DOI:
10.1039/C1PY00053E
(Paper)
Polym. Chem., 2011,
2, 1661-1670
Design and synthesis of thermo-responsive hyperbranched poly(amine-ester)s as acid-sensitive drug carriers
Received
30th January 2011
, Accepted 7th April 2011
First published on 20th April 2011
Abstract
Novel thermo-responsive hyperbranched poly(amine-ester)s were designed and synthesized successfully in one-pot through proton-transfer polymerization of triethanolamine, trimethylolpropane, and glycidyl methacrylate with potassium hydride as a catalyst. The structure of the obtained polymers was confirmed by nuclear magnetic resonance, gel permeation chromatography, Fourier transformed infrared spectroscopy, and differential scanning calorimetery techniques. Thermal induced phase transition behaviors of hyperbranched poly(amine-ester)s were investigated by dynamic light scattering measurements, and the results indicated that these polymers had a tunable lower critical solution temperature (LCST) ranging from 37 to 57 °C. In vitro evaluation suggested that hyperbranched poly(amine-ester)s exhibited low cell cytotoxicity and efficient cell internalization against COS-7 cells. Moreover, doxorubicin (DOX) as a model drug was encapsulated into hyperbranched poly(amine-ester)s in aqueous solution above the LCST. In vitro release studies revealed that the loaded DOX displayed acid-triggered (pH ≈ 5.0) drug release behaviors. The DOX-loaded delivery system was investigated for proliferation inhibition of a Hela human cervical carcinoma cell line, and the DOX dose required for 50% cellular growth inhibition (IC50) was found to be 1.1 μg mL−1. All of these results suggest that thermo-responsive hyperbranched poly(amine-ester)s can be used to construct promising drug delivery systems for cancer therapy.
Introduction
Thermo-responsive polymers that exhibit unique property changes in response to environmental temperature are promising materials for various biomedical applications, such as smart drug/gene delivery systems, injectable tissue engineering scaffolds, etc.1–6 These polymers are readily soluble in water below their lower critical solution temperature (LCST) and their aqueous solutions undergo a phase transition to insoluble state under increased temperatures. In particular, polymers with LCST around 37 °C have received much attention due to their phase transition close to body temperature. In the past few years, a large number of linear thermo-responsive polymers have been prepared consequently and some of them have been further used to construct drug delivery systems.7–10 Thermo-responsive dendritic polymers, which combine the advantages of thermo-responsiveness and the unique properties of dendritic structure such as high solubility, low viscosity, and abundance of terminal groups, have attracted increasing interest.11–14 Two different strategies have been employed to prepare thermo-responsive dendritic polymers. One is the incorporation of thermo-responsive groups or polymeric chains onto the surface of a dendritic polymer. The other is the grafting of hydrophobic and hydrophilic functionalities into a highly branched polymer, and the thermo-responsiveness is given to the dendritic polymer by the appropriate balance of hydrophilic and hydrophobic moieties. To date, many kinds of thermo-responsive dendritic polymers have been designed and synthesized.15–28 However, drug delivery vehicles based on these polymers have only just been studied, while the unique architecture and properties of thermo-responsive dendritic polymers may bring advantages in drug loading and release.
For drug delivery applications, it is preferred that the drug delivery systems remain stable without or with little drug leakage during circulation in the blood but release the drug after reaching the target sites. According to previous reports, there are numerous pH gradients existing in both normal and pathological states.29 For example, the pH of tumor extracellular environment is about 6.5, which is more acidic than that of blood (pH ≈ 7.4). Meanwhile, the pH of endosomes and lysosomes are even lower (pH = 5.0–5.5). Therefore, acid-sensitive drug delivery systems are very attractive and required. In our previous report, a series of backbone thermo-responsive hyperbranched polymers have been produced easily by proton-transfer polymerization.27,28 The thermo-responsive behavior can be attributed to the combination of the hydrophilic groups (such as −OH and –O–) and hydrophobic groups (such as –CH3, –CH2CH3, and –C4H8–) in the highly branched backbone. Herein, novel thermo-responsive hyperbranched poly(amine-ester)s were designed and successfully synthesized viaproton-transfer polymerization of commercial available trimethylolpropane, triethanolamine, and glycidyl methacrylate in one-pot. Interestingly, the LCST can be easily controlled near 37 °C through adjusting the composition of hyperbranched poly(amine-ester)s. Moreover, hydrophobic molecules such as anticancer drug doxorubicin (DOX) can be encapsulated by hyperbranched poly(amine-ester)s in aqueous solution above the LCST. The DOX-loaded delivery system is relatively stable at neutral pH but susceptible to mild acidic environments which would trigger the release of encapsulated drugs. The structure of the obtained polymers was analyzed by nuclear magnetic resonance (NMR), gel permeation chromatography (GPC), Fourier transformed infrared spectrum (FTIR), and differential scanning calorimeter (DSC) techniques. Thermal induced phase transition behaviors of hyperbranched poly(amine-ester)s were investigated by dynamic light scattering (DLS) measurements. Moreover, the cell cytotoxicity and internalization of hyperbranched poly(amine-ester)s against living cells were evaluated by methyl tetrazolium (MTT) assay, flow cytometry and confocal laser scanning microscopy (CLSM) methods. In addition, the size and morphology of the DOX-loaded hyperbranched poly(amine-ester)s in aqueous solution above the LCST was characterized by DLS and transmission electron microscopy (TEM). The acid-triggered (pH ≈ 5.0) drug release behaviors and the cell proliferation inhibition efficiency of the DOX-loaded delivery system was also studied.
Experimental section
Materials
Potassium hydride (KH, 30 wt% dispersion in mineral oil, Acros), trimethylolpropane (TMP, Acros), N-hydroxysuccinamide (NHS, Acros), N,N-dicyclohexylcarbodiimide (DCC, Aldrich), 4-dimethylamino-pyridine (DMAP, Aldrich), 3-(4,5-dimethyl-thiazol-2-yl)-2,5-diphenyl tetrazolium bromide (MTT, Aldrich), and polyethylenimine (PEI, water free, Mw = 25 kDa, Mn = 10 kDa, Aldrich) were used as received. Triethanolamine (TEOA) was distilled under reduced pressure. Glycidyl methacrylate (GMA, Acros), dimethyl sulfoxide (DMSO), and N,N-dimethylformamide (DMF) were dried over calcium hydride (CaH2) and distilled under reduced pressure. Tetrahydrofuran (THF) was dried by refluxing over fresh sodium-benzophenone complex (a deep purple color indicating an oxygen- and moisture-free solvent) and distilled before polymerization. Doxorubicin hydrochloride (DOX·HCl) was purchased from Beijing Huafeng United Technology Corporation and used as received. Clear polystyrene tissue culture treated 6-well and 96-well plates were obtained from Corning Costar. Other reagents were purchased from Shanghai Chemical Reagent Co. and used as received.
Synthesis of hyperbranched poly(amine-ester)s
A typical polymerization procedure is described as follows: a suspension of KH in mineral oil (30% in weight) was introduced in a dry pre-weighed 100 mL Schlenk flask under argon (Ar). The mineral oil was removed by three extractions with THF, and the remaining THF was removed by vacuum. When KH was completely dried, the flask was weighed again to determine the amount of KH (0.767 g, 19.1 mmol). Then TEOA (4.14 g, 27.8 mmol) and TMP (3.73 g, 27.8 mmol) were introduced into the flask. After that, 40 mL DMSO was added into the flask. The solution was stirred for 30 min to form the alcoholate potassium. Then GMA (8.01 g, 56.3 mmol) was added by syringe and the polymerization was conducted at 80 °C for 48 h. Upon completion of the polymerization, the mixture was precipitated into 1000 mL acetone/diethyl ether (v/v = 1/4). The product was redissolved in methanol and neutralized by filtration over cation-exchange resin. The polymer was precipitated twice from methanol solution into cold diethyl ether and subsequently dried in vacuo at 50 °C for 24 h. Similarly, polymers with different compositions were synthesized similarly by changing TEOA/TMP molar ratios (see Table 1). The resultant purified product was highly viscous.
Table 1 Reaction conditions and results of hyperbranched poly(amine-ester)s
Entrya |
T(TEOA + TMP)/Gb |
TEOA/TMPc |
M
w
d (×103) |
M
n
d (×103) |
M
w/Mnd |
DB
e |
T
g
f (°C) |
LCSTg (°C) |
Zeta potential (mV) |
The polymer obtained from the corresponding condition listed.
The molar ratio of TEOA + TMP to GMA.
The molar ratio of TEOA to TMP.
The molecular weights and polydispersities were determined by GPC.
The degree of branching (DB) was calculated from quantitative 13C NMR analysis.
T
g was obtained from DSC.
LCST was measured by DLS.
|
P1
|
1 : 1 |
1 : 1 |
5.8 |
4.3 |
1.34 |
0.58 |
−69 |
37 |
6.7 |
P2 |
1 : 1 |
1.2 : 1 |
7.2 |
4.7 |
1.52 |
0.68 |
−27 |
41 |
7.4 |
P3 |
1 : 1 |
1.5 : 1 |
5.7 |
4.1 |
1.40 |
0.71 |
−14 |
57 |
8.2 |
Synthesis of RB-labeled hyperbranched poly(amine-ester)s
A typical reaction is described as follows: NHS (0.123 g, 1.06 mmol) and rhodamine B (RB, 0.51 g, 1.06 mmol) were dissolved in 20 mL of DMF, and then DCC (0.219 g, 1.06 mmol) and DMAP (130 mg, 1.06 mmol) were added to the solution. The mixture was conducted at room temperature for 0.5 h with stirring. Hyperbranched poly(amine-ester) (P2, 0.92 g, 0.196 mmol) in 15 mL DMF was added to the reaction solution. The reaction was conducted at room temperature for another 48 h. Then the insoluble substance was filtered and DMF was removed by rotary evaporation. The residues were dissolved in distilled water. Exhaustive dialysis against exchanged distilled water was carried out for 7 days, followed by lyophilization to give the RB-labeled hyperbranched poly(amine-ester).
Characterization of polymers
1H and 13C NMR spectra of the polymers were recorded using Brucker Avance III 400 MHz spectrometer with dimethyl sulfoxide-d6 (DMSO-d6) as a solvent. Quantitative 13C NMR spectra were measured by the method of inverse gated 1H decoupling. In DEPT experiments, the 1H tip angle θ was set to 135° to determine carbon multiplicities with CH, CH3 up and CH2 down. 1H,1H-COSY and 13C,1H-HSQC spectra were recorded using the standard pulse sequence provided by Brucker. FTIR measurements were performed on a Bruker Equinox-55 FTIR spectrometer with a disk of KBr. The molecular weights and polydispersity index were determined by GPC. GPC was performed on a Perkin-Elmer series 200 system (10 μm PL gel 300 × 7.5 mm mixed-B and mixed-C column, polystyrene calibration) equipped with a refractive index (RI) detector. DMF contained 0.01 mol L−1lithium bromide was used as the mobile phase at a flow rate of 1 mL min−1 at 70 °C. The glass transition temperature (Tg) of hyperbranched polymers was measured with a Perkin-Elmer Pyris 1 DSC under nitrogen (N2) atmosphere. All samples were heated from −80 to 100 °C at a heating rate of 10 °C min−1. The midpoint of the slope change of the heat capacity plot of the second heating scan was taken as Tg. For zeta potential, the hyperbranched poly(amine-ester)s were dissolved in phosphate buffered saline (PBS) (pH = 7.4) at the concentration of 1 mg mL−1. The zeta potential was measured with Zetasizer 2000 (Malvern, UK). Values of zeta potential were averaged over three repeated measurements.
TEM measurements
TEM studies were performed with a JEOL 2010 instrument operated at 200 kV. The samples were prepared by directly dropping the aqueous solutions of DOX-loaded hyperbranched poly(amine-ester)s at 50 °C onto carbon-coated copper grids and dried at 50 °C without staining before measurement.
DLS measurements
DLS measurements were performed in aqueous solution using a Malvern Zetasizer Nano S apparatus equipped with a 4.0 mW laser operating at λ = 633 nm. All samples were measured at a scattering angle of 173°.
Cell cultures
COS-7 cells (a cell line derived from kidney cells of the African green monkey) and Hela cells (a human cervical carcinoma cell line) were cultivated in DMEM (Dulbecco's modified Eagle's medium) containing 10% FBS (fetal bovine serum), and antibiotics (50 units/mL penicillin and 50 units/mL streptomycin) at 37 °C in a humidified atmosphere containing 5% CO2.
Cytotoxicity assay of hyperbranched poly(amine-ester)s
The cytotoxicity of the hyperbranched poly(amine-ester)s was performed by MTT assays. The MTT assay is based on the ability of a mitochondrial dehydrogenation enzyme in viable cells to cleave the tetrazolium rings of the pale yellow MTT and form formazan crystals with a dark blue color. Therefore, the number of surviving cells is directly proportional to the level of formed formazan.30,31 For cytotoxicity, the P1–P3 samples were dissolved in DMEM at diluted polymer concentrations from 3/256 to 3 mg mL−1, respectively. COS-7 cells were seeded into 96-well plates at a seeding/initial density of 6000 cells/well in 200 μL complete DMEM. After 24 h incubation, the culture medium was removed and replaced with 200 μL medium containing serial concentrations of polymers. The cells were grown for another 48 h. Then 20 μL of 5 mg mL−1 MTT assays stock solution in PBS was added to each well. After incubating the cells for 4 h, the medium containing unreacted dye was removed carefully. The obtained blue formazan crystals were dissolved in 200 μL per well DMSO and the absorbance was measured in a BioTek ELX 800 at a wavelength of 490 nm.
Thermal induced phase transition
The PBS (pH = 7.4) solution of hyperbranched poly(amine-ester)s with a concentration of 1 wt% was prepared at 4 °C and filtered with 0.22 μm millipore to make dust-free it prior to measurement. During the DLS measurements, the samples were heated 1–2 °C per step and equilibrated at each temperature for 20 min before measurements. The LCST values were determined by the plot of count rate vs. temperature. LCST was defined as the intersection of the horizontal tangent through the points at high temperatures with the line drawn through the points of the drastically decreasing regions at low temperatures.
Cell internalization
Flow cytometry was firstly used to provide statistics on the uptake of RB-labeled polymers into COS-7 cells. COS-7 cells were seeded in a 6-well tissue culture plate with an initial seeding density of 5.0 × 105cells per well. After 24 h incubation, the RB-labeled P2 dissolved in DMEM with a polymer concentration of 1 mg mL−1 was added and the cells were further incubated at 37 °C for predetermined time intervals. Samples were prepared by removing the cell growth media, rinsing with PBS buffer, and treating with trypsin. Data for 1.0 × 104 events were collected and analysis was performed by means of a BD FACSCalibur flow cytometer and CELLQuest software.
Cell internalization was further characterized by CLSM. COS-7 cells were seeded on coverslips in a 6-well tissue culture plate with an initial seeding density of 2.0 × 105cells per well. After 24 h culture, the RB-labeled P2 dissolved in DMEM culture medium with a polymer concentration of 1 mg mL−1 was added and the cells were further incubated at 37 °C for predetermined time intervals. After washing with PBS, the cells were fixed with 4% formaldehyde for 30 min at room temperature, and the slides were rinsed with PBS for three times. Finally, the cells were stained with 2-(4-amidinophenyl)-6-indolecarbamidine dihydrochloride (DAPI) for 10 min and the slides were rinsed with PBS for three times. The slides were mounted and observed by a LSM 510META.
Acid-triggered drug release
The pH-dependent release behaviors of DOX were studied at two different pHs. For pH = 7.4, 2.5 mL P2 solution (8 mg mL−1) was placed in a refrigerator (∼ 4 °C) for 2 h, then 2.5 mL DOX solution (0.8 mg mL−1) were added to the polymer solution. Thereafter the solution was quickly heated to 37 °C and incubated overnight to equilibrate the DOX. After dialyzing against pH = 7.4 phosphate buffer solution (PBS, MWCO = 1000 g mol−1), the absorbance of the solution was recorded at 37 °C as for the starting point. At regular intervals, 2 mL of media was withdrawn and replenished with 2 mL of fresh PBS. The solutions were measured at the desired time points at a wavelength of 485 nm. In the case of pH = 5.0, the mixture solution of the polymer and DOX was dialyzed against pH = 5.0 acetate buffer solution (MWCO = 1000 g mol−1), and the absorbance of the solution was recorded at 37 °C as for the starting point and measured at the desired time points at a wavelength of 485 nm with Lambda 20.
Activities
The cytotoxicity of DOX-loaded hyperbranched poly(amine-ester)s and free DOX against Hela cells was evaluated in vitro by MTT assay. Hela cells were seeded into 96-well plates at 1 × 104cells per well in 200 μL medium. After 24 h incubation, the culture medium was removed and replaced with 200 μL medium containing serial dilutions of DOX-loaded hyperbranched poly(amine-ester)s or free DOX. The cells were grown for another 24 h. Then 20 μL 5 mg mL−1 MTT assays stock solution in PBS was added to each well. After incubating the cells for 4 h, the medium containing unreacted MTT was removed carefully. The obtained blue formazan crystals were dissolved in 200 μL DMSO/well and the absorbance was measured in a BioTek ELX 800 at a wavelength of 490 nm.
Results and discussion
Synthesis and characterization of hyperbranched poly(amine-ester)s
As shown in Scheme 1, hyperbranched poly(amine-ester)s are synthesized through proton-transfer polymerization of triethanolamine (TEOA), trimethylolpropane (TMP), and glycidyl methacrylate (GMA) in DMSO at 80 °C with potassium hydride (KH) as a catalyst. During the polymerization, the TEOA (1) and TMP (2) first react with KH to originate initiator 3 and 4. Due to the bifunctionality of GMA, there are two possibilities for the reaction of GMA with 3 and 4, respectively. 3 and 4 can separately initiate the epoxy group of GMA leading to 5 and 7. Meanwhile, 3 and 4 can react with the vinyl group of GMA producing 9 and 11, respectively. Proton-transfer from the secondary alkoxide or carbonanion to the more stable primary alkoxide is expected, thus producing 6, 8, 10 and 12. Further polymerization of 5, 6, 7, 8, 9, 10, 11, and 12 gives rise to hyperbranched poly(amine-ester)s (13). The experimental conditions and corresponding characterization data are summarized in Table 1.
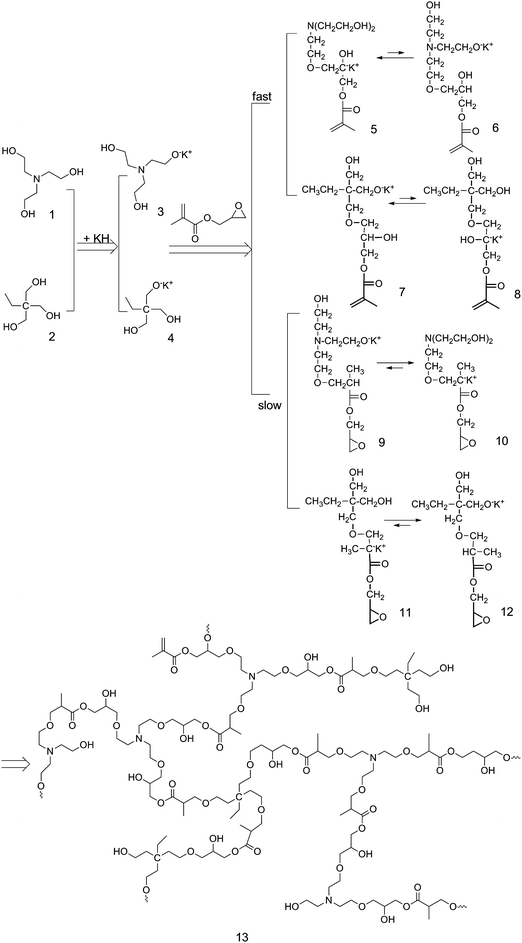 |
| Scheme 1 Synthetic route to hyperbranched poly(amine-ester)s. | |
Careful examination of the structure of hyperbranched poly(amine-ester)s reveals that four terminal subunits, six linear subunits, and six dendritic subunits may be present (Fig. 1). A DEPT-135 spectrum experiment was performed to distinguish methylene, methane or methine carbons. As shown in Fig. 2, the peaks 5, 18, 22, 31 and 34 are assigned to the methyl groups from GMA units according to the DEPT spectrum. The peaks 6, 7 and 10 correspond to the double bond signals and epoxide resonances from GMA, respectively. The peaks 15, 32 and 35 are attributed to quaternary carbon atoms, which disappear in the corresponding DEPT spectra. The branched topology of hyperbranched poly(amine-ether)s could be further verified by 2D-NMR analysis.32–34 The 1H,1H-COSY and 13C,1H-HSQC spectra in Fig. 3 can be used for assignment of the peaks. Fig. 3A reveals that the signals 3-H/4-H, 5-H/7-H, 7-H/7-H, 12-H/13-H, 17-H/19-H, 18-H/19-H, 22-H/23-H, 28-H/29-H and 41-H/42-H are in different spin systems. Based on the information of the 1H,1H-COSY and 13C,1H-HSQC spectra, the detailed assignment is shown in Fig. 3. Therefore the degree of branching (DB) for the hyperbranched polymer can be calculated according to the following equation:35
where
D,
T and
L represent the fractions of the dendritic, terminal, and linear units, respectively. The DBs of the samples synthesized from TMP, TEOA and GMA are listed in
Table 1, which confirms the formation of highly branched products.
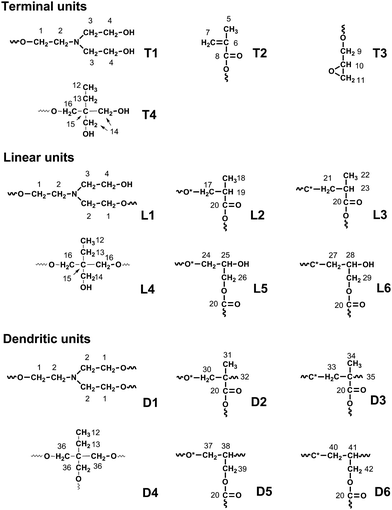 |
| Fig. 1 The various structural units of hyperbranched poly(amine-ester)s. | |
FTIR results provide the additional chemical structure information of hyperbranched poly(amine-ester)s. As displayed in Fig. 4, the absorption peaks at 1115 and 1024 cm−1 are assigned to C–N–C and C–O–C stretching vibrations, respectively. The band at 1460 cm−1 corresponds to the asymmetric deformation vibration of the –CH2– groups. A strong ester carbonyl band at 1728 cm−1 confirms the presence of ester bonds. The band at 2881 cm−1 is related to the symmetric –CH2– stretching vibration, and the bands at 2920 and 2956 cm−1 are attributed to the asymmetric –CH2– and –CH3 stretching vibrations respectively. The broad O-H stretching vibration around 3381 cm−1 suggests the appearance of many terminal hydroxyl groups on the periphery of hyperbranched poly(amine-ester)s.
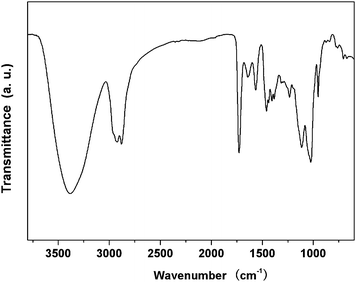 |
| Fig. 4 Representative FTIR spectrum of hyperbranched poly(amine-ester)s. | |
The molecular weights and polydispersity index (PDI) of hyperbranched poly(amine-ester)s were analyzed by GPC measurements. As summarized in Table 1, the weight average molecular weights of the polymers are around 6.0 × 103 g mol−1, with PDI about 1.4. Moreover, zeta potential analyses were further used to measure the charge properties of hyperbranched poly(amine-ester)s. The results show that the zeta potentials of these polymers change from 6.7 to 8.2 mV (Table 1). Zeta potential analysis demonstrates that hyperbranched poly(amine-ester)s are positively charged, attributing to the presence of numerous tertiary amines. Additionally, DSC measurements show that the Tg values of hyperbranched poly(amine-ester)s range from −69 to −14 °C. The introduction of flexible units such as ether bond into the macromolecular structure may plasticize the polymers, which reduces Tg of hyperbranched polymers in comparison with that of linear poly(glycidyl methacrylate) (78 °C).
Thermal induced phase transition
Hyperbranched poly(amine-ester)s are highly soluble in water at low temperatures. Interestingly, the solution becomes opaque at a specific temperature as the increase of temperature. Contrarily, the polymer solution becomes transparent again as the temperature decreases. In order to determine the LCSTs of hyperbranched poly(amine-ester)s, DLS was used to measure the transmittance of the polymer aqueous solution as a function of temperature. Fig. 5 shows the temperature dependence of light scattering intensity at the polymer concentration of 1 mg mL−1 in PBS (pH = 7.4). All samples were equilibrated at each temperature for 20 min before measurements. It can be seen that the light scattering intensity of all the polymer solutions remain invariably below their LCSTs. After reaching their critical temperatures, a clear increase in the scattering intensity is detected. It is explained that hyperbranched poly(amine-ester)s possesses hydrophilic groups (such as –OH and –O–) and hydrophobic moieties (such as –CH2–) in the highly branched backbone. As the temperature is above LCST, the hydrophobic interaction drives the polymer chains to aggregate and separate from the water. Here LCST is defined as the intersection of the horizontal tangent through the points at high temperatures with the line drawn through the points of the drastically decreasing regions at low temperatures. The results show that the LCSTs of hyperbranched poly(amine-ester)s are about 37, 41, and 57 °C for P1, P2, and P3, respectively (Table 1). It is reported that the ratio of the hydrophobic and hydrophilic units in a polymer chain will affect the LCSTs of the polymer.36–38 In general, hydrophobic units result in the low LCSTs,39 while hydrophilic units increase the LCSTs.40 Because TEOA is more hydrophilic than TMP, the LCST of hyperbranched poly(amine-ester)s becomes higher with increasing of the molar ratio of TEOA/TMP.
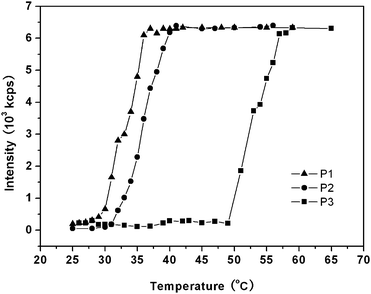 |
| Fig. 5 Temperature dependence of light scattering intensity of hyperbranched poly(amine-ester) solutions in the heating process. | |
Cell cytotoxicity
Low cytotoxicity is a very important requirement of polymer materials using for drug delivery applications. To evaluate the cytotoxicity of hyperbranched poly(amine-ester)s, in vitro evaluation against COS-7 cells was determined by MTT assay. PEI was used as a positive control. As shown in Fig. 6, cells cannot tolerate the treatment of PEI even at a concentration of 3/256 mg mL−1 after incubation for 48 h. On the contrast, the cell viabilities after 48 h incubation with hyperbranched poly(amine-ester)s up to 3 mg mL−1 remain above 80% compared with the untreated cells. The results suggest the low cytotoxicity of hyperbranched poly(amine-ester)s.
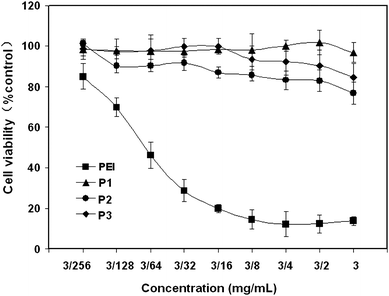 |
| Fig. 6
Cell viability of hyperbranched poly(amine-ester)s against COS-7 cells at different polymer concentration compared to the control (PEI). | |
Cell internalization studies
Flow cytometry analysis was performed to test the cellular uptake of RB-labeled hyperbranched poly(amine-ester)s by COS-7 cells. COS-7 cells were cultured for predetermined time intervals with polymer concentration of 1 mg mL−1 before analysis. Cellular uptake was determined as the number of fluorescent cells increased. Fig. 7 displays the histograms of cell-associated RB fluorescence against COS-7 cells. The relative geometrical mean fluorescence intensities of polymer pretreated cells are about 60 fold of nonpretreated cells within 30 min and the fluorescence intensity gradually increases over a period of 3 h. The fast enhancement of fluorescence signals indicates the efficient cellular uptake of hyperbranched poly(amine-ester)s by COS-7 cells. It is suggested that the positive charge of hyperbranched poly(amine-ester)s enhances the electrostatic interaction between the polymers and negatively charged cell membranes. Consequently, the increased binding is followed by rapid internalization within the cells.41,42 The cellular uptake of hyperbranched poly(amine-ester)s by COS-7 cells was further evaluated by CLSM. COS-7 cells were incubated with RB-labeled hyperbranched poly(amine-ester)s of 1 mg mL−1 at 37 °C for 0.5, 1, and 3 h, respectively. Cells incubated with free RB were used as a control. RB fluorescence of the cells was observed directly under CLSM. As exhibited in Fig. 8, the RB fluorescence in all cells pretreated with RB-labeled hyperbranched poly(amine-ester)s is accumulated preferentially in the perinuclear region instead of the nucleus. Furthermore, the fluorescence remains in cytoplasm when the incubation time is prolonged (Fig. 8A–C). However, the fluorescence is mostly localized in the cell nucleus when cells are pretreated with free RB for only 30 min (Fig. 8D). Unlike the simple passive diffusion of small molecules between the extracellular and intracellular milieu, the cellular uptake of hyperbranched poly(amine-ester)s is unidirectional, resulting in the efficient cell internalization of hyperbranched poly(amine-ester)s.43,44
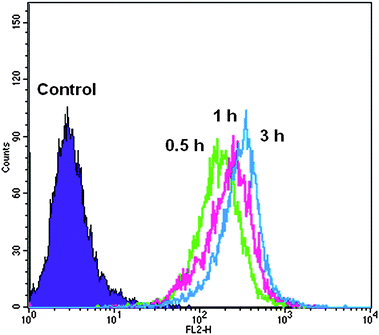 |
| Fig. 7
Flow cytometry histogram profiles of COS-7 cells incubated with RB-labeled hyperbranched poly(amine-ester)s at different time intervals. | |
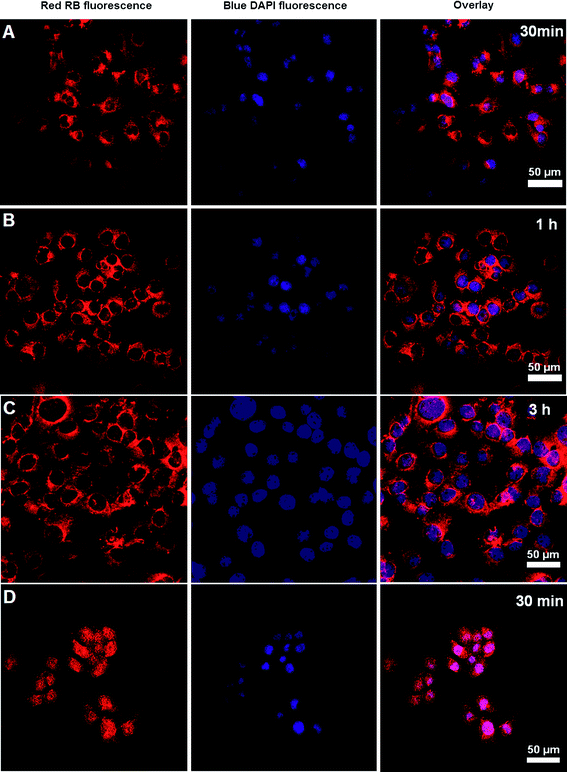 |
| Fig. 8
CLSM images of COS-7 cells incubated with RB-labeled hyperbranched poly(amine-ester)s for different time intervals. Free RB for 30 min was used as control. The cell nuclei were stained with DAPI. | |
pH-dependent release of DOX and the inhibition of tumor cell proliferation
Hyperbranched polymers are good candidates for drug carriers because of their intrinsic properties, such as branching architecture, 3D globular architecture, a large number of terminal functional groups, lower viscosity and better solubility. The highly branched architecture induces the formation of nanocavities, the environment of which determines their solubilizing or encapsulating properties, while the external groups primarily characterize their solubility and chemical behavior. DOX is one of the most potent anticancer drugs used widely in the treatment of different types of solid malignant tumors and known to interact with DNA by intercalation and inhibition of macromolecular biosynthesis.45,46 Here DOX was selected as a model drug for encapsulating in hyperbranched poly(amine-ester)s and the acid-sensitive release profiles were investigated. The size and morphology of the DOX-loaded hyperbranched poly(amine-ester)s in aqueous solution above the LCST was characterized by DLS and TEM techniques. As given in Fig. 9A, the DOX-loaded drug delivery system has an average size of 104 nm and a size distribution of 0.273. The representative TEM image in Fig. 9B shows that the DOX-loaded hyperbranched poly(amine-ester)s in aqueous solution above the LCST can form approximate spherical nanoparticles. Furthermore, the release of DOX was monitored by UV-Vis at a wavelength of 485 nm. DOX-loaded hyperbranched poly(amine-ester)s were formed at the 37 °C and equilibrated overnight. Then the equilibrated solution was dialyzed against different buffer solution with varied pH. As plotted in Fig. 10, the cumulative release of DOX is 35.7% at a release time of 29 h under physiological conditions (pH = 7.4 and 37 °C), while 63.1% of DOX is released under slightly acidic solution (pH = 5.0 and 37 °C). The results indicate that the drug release from hyperbranched poly(amine-ester)s can be responsible for tumorigenesis of local tissue, which is slightly more acidic than normal tissue.
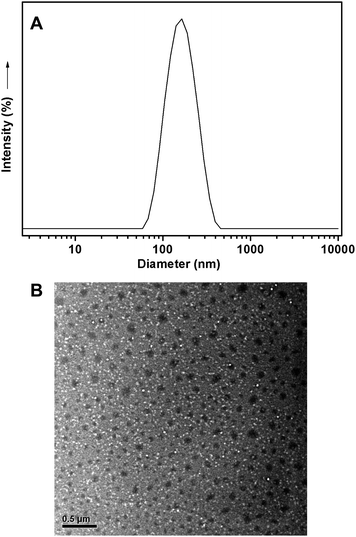 |
| Fig. 9 Representative DLS curve and TEM photo of DOX-loaded hyperbranched poly(amine-ester)s in aqueous solution above the LCST with a DOX content of 1.02%. | |
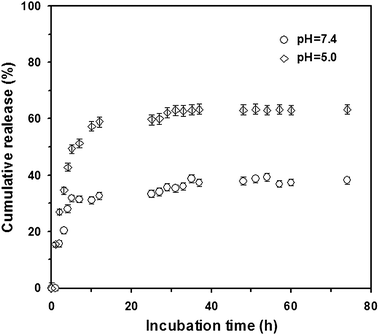 |
| Fig. 10 Cumulative release of drug from DOX-loaded hyperbranched poly(amine-ester)s at different pHs. Mean ± SD (n = 3). | |
The tumor cell proliferation inhibition of loaded DOX and free DOX were determined by MTT assays against Hela cells. The cells were incubated with DOX-loaded hyperbranched poly(amine-ester)s for 24 h at different drug concentration from 0.1 to 10 μg mL−1. Free DOX with the same concentration was used as a control. As revealed in Fig. 11, the cell viability of loaded DOX show slightly less activity than that of the free DOX. The loaded DOX dose required for 50% cellular growth inhibition (IC50) after 24 h incubation is 1.1 μg mL−1. It is well reported by other groups that DOX-loaded nanoparticles are less potent in cell proliferation inhibition than free DOX at the same dose in vitro.47,48 This may be due to the time-consuming DOX-release and delayed nuclear uptake.
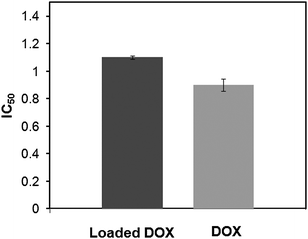 |
| Fig. 11 The IC50 of loaded DOX in hyperbranched poly(amine-ester)s against Hela cells. Free DOX was used as a control. | |
Conclusions
A series of novel thermo-responsive hyperbranched poly(amine-ester)s were prepared successfully viaproton-transfer polymerization of triethanolamine, trimethylolpropane, and glycidyl methacrylate in DMSO at 80 °C using potassium hydride as a catalyst. The structure of hyperbranched poly(amine-ester)s was well confirmed by NMR, GPC, FTIR, and DSC techniques. DLS measurements indicated that hyperbranched poly(amine-ester)s had a tunable LCST ranging from 37 to 57 °C. MTT assay indicated that these polymers exhibited low cell cytotoxicity against COS-7 cells even with the concentration up to 3 mg mL−1. Flow cytometry and CLSM analyses verified the efficient cell internalization of positive charged hyperbranched poly(amine-ester)s. Moreover, hyperbranched poly(amine-ester)s in aqueous solution above the LCST were further used to construct drug delivery systems. In vitro release studies revealed that DOX-loaded delivery system displayed an acid-triggered (pH ≈ 5.0) release profile. The DOX-loaded polymers were further investigated for proliferation inhibition of Hela cells and the DOX dose required for IC50 was found to be 1.1 μg mL−1. Combining the advantages of thermo-responsiveness and highly branched structure, hyperbranched poly(amine-ester)s can be used to construct smart drug delivery systems.
Acknowledgements
This work was financially supported by the National Natural Science Foundation of China (20974062, 30700175) and National Basic Research Program 2009CB930400, Shanghai Leading Academic Discipline Project (No. B202), and China National Funds for Distinguished Young Scientists (21025417). J. Y. Liu gratefully acknowledges a Scholarship Award for Excellent Doctoral Student granted by the Ministry of Education.
References
- S. Nayak, H. Lee, J. Chmielewski and L. A. Lyon, J. Am. Chem. Soc., 2004, 126, 10258 CrossRef CAS.
- R. Yoshida, T. Takahashi, T. Yamaguchi and H. Ichijo, Adv. Mater., 1997, 9, 175 CrossRef CAS.
- J. Guan, Y. Hong, Z. Ma and W. R. Wagner, Biomacromolecules, 2008, 9, 1283 Search PubMed.
- S. H. Yuk, S. H. Cho and S. H. Lee, Macromolecules, 1997, 30, 6856 CrossRef CAS.
- H. Cheng, J. Zhu, Y. Sun, S. Cheng, X. Zhang and R. Zhuo, Bioconjugate Chem., 2008, 19, 1368 Search PubMed.
- A. Chilkotia, M. R. Drehera, D. E. Meyera and D. Raucherb, Adv. Drug Delivery Rev., 2002, 54, 613 CrossRef CAS.
- X. Huang, F. Du, J. Cheng, Y. Dong, D. Liang, S. Ji, S. Lin and Z. Li, Macromolecules, 2009, 42, 783 CrossRef CAS.
- X. J. Loh, Z. Zhang, Y. Wu, T. S. Lee and J. Li, Macromolecules, 2009, 42, 194 CrossRef CAS.
- Y. Wang, L. Tang, Y. Li and J. Wang, Biomacromolecules, 2009, 10, 66 CrossRef CAS.
- D. Wu, F. Qiu, T. Wang, X. Jiang, X. Zhang and R. Zhuo, ACS Appl. Mater. Interfaces, 2009, 1, 319 Search PubMed.
- H. Hong, Y. Mai, Y. Zhou, D. Yan and Y. Chen, J. Polym. Sci., Part A: Polym. Chem., 2008, 46, 668 CrossRef CAS.
- J. Zhang, S. Huang and R. Zhuo, Macromol. Biosci., 2004, 4, 575 CrossRef CAS.
- Y. You, C. Hong, C. Pan and P. Wang, Adv. Mater., 2004, 16, 1953 CrossRef CAS.
- A. Saha and S. Ramakrishnan, Macromolecules, 2008, 41, 5658 Search PubMed.
- C. Kojima, K. Yoshimura, A. Harada, Y. Sakanishi and K. Kono, Bioconjugate Chem., 2009, 20, 1054 Search PubMed.
- Y. Haba, A. Harada, T. Takagishi and K. Kono, J. Am. Chem. Soc., 2004, 126, 12760 CrossRef CAS.
- Y. Haba, C. Kojima, A. Harada and K. Kono, Macromolecules, 2006, 39, 7451 CrossRef.
- Y. Tono, C. Kojima, Y. Haba, T. Takahashi, A. Harada, S. Yagi and K. Kono, Langmuir, 2006, 22, 4920 CrossRef.
- M. Kimura, M. Kato, T. Muto, K. Hanabusa and H. Shirai, Macromolecules, 2000, 33, 1117 CrossRef CAS.
- C. Kojima, Y. Haba, T. Fukui, K. Kono and T. Takagishi, Macromolecules, 2003, 36, 2183 CrossRef CAS.
- C. Kojima, K. Yoshimura, A. Harada, Y. Sakanishi and K. Kono, J. Polym. Sci., Part A: Polym. Chem., 2010, 48, 4047 Search PubMed.
- S. V. Aathimanikandan, E. N. Savariar and S. Thayumanavan, J. Am. Chem. Soc., 2005, 127, 14922 CrossRef CAS.
- M. C. Parrott, E. B. Marchington, J. F. Valliant and A. Adronov, J. Am. Chem. Soc., 2005, 127, 12081 CrossRef.
- M. C. Parrott, J. F. Valliant and A. Adronov, Langmuir, 2006, 22, 5251 Search PubMed.
- I. Gitsov and J. M. J. Fréchet, J. Am. Chem. Soc., 1996, 118, 3785 CrossRef CAS.
- E. R. Gillies, T. B. Jonsson and J. M. J. Fréchet, J. Am. Chem. Soc., 2004, 126, 11936 CrossRef CAS.
- Z. F. Jia, G. L. Li, Q. Zhu, D. Y. Yan, X. Y. Zhu, H. Chen, J. L. Wu, C. L. Tu and J. Sun, Chem.–Eur. J., 2009, 15, 7593 Search PubMed.
- Z. F. Jia, H. Chen, X. Y. Zhu and D. Y. Yan, J. Am. Chem. Soc., 2006, 128, 8144 CrossRef.
- P. Vaupel, F. Kallinowski and P. Okunieff, Cancer Res., 1989, 49, 6449 CAS.
- T. Mosmann, J. Immunol. Methods, 1983, 65, 55 CrossRef CAS.
- M. Prabaharan, J. J. Grailer, S. Pilla, D. A. Steeber and S. Gong, Macromol. Biosci., 2009, 9, 515 Search PubMed.
- Y. Pang, Q. Zhu, J. Y. Liu, J. L. Wu, R. B. Wang, S. Y. Chen, X. Y. Zhu, D. Y. Yan, W. Huang and B. S. Zhu, Biomacromolecules, 2010, 11, 575 Search PubMed.
- Y. Pang, J. Y. Liu, J. L. Wu, G. L. Li, R. B. Wang, Y. Su, P. He, X. Y. Zhu, D. Y. Yan and B. S. Zhu, Bioconjugate Chem., 2010, 21, 2093 Search PubMed.
- X. Y. Zhu, L. Chen, Y. Chen and D. Y. Yan, Sci. China, Ser. B: Chem., 2008, 51, 1057 Search PubMed.
- C. J. Hawker, R. Lee and J. M. J. Fréchet, J. Am. Chem. Soc., 1991, 113, 4583 CrossRef CAS.
- M. Gao, X. Jia, G. Kuang, Y. Li, D. Liang and Y. Wei, Macromolecules, 2009, 42, 4273 CrossRef CAS.
- L. E. Bromberg and E. S. Ron, Adv. Drug Delivery Rev., 1998, 31, 197 CrossRef CAS.
- B. Verdonck, E. J. Goethals and F. E. D. Prez, Macromol. Chem. Phys., 2003, 204, 2090 CrossRef CAS.
- Y. G. Takei, T. Aoki, K. Sanui, N. Ogata, T. Okano and Y. Sakurai, Bioconjugate Chem., 1993, 4, 341 CrossRef CAS.
- M. Shibayama, T. Tanaka and C. C. Han, J. Chem. Phys., 1992, 97, 6842 CrossRef CAS.
- Y. S. Nam, H. S. Kang, J. K. Park, T. G. Park, S. H. Han and I. S. Chang, Biomaterials, 2003, 24, 2053 Search PubMed.
- W. T. Godbey, K. K. Wu and A. G. Mikos, Proc. Natl. Acad. Sci. U. S. A., 1999, 96, 5177 CrossRef CAS.
- O. L. P. D. Jesús, H. R. Ihre, L. Gagne, J. M. J. Fréchet and F. C. Jr. Szoka, Bioconjugate Chem., 2002, 13, 453 CrossRef CAS.
- T. Ouchi and Y. Ohya, Prog. Polym. Sci., 1995, 20, 211 CrossRef CAS.
- A. Akinc, D. G. Anderson, D. M. Lynn and R. Langer, Bioconjugate Chem., 2003, 14, 979 CrossRef CAS.
- D. A. Gewirtz, Biochem. Pharmacol., 1999, 57, 727 CrossRef CAS.
- X. Shuai, H. Ai, N. Nasongkla, S. Kim and J. Gao, J. Controlled Release, 2004, 98, 415 CrossRef CAS.
- K. S. Soppimath, L. H. Liu, W. Y. Seow, S. Q. Liu, R. Powell, P. Chan and Y. Y. Yang, Adv. Funct. Mater., 2007, 17, 355 CrossRef CAS.
Footnote |
† These authors are joint first authors. |
|
This journal is © The Royal Society of Chemistry 2011 |