2833 RSC.
- S. Coppola, N. Grizzuti, G. Floudas and D. Vlassopoulos, J. Rheol., 2007, 51, 1007–1025 CrossRef CAS.
- T. K. Goh, K. D. Coventry, A. Blencowe and G. G. Qiao, Polymer, 2008, 49, 5095–5104 CrossRef CAS.
- M. Sugimoto, T. Koizumi, T. Taniguchi, K. Koyama, K. Saito, D. Nonokawa and T. Morita, J. Polym. Sci., Part B: Polym. Phys., 2009, 47, 2226–2237 Search PubMed.
- M. E. Fox, F. C. Szoka and J. M. J. Fréchet, Acc. Chem. Res., 2009, 42, 1141–1151 CrossRef CAS.
- C. Boyer, V. Bulmus, T. P. Davis, V. Ladmiral, J. Liu and S. Perrier, Chem. Rev., 2009, 109, 5402–5436 CrossRef CAS.
- J. T. Wiltshire and G. G. Qiao, Aust. J. Chem., 2007, 60, 699–705 CrossRef CAS.
- J.-H. Ryu, R. T. Chacko, S. Jiwpanich, S. Bickerton, R. P. Babu and S. Thayumanavan, J. Am. Chem. Soc., 2010, 132, 17227–17235 Search PubMed.
- V. Rodionov, H. Gao, S. Scroggins, D. A. Unruh, A.-J. Avestro and J. M. J. Fréchet, J. Am. Chem. Soc., 2010, 132, 2570–2572 CrossRef CAS.
- Q. Zhao, S.-J. Liu and W. Huang, Macromol. Chem. Phys., 2009, 210, 1580–1590 Search PubMed.
- A. L. Kanibolotsky, I. F. Perepichka and P. J. Skabara, Chem. Soc. Rev., 2010, 39, 2695–2728 RSC.
- N. Hadjichristidis, H. Iatrou, M. Pitsikalis and J. Mays, Prog. Polym. Sci., 2006, 31, 1068–1132 CrossRef CAS.
- K. Khanna, S. Varshney and A. Kakkar, Polym. Chem., 2010, 1, 1171–1185 RSC.
- S. Aoshima and S. Kanaoka, Chem. Rev., 2009, 109, 5245–5287 CrossRef CAS.
- K. Matyjaszewski and N. V. Tsarevsky, Nat. Chem., 2009, 1, 276–288 CrossRef CAS.
- W. Li and K. Matyjaszewski, Macromol. Rapid Commun., 2011, 32, 74–81 Search PubMed.
- H. Gao and K. Matyjaszewski, Prog. Polym. Sci., 2009, 34, 317–350 CrossRef CAS.
- G. Deng, M. Cao, J. Huang, L. He and Y. Chen, Polymer, 2005, 46, 5698–5701 Search PubMed.
- M. Ouchi, T. Terashima and M. Sawamoto, Acc. Chem. Res., 2008, 41, 1120–1132 CrossRef CAS.
- A. Blencowe, J. F. Tan, T. K. Goh and G. G. Qiao, Polymer, 2009, 50, 5–32 CrossRef CAS.
- S. Hou, E. L. Chaikof, D. Taton and Y. Gnanou, Macromolecules, 2003, 36, 3874–3881 CrossRef CAS.
- C. Boyer, M. H. Stenzel and T. P. Davis, J. Polym. Sci., Part A: Polym. Chem., 2011, 49, 551–595 CrossRef CAS.
- G. Moad, Aust. J. Chem., 2006, 59, 661–662 CrossRef CAS.
- H. Chaffey-Millar, M. H. Stenzel, T. P. Davis, M. L. Coote and C. Barner-Kowollik, Macromolecules, 2006, 39, 6406–6419 CrossRef CAS.
- M. H. Stenzel and T. P. Davis, J. Polym. Sci., Part A: Polym. Chem., 2002, 40, 4498–4512 CrossRef CAS.
- G. Moad, Y. K. Chong, A. Postma, E. Rizzardo and S. H. Thang, Polymer, 2005, 46, 8458–8468 CrossRef CAS.
- K. Ranganathan, R. Deng, R. K. Kainthan, C. Wu, D. E. Brooks and J. N. Kizhakkedathu, Macromolecules, 2008, 41, 4226–4234 CrossRef CAS.
-
D. Taton, J.-F. Baussard, L. Dupayage, Y. Gnanou, M. Destarac, C. Mignaud and C. Pitois, in Controlled/Living Radical Polymerization, American Chemical Society, 2006, vol. 944, pp. 578–594 Search PubMed.
- A. Duréault, D. Taton, M. Destarac, F. Leising and Y. Gnanou, Macromolecules, 2004, 37, 5513–5519 CrossRef CAS.
- G. Moad, E. Rizzardo and S. H. Thang, Acc. Chem. Res., 2008, 41, 1133–1142 CrossRef CAS.
- S. A. Bencherif, H. Gao, A. Srinivasan, D. J. Siegwart, J. O. Hollinger, N. R. Washburn and K. Matyjaszewski, Biomacromolecules, 2009, 10, 1795–1803 CrossRef CAS.
- H. Y. Cho, H. Gao, A. Srinivasan, J. Hong, S. A. Bencherif, D. J. Siegwart, H.-j. Paik, J. O. Hollinger and K. Matyjaszewski, Biomacromolecules, 2010, 11, 2199–2203 CrossRef CAS.
- C. Barner-Kowollik, T. P. Davis and M. H. Stenzel, Aust. J. Chem., 2006, 59, 719–727 CrossRef CAS.
-
D. Boschmann, M. Mänz, M. G. Fröhlich, G. Zifferer and P. Vana, in Controlled/Living Radical Polymerization: Progress in RAFT, DT, NMP & OMRP, American Chemical Society, 2009, vol. 1024, pp. 217–232 Search PubMed.
- M. G. Frohlich, P. Vana and G. Zifferer, J. Chem. Phys., 2007, 127, 164906–164907 Search PubMed.
- M. G. Fröhlich, M. M. Nardai, N. Förster, P. Vana and G. Zifferer, Polymer, 2010, 51, 5122–5134 Search PubMed.
- E. Setijadi, L. Tao, J. Liu, Z. Jia, C. Boyer and T. P. Davis, Biomacromolecules, 2009, 10, 2699–2707 CrossRef CAS.
- H. T. Lord, J. F. Quinn, S. D. Angus, M. R. Whittaker, M. H. Stenzel and T. P. Davis, J. Mater. Chem., 2003, 13, 2819–2824 RSC.
- J. Liu, L. Tao, J. Xu, Z. Jia, C. Boyer and T. P. Davis, Polymer, 2009, 50, 4455–4463 CrossRef CAS.
- H. Hussain, B. H. Tan, C. S. Gudipati, Y. Xaio, Y. Liu, T. P. Davis and C. B. He, J. Polym. Sci., Part A: Polym. Chem., 2008, 46, 7287–7298 Search PubMed.
- J. F. Quinn, R. P. Chaplin and T. P. Davis, J. Polym. Sci., Part A: Polym. Chem., 2002, 40, 2956–2966 CrossRef CAS.
- M. H. Stenzel-Rosenbaum, T. P. Davis, V. Chen and A. G. Fane, Macromolecules, 2001, 34, 5433–5438 CrossRef CAS.
- M. H. Stenzel, T. P. Davis and C. Barner-Kowollik, Chem. Commun., 2004, 1546–1547 RSC.
- J. Bernard, X. Hao, T. P. Davis, C. Barner-Kowollik and M. H. Stenzel, Biomacromolecules, 2005, 7, 232–238 Search PubMed.
- L. Viau, M. Even, O. Maury, D. M. Haddleton and H. Le Bozec, C. R. Chim., 2005, 8, 1298–1307 Search PubMed.
- A. P. Narrainen, S. Pascual and D. M. Haddleton, J. Polym. Sci., Part A: Polym. Chem., 2002, 40, 439–450 CrossRef CAS.
- K. Ohno, B. Wong and D. M. Haddleton, J. Polym. Sci., Part A: Polym. Chem., 2001, 39, 2206–2214 CrossRef CAS.
- D. M. Haddleton, R. Edmonds, A. M. Heming, E. J. Kelly and D. Kukulj, New J. Chem., 1999, 23, 477–479 RSC.
- B. S. Sumerlin and A. P. Vogt, Macromolecules, 2009, 43, 1–13.
- P. L. Golas and K. Matyjaszewski, Chem. Soc. Rev., 2010, 39, 1338–1354 RSC.
- J. A. Johnson, M. G. Finn, J. T. Koberstein and N. J. Turro, Macromol. Rapid Commun., 2008, 29, 1421 Search PubMed.
- J. W. Chan, B. Yu, C. E. Hoyle and A. B. Lowe, Polymer, 2009, 50, 3158–3168 CrossRef CAS.
- J. W. Chan, B. Yu, C. E. Hoyle and A. B. Lowe, Chem. Commun., 2008, 4959–4961 RSC.
- J. F. Tan, A. Blencowe, T. K. Goh, I. T. M. Dela Cruz and G. G. Qiao, Macromolecules, 2009, 42, 4622–4631 CrossRef CAS.
- M. Spiniello, A. Blencowe and G. G. Qiao, J. Polym. Sci., Part A: Polym. Chem., 2008, 46, 2422–2432 CrossRef CAS.
- T. K. Goh, A. P. Sulistio, A. Blencowe, J. W. Johnson and G. G. Qiao, Macromolecules, 2007, 40, 7819–7826 Search PubMed.
- J. A. Syrett, D. M. Haddleton, M. R. Whittaker, T. P. Davis and C. Boyer, Chem. Commun., 2011, 47, 1449–1451 RSC.
- C. Boyer, V. Bulmus and T. P. Davis, Macromol. Rapid Commun., 2009, 30, 493–497 CrossRef.
- J. Rosselgong, S. P. Armes, W. Barton and D. Price, Macromolecules, 2009, 42, 5919–5924 CrossRef CAS.
- J. Rosselgong, S. P. Armes, W. R. S. Barton and D. Price, Macromolecules, 2010, 43, 2145–2156 CrossRef CAS.
Footnotes |
† Electronic supplementary information (ESI) available. See DOI: 10.1039/c1py00102g |
‡ Dedicated to the honour of Professor Malcolm B. Huglin on the occasion of his 80th Birthday. |
|
This journal is © The Royal Society of Chemistry 2011 |