DOI:
10.1039/C1PY00041A
(Review Article)
Polym. Chem., 2011,
2, 2133-2145
Diels–Alder “click” reactions: recent applications in polymer and material science
Received
25th January 2011
, Accepted 29th April 2011
First published on 27th May 2011
Abstract
The “click” chemistry concept is based on utilizing rapid reactions which are efficient, versatile, and selective. Indeed, Diels–Alder (DA) reactions fulfill most of the requirements for the “click” chemistry concept. In this review, we discuss the recent reports concerned with the use of DA “click” reactions in the synthesis of various macromolecular architectures, bioconjugates and hybrid materials.
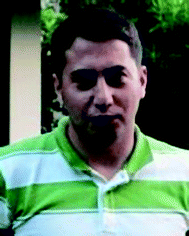 Mehmet Atilla Tasdelen | Mehmet Atilla Tasdelen received his MSc (2002) and PhD degree (2008) from the Polymer Science and Technology Program of the Istanbul Technical University under the supervision of Professor Yagci. He joined Yalova University, Department of Polymer Engineering in July, 2010 as an Assistant Professor. His research topics focus on the design of functional macromolecular architectures, photoinitiated cationic and radical polymerization, controlled polymerization techniques, “click” chemistry and nanocomposites. He is the co-author of 35 research papers and 11 book chapters. |
1 Introduction
Diels–Alder (DA) reaction is one of the most common reactions used in organic chemistry and invented by Otto Diels and Kurt Alder who received the Nobel Prize in 1950 for their discovery.1 DA reaction involves a straightforward [4 + 2] cycloaddition reaction between an electron-rich diene (furan and its derivatives, 1,3 cyclopentadiene and its derivatives, etc.) and an electron-poor dienophile (maleic acid and its derivatives, vinyl ketone, etc.) to form a stable cyclohexene adduct as illustrated in Fig. 1.2,3 This reaction requires a very low energy to form a cyclohexene ring and it allows the formation and functionalization of numerous molecules. DA cycloaddition reaction forms not only carbon–carbon bonds but also heteroatom–heteroatom bonds (hetero-Diels–Alder, HDA) and it is widely used synthetically to prepare six-membered rings.4 Some attractive features of DA reactions (retro-Diels–Alder, rDA) are thermal reversibility and the decomposition reaction of the cyclic system that can be controlled by temperature.5
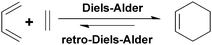 |
| Fig. 1 General mechanism of Diels–Alder/retro Diels–Alder reactions of dienophile and diene. | |
Over the last decade, increasing attention has been devoted to the use of rapid reactions that meet the three main criteria of an ideal synthesis: efficiency, versatility, and selectivity. The extensively studied reactions that have been adapted to fulfill these criteria are known as “click” reactions. They are classified into four categories: (i) cycloaddition reactions (most commonly Huisgen 1,3-dipolar cycloaddition,6–9 but also Diels–Alder reaction), (ii) nucleophilic ring-opening reactions of strained heterocyclic electrophiles (epoxides, aziridines and aziridinium ions),10 (iii) non-aldol carbonyl chemistry (ureas, oximes and hydrazones)11,12 and (iv) additions to carbon–carbon multiple bonds (especially thiol–ene chemistry but also Michael additions).13–16 Among these, the most widely used reaction is copper catalyzed Huisgen 1,3-dipolar cycloaddition of terminal azides and alkynes to form triazole rings.17 Apparently, some Diels–Alder reactions easily accomplish the several requirements of “click” chemistry.18 Additionally, an advantage of these reactions is that they can proceed in the absence of a metal catalyst. The chemical structures of well-known dienes, dienophiles and adducts and reaction conditions are shown in Table 1. In order to assess whether a reaction may be classified as a “click” reaction, a modified definition in the context of polymer chemistry has been proposed by a group of polymer chemists.19 In the adapted definition, a few more requirements have been added to the original definition of Sharpless' because of major differences in the macromolecular synthesis: (i) to use equimolar amounts of the building blocks, (ii) a simple large-scale purification process and (iii) a reasonable timescale and require no tedious fine-tuning of reaction conditions. Therefore, it is more appropriate to call some Diels–Alder reactions as “click-type” or simply “efficient conjugation” methods.
Table 1 Selected well-known Diels–Alder reactions in polymer and material science
A large number of specialized reviews have been published during the last decade on both copper-catalyzed azide–alkyne cycloaddition (CuAAC) and thiol–ene “click” reactions, however, only limited literature address the use of Diels–Alder (DA) cycloaddition reactions as “click” or “click-type” reactions in polymer and material science.9,18,31–35 From this point, this review summarizes the potential use of DA “click” reactions in these fields and presents the recent examples to demonstrate the current state-of-the-art.
2 Macromolecular architectures via DA “click” chemistry
Synthesis of macromolecules with advanced architectures (e.g. homopolymers, telechelic, dendronized and star polymers, and block and graft copolymers) has been the essential aim of polymer chemists.9,35,36 DA “click” reactions in combination with living/controlled polymerization methods (including reversible addition-fragmentation chain transfer polymerization (RAFT),18,26,37,38 nitroxide mediated radical polymerization (NMRP),25,39 atom transfer radical polymerization (ATRP),24,40,41 ring-opening polymerization (ROP)37,40,42,43) are a facile route to access various architectures.
A simple and efficient DA cycloaddition reaction can be employed in the preparation of linear thermoplastic44 and thermosetting polymers45 such as polyimides,46–55 polyphenylenes,56–59 ladder polymers, etc.60,61 The polymerization by the DA cycloaddition reaction can be achieved by starting with monomers containing both diene and dienophile functional groups (A–B type monomer) in a single molecule or via the intermolecular reaction of bisdienes with bisdienophiles (A–A and B–B co-monomers) (Fig. 2).62 Although, the DA polymerization proceeds via the reaction of unsaturated compounds and the resulting polymers do not have a structure typical for condensation products, the polymerization process is similar to polycondensation.63–65 Diels–Alder linkages on the polymer backbone are desirable for adjusting the chain length,44 control of processing viscosity66 and for improved recyclability.67
Telechelic polymers are defined as macromolecules containing reactive end groups that have the capacity to enter into further polymerization or other reactions.68 The efficiencies of DA cycloaddition reactions have allowed that they can be used for the preparation of telechelic polymers. For example, Haddleton and co-workers reported an alternative synthetic route to prepare maleimido-functionalized telechelic polymers via retro-Diels–Alder reaction. Well-defined telechelic polymethacrylates can be prepared by this strategy, which avoided the potential cross-linking side-reactions.69
Similarly, bismaleimide end-functionalized poly(ethylene oxide) (PEO) or poly(polypropylene oxide) (PPO) was reacted with an anthracene molecule having 2,2,6,6-tetramethylpiperidin-1-oxy (TEMPO) and tert-bromide groups via DA cycloaddition reaction. The obtained telechelic polymer with tertiary bromide and TEMPO functional groups was subsequently used for ATRP of tert-butyl acrylate (tBA) and for NMRP of styrene (St) to produce the heteroarm H-shaped terpolymers.70 DA “click” reaction was also successfully combined with RAFT polymerization for the preparation of telechelic polymers. Sumerlin et al. presented a general strategy for end group functionalization of RAFT-generated polymers by DA “click” reaction.71 Thiol terminated poly(N-isopropylacrylamide) (PNIPAM), prepared by RAFT/aminolysis, was reacted with excess of 1,8-bismaleimide derivatives to yield maleimido terminated PNIPAM. The maleimide dienophile allowed efficient coupling with 9-anthracene methanol by DA “click” reaction to yield a hydroxy monotelechelic polymer (Fig. 3).
In another study, thioxanthone end-functionalized PEO was successfully synthesized by using the DA “click” chemistry strategy. The final polymer could be used as photoinitiator for water-borne photopolymerization of several hydrophilic vinyl monomers, such as acrylic acid, acrylamide, 2-hydroxyethyl acrylate, and 1-vinyl-2-pyrrolidone.72
As a model case, the preparation of block copolymers would be a suitable platform to show that DA cycloaddition reactions allow a facile access to more complex macromolecular architectures. For example, DA “click” reaction, [4 + 2] system, based on the coupling of furan protected maleimide- and anthracene-end functionalized polymers has been successfully used to generate numerous block copolymers.24,73 Well-defined functional precursors, PEO, polystyrene (PSt) and poly(methyl methacrylate) (PMMA), were prepared by anionic ROP, NRMP and ATRP techniques, respectively.24,25,70 The overall strategy is represented in Fig. 4 by the preparation of PEO-b-PSt and PEO-b-PMMA.
Recently, Kimura and co-workers prepared an anthracene-terminated poly(L-lactide) and a maleimide-terminated poly(D-lactide) by tin-catalyzed ROP of the corresponding lactide with 2-hydroxy-methylanthracene or N-(2-hydroxyethyl)maleimide as the initiator. Simple heating of these polymers could generate the poly(L-lactide)-b-poly(D-lactide) copolymer through anthracene–maleimide type DA “click” reaction.74
The HDA reactions between heterodienophiles such as imines, aldehydes or thiocarbonyl compounds with suitable dienes are also powerful method in the synthesis of heterocycles.4,75,76 Barner-Kowollik and co-workers have recently introduced an efficient HDA type “click” reaction between diene- and dithioester-functional polymers.26,77,78 In the first example of the HDA “click” reaction, they reported the synthesis of polystyrene-b-poly(ε-caprolactone) (PSt-b-PCL) by heating a solution of dithioester-terminated polystyrene and a diene-terminated PCL in the presence of catalyst at 50 °C between 2 and 24 h depending upon the nature of the dithioester end-group (Fig. 5).37 Precursor polymers could be obtained by RAFT and ROP methods using benzyl(diethoxyphosphoryl)dithioformate or benzylpyridin-2-yl dithioformate and trans,trans-2,4-hexadienol, respectively.37 In this approach, an electron-withdrawing dithioester played a key role regarding the controlling agent in a RAFT polymerization and subsequently as the reactive heterodienophile in an HDA “click” reaction. The reaction was catalyzed by the addition of either Lewis acids such as zinc chloride in the case of the diethoxyphosphoryl dithioester end-group or trifluoroacetic acid in the case of the pyridinyl dithioester end-group. These catalysts coordinate to sulfur atoms of the dithioester end-group in order to increase the electrophilicity on the thiocarbonyl bond.
The efficiency of HDA “click” reaction is dramatically accelerated by changing the open-chain diene to a cyclic one such as cyclopentadiene, even at ambient temperature in just a few minutes without the addition of catalyst. This ultrafast HDA “click” reaction was extremely efficient and resulted in almost complete conversion of macromolecular coupling just by shaking the reaction flask at room temperature.26,79,80 Furthermore, this “click” reaction can also provide an access to high molecular weight block copolymers ranging from 34
000 to over 100
000 and with low polydispersities (PDI < 1.2).81
Durmaz et al.82 elegantly achieved a one-pot “click” construction of an ABC triblock copolymers such as PEO-b-PSt-b-PMMA and PCL-b-PSt-b-PMMA by utilizing tandem CuAAC and DA “click” reactions (Fig. 6). Well-defined polymeric precursors were obtained by either ROP of EO and CL or ATRP of St and MMA. The middle block was heterobifunctional PSt containing anthracene at the initiator end and azide at the termination end. Copper catalytic system was used to catalyze both CuAAC and DA “click” reactions at high temperature, and the triblock copolymer was obtained with high coupling efficiencies in a one-pot strategy.
Yagci, Tunca and Hizal et al. reported on the preparation of well-defined graft copolymers on the basis of the DA “click” chemistry between polymer backbone bearing anthryl pendant groups and maleimide end-functionalized polymers.25 The “grafting onto” processes were carried out at the reflux temperature of toluene with a quantitative yield and without need for an additional purification step. Two series of well-defined PSt-g-PEO and PSt-g-PMMA copolymers were successfully prepared through the DA “click” reaction.
Later on, Barner-Kowollik and Stenzel demonstrated the synthesis of a graft copolymer via a combination of RAFT and HDA “click” methods.83,84 The diene functionalized poly(trans-hexa-2,4-dienylacrylate-r-styrene) backbone and various dienophile functionalized polymers were prepared via RAFT polymerization of corresponding monomers, independently. Then, the HDA “click” reaction of these two polymers led to the formation of graft copolymer in 12 to 24 hours at 50 °C.
The orthogonality of DA and CuAAC “click” reactions was also demonstrated by preparing modular hetero-graft copolymers from maleimide-terminated PtBA, alkyne-terminated PEO, and anthracene- and azide-functionalized PSt backbone in a one-pot strategy (Fig. 7).39 The graft copolymers were obtained with high grafting efficiency in the range from about 90% to nearly 100% showing low polydispersity indices (1.15–1.17). The use of consecutive DA and CuAAC “click” chemistries in a one-pot reaction was a versatile and straightforward strategy for the preparation of well-defined heterograft copolymer which cannot be obtained by using only one of the “click” reactions.
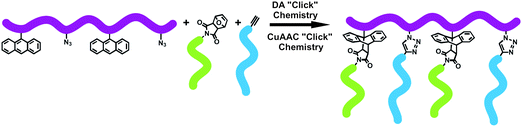 |
| Fig. 7 Synthesis of hetero-graft copolymer via one-pot CuAAC and DA “click” reactions. | |
In another study, graft and graft-block copolymers were synthesized by either combination of ring-opening metathesis polymerization/Diels–Alder (ROMP/DA) or CuAAC/ROMP/DA strategies. First, anthracene end-functionalized polystyrene macromonomer was synthesized through CuAAC “click” reaction of an oxanorbornenyl alkyne with azido terminated polystyrene. Then the obtained macromonomer or anthracene-functionalized oxanorbornene was polymerized via ROMP using the first-generation Grubbs' catalyst. Finally, poly(oxanorbornenyl anthracene) or poly(oxanorbornene)-g-polystyrene-anthracene copolymer was coupled with maleimide end-functionalized polymers, such as PEO, PMMA and poly(n-butyl acrylate) via DA “click” reactions to yield corresponding graft and graft-block copolymers, respectively.85 Recently, this strategy was applied to synthesise brush copolymers with AB block-brush architectures containing polyoxanorbornene backbone and PCL, PMMA or PtBA side chains.86
2.5 Complex macromolecular architectures
Diels–Alder cycloaddition reaction is a very useful and versatile toolbox to construct complex macromolecular architectures because of its quantitative yield, mild reaction conditions and tolerance of numerous functional groups. The Tunca and Hizal group demonstrated that a number of these examples were accessible by DA “click” reactions, sometimes in combination with CuAAC, thus representing the wide scope and modularity. For example, several A3 type star polymers containing PEO, PMMA and PtBA arms were efficiently synthesized by DA “click” reactions using furan protected maleimide end-functional polymers and a trianthracene core.87 ABC type miktoarm star polymers were prepared by combination of DA “click”, ATRP and NMRP processes.88 In this case, DA cycloaddition was performed with a maleimide-terminated PEO and an anthracene functionalized dual initiator which is capable to initiate both NMRP and ATRP of St and t-BA, respectively (Table 2).
Type of reactions |
Polymerization methods |
Topology |
Polymera |
PSt, polystyrene; PMMA, poly(methyl methacrylate); PEO, poly(ethylene oxide); PPO, poly(propylene oxide); PtBA, poly(t-butyl acrylate); PCL, poly(ε-caprolactone); PiBorA, poly(isobornyl acrylate); PDVB, poly(divinyl benzene).
|
DA |
ATRP/AROP |
Linear AB block copolymer24,73 |
PMMA-b-PSt, PEO-b-PSt, PtBA-b-PSt, PMMA-b-PEO, PCL-b-PtBA |
CuAAC and DA |
ATRP/ATRP/AROP |
Linear ABC block copolymer82 |
PEO-PSt-PMMA PCL-PSt-PMMA |
CuAAC and DA |
AROP/ATRP/NMRP |
Hetero-graft copolymer39 |
PSt-g-(PMMA-PEO) |
CuAAC and DA |
ATRP/AROP |
Multiblock-graft copolymer89 |
PSt-g-PMMA, PSt-g-PEO, PSt-g-PtBA |
DA |
AROP/ATRP/NMRP |
H-shaped A2B2C block copolymer70 |
PSt-b-PtBA-b-PEO-b-PtBA-b-PSt PSt-b-PtBA-b-PPO-b-PtBA-b-PSt |
DA |
AROP/ATRP/NMRP/ROP |
H-shaped ABCDE quintopolymer90 |
PEO-b-PMMA-b-PtBA-b-PCL-b-PSt |
DA |
AROP/ATRP |
Star polymer A387 |
PEO-PMMA-PtBA |
CuAAC and DA |
ROP |
Star polymer A4 and A891 |
PCL4, PCL8 |
HDA |
RAFT |
Star polymers A2, A3, A438 |
PSt2, PSt3, PSt4 |
CuAAC and DA |
ATRP/AROP |
Mikto-arm star A3B392 |
(PSt-b-PMMA)3 (PSt-b-PEO)3 |
DA |
ATRP |
Multi-arm star-block polymer93 |
PMMA-PSt PtBA-PSt |
CuAAC and DA |
ATRP/AROP |
Multi-arm star triblock terpolymers94 |
(PtBA)k-(PMMA)n-(PSt)m-PDVB |
CuAAC and HDA |
ROP/RAFT |
Multi-arm star block copolymers95 |
(PSt-b-PCL)3 |
CuAAC and DA |
ATRP |
Multi-arm star polymers with peripheral dendritic PMMA96 |
(PMMA)2n-(PSt)m-PDVB and (PMMA)4n-(PSt)m-PDVB |
CuAAC and DA |
ATRP |
Multi-miktoarm star block copolymers97 |
(PtBA)n-(PSt)m-PDVB-(PSt)m-(PMMA)k |
HDA |
ATRP/RAFT |
Multi-arm star block copolymers98 |
(PiBorA-b-PSt)12 |
The orthogonality of DA and CuAAC “click” reactions was demonstrated by preparing modular three-arm star block copolymers ((PSt-b-PMMA)3 and (PSt-b-PEO)3) from maleimide-terminated PMMA or PEO and α-anthracene-ω-azide-terminated polystyrene.92 Linear PEO, PSt, and PMMA precursors were prepared via ATRP of related monomers, except a commercially available PEO. Similarly, a one-pot “double-click” approach was employed to prepare H-shaped ABCDE quinto-polymers.90
Barner-Kowollik and co-workers recently reported synthesis of star-shaped polymers by combination of RAFT/HDA “click”, RAFT and ATRP/HDA “click” or ROP and RAFT/CuAAC and HDA “click” processes. In the first example, RAFT polymerization of styrene was followed by a HDA “click” reaction to yield star polymers with up to four arms.38 In general, the coupling efficiencies were found to decrease with increasing number of arms. When a diethoxyphosphoryldithioformate terminated polymer was used the yields of 2-arm star, 3-arm star, and 4-arm star polymers were 81%, 77% and 65%, respectively, and when a pyridin-2-yl dithioformate terminated polymer was used, the yields of 2-arm star, 3-arm star and 4-arm star polymers were 91%, 86% and 82% respectively.
The synthesis of three-arm (PSt-b-PCL)3 star-block copolymers by a sequential combination of the CuAAC and HDA “click” reactions was successfully performed through an arm-first and core-first strategy.95 The strategy involves the preparation of an α-diene-ω-alkyne functionalized PCL by AROP, a PSt equipped with an electron-deficient dithioester end group by RAFT, and a triazide coupling agent as the corresponding building blocks. In addition, a twelve-armed star-block copolymer composed of PiBorA internal and PSt external blocks was synthesized by combination of ATRP, RAFT and HDA “click” reactions.98 In this system, bromine functionality of an ATRP polymerized multi-functional (PiBorA-Br)12 was converted into diene end groups and subsequently coupled with dithioester functionality of a RAFT-polymerized PSt via HDA “click” chemistry.
In a recent study, Tunca et al. synthesized cyclic homo and block copolymers by combination of double CuAAC and DA “click” reaction strategy.99 First, well-defined linear precursors were obtained via combination of ATRP, anionic ROP and CuAAC methods. Afterwards, these linear homo or block copolymers were efficiently coupled via DA “click” reaction to give their corresponding cyclic homo and block copolymers. Combination of CuAAC and DA “click” reactions strategy enabled us to prepare cyclic block copolymers, which cannot be achieved by using a single “click” reaction (Fig. 8).
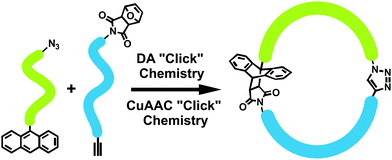 |
| Fig. 8 Synthesis of cyclic block copolymers via tandem CuAAC and DA “click” reactions. | |
Diels–Alder cycloaddition reaction between anthracene and maleimide derivatives has proven to produce excellent results in the formation of dendrimers and dendronized polymers in a modular approach. First, McElhanon and co-workers reported the use of thermally reversible furan–maleimide DA cycloaddition reactions for a convergent approach towards benzyl aryl ether dendrimers up to the third generation (Fig. 9).22 The system required a disubstituted furan that could serve as an AB2 monomer and an N-substituted maleimide with a reactive hydroxyl focal point, which was finally coupled with the 1,3,5-benzenetricarbonyl trichloride central linker in the presence of triethylamine in tetrahydrofuran. This work was the first example of a new class of thermally reversible dendrimer taking advantage of the DA/rDA approach. Later on, the same group20 reported a similar system with a bismaleimide core and fourth generation benzyl aryl ether based dendrons that contained furan moieties at their focal point to access first through fourth generation Diels–Alder dendrimers. The DA cycloaddition reactions have also been used in peripheral modification of carbosilane dendrimers and represent a facile example of covalent structural modification.100–103
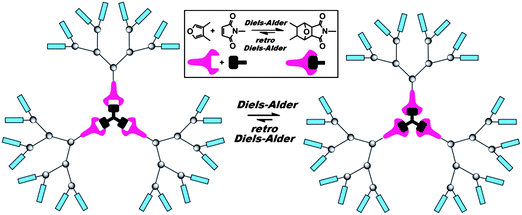 |
| Fig. 9 Reversible furan–maleimide Diels–Alder reaction for the formation and controlled cleavage of dendrimers around a plurifunctional core. | |
Sanyal's group104 recently reported the synthesis of unsymmetrical (AB type) dendrimers by DA “click” reaction of furan functionalized polyaryl ether dendrons and maleimide functionalized polyester dendrons. Precursor dendrons were prepared by coupling of acid-functionalized Frechet dendrons with furfuryl alcohol and divergently starting with a furan-protected N-hydroxypropylmaleimide. The DA cycloaddition reaction of dendrons in benzene at 85 °C for 24 h led to the three different generations of unsymmetrical dendrimers (G1, G2 and G3) with 98%, 76%, and 79%, respectively.
Similarly, dendronized polymers (linear polymer chains with bulky side dendrons) have been prepared by DA “click” reaction using an anthracene-bearing PSt backbone and third-generation polyester dendrons containing reactive maleimide at the focal point.105 Kakkar et al. have designed appropriate building blocks to construct thermoresponsive dendrimers by combination of CuAAC and furan–maleimide type DA “click” reactions, in a sequential manner.106 These thermally labile dendrimers, which can undergo facile retro-DA disassembly, led to a suite of new nanoscopic materials for a variety of applications. McElhanon and co-workers also reported the synthesis of a linear dendronized step polymer utilizing sequential “double-click” reactions.107 First, third generation of dendritic bisfuran monomers could be prepared by CuAAC “click” reaction, followed by thermally reversible polymerization by DA “click” reaction between the bisfurans and bismaleimides.
2.7 Cross-linked and mendable polymers
Utilization of the DA “click” reactions in the preparation of polymer networks has resulted in a new class of thermally reversible cross-linked polymers108 and hydrogels109 which are widely tested in encapsulants, self-healing materials and coatings applications.109–111 Three synthetic strategies can be employed for the synthesis of polymer networks, namely (i) direct DA cycloaddition reactions involving multifunctional monomers, e.g. a di- or tri-furan derivative and a bismaleimide,48,112–116 (ii) DA cycloaddition of linear polymers bearing pendant furan and/or maleimide111,117–132 and (iii) a cross-linker or an initiator containing a Diels–Alder linkage in the polymerization (Fig. 10).133,134
For the first strategy, Wudl and co-workers reported that the synthesis of a highly cross-linked polymer network can be formed via the DA cycloaddition reaction of a multi-diene (four furan-functionalized monomer) and multi-dienophile (three maleimide-functionalized monomer) and thermal reversibility of this network can be accomplished by the retro-DA reaction.135 For the second strategy, thermoresponsive hydrogels based on the aqueous Diels–Alder reaction of various polymer backbone bearing pendant furfuryl groups and PEO bismaleimide were reported by Wei and co-workers.136–138 For the last strategy, a cross-linker or an initiator containing a Diels–Alder linkage can be synthesized by a series of reactions involving DA, retro-DA cycloaddition and esterification. The thermally labile cross-linker or initiator was successfully converted to polymer networks via different polymerization methods.133,134 Recently, Barner-Kowollik et al. reported an alternative approach for the synthesis of a reversible cross-linked polymer via the HDA “click” reaction of biscyclopentadiene end-functionalized PMMA and trifunctional pyridinyldithioformate cross-linker.139 The reversibility of rapid HDA reactions allows regeneration of the highly reversibly stimuli-responsive cross-linked polymer network.
It is clear that DA “click” chemistry is the most frequently studied reaction for the synthesis of thermoreversible cross-linked networks including polymer gels and thermoset networks. This ‘thermoreversibility’ enables the fabrication of re-mendable polymers carrying diene and dienophile functionalities, either as the polymer chain-end or in the repeating units.140 In these polymers, when the fractured polymers are reheated, first DA linkages should disconnect but then the diene and dienophile moieties should reconnect upon cooling and the cracks, or fractures, should repair. This fracture/repair cycle can be repeated multiple times, and it does not require additional chemicals such as a catalyst, monomer, or special surface treatment of the fractured interface. There are a number of Diels–Alder systems based on furan–maleimide,23,124,132,135,141–147 cyclopentadiene148–155 and anthracene–maleimide134,156 couples for re-mendable polymers (Fig. 11). Recent reviews on re-mendable polymers are available in the literature.140,157–160
2.8 Side-chain functionalization
Side-chain functionalization is the process by which desired groups are introduced into the side chain unit of the polymer backbone. Typically side chain functional polymers can be simply prepared by DA “click” chemistry. In this context, Jen and co-workers have used the DA “click” reaction between polymer backbone bearing side-chain anthracene molecules and maleimide-containing nonlinear optical (NLO) chromophores to yield high-performance NLO polymers.161 DA “click” reaction was also applied using the opposite end functional (side-chain maleimide) polymer backbone and anthryl-containing chromophores.162 The resulting polymers exhibited high dielectric strength and thermal stability, excellent optical and poling efficiencies, and good processability. The facile access of these polymers highlighted the importance of the reagent-free DA “click” reactions. Moreover, DA click reactions have a significant advantage over the CuAAC “click” reaction because of the high reactivity of azides, towards cyano-containing acceptors that are the most used NLO chromophores.
Another example of side chain functionalization was the incorporation of photoactive groups onto polystyrene backbone by using a “double-click” chemistry strategy (Fig. 12).163 For this purpose, thioxanthone–anthracene, N-propargyl-7-oxynorbornene acted as a “click-linker”, and polystyrene with side-chain azide moieties were reacted in N,N-dimethylformamide for 36 h at 120 °C. The obtained polymeric photoinitiators were shown to efficiently initiate the free radical polymerization of mono- and multi-functional monomers via type II photoinitiation mechanism.
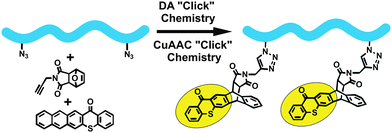 |
| Fig. 12 Side-chain functionalization of polymers via one-pot CuAAC and DA “click” reactions. | |
3 Bioconjugates and modification of biomolecules via DA “click” chemistry
The modification of biomolecules with synthetic polymers offers many advantages over unmodified counterparts, including increased stability and solubility, the ability to modulate protein activity, and increased bioavailability for therapeutic applications.164–166 The DA cycloaddition reactions are a promising tool for the bioconjugation of biomolecules since it occurs within a short period (hours), with very high yields and under mild conditions (at room temperature in aqueous solution). The high compatibility of DA cycloaddition reactions with biomolecules has been operated elegantly in the bioconjugation or immobilization of oligonucleotides, protein, peptides, carbohydrates and antibodies. For example, a number of studies described the use of DA “click” chemistry with oligonucleotides167 bearing a diene or dienophile moiety with small molecules (carboxylic acid, activated ester, benzophenone, pyrene, fluorescent dye, TEMPO, biotin, etc.),168–180 polymers,170,171,181 gold nanoparticles,171,182 and glass surfaces.183–187 The reactions were carried out without interference of the many functional groups presented in RNA and DNA. The yields were greater than 80% and often as much as 90–95% within several hours at room or slightly higher temperature (e.g., 30 °C).
The conjugation of diene-modified oligonucleotides with maleimide derivatives of peptides could also be done using DA cycloaddition reaction under mild conditions without additional catalysts (Fig. 13). The reaction was strongly affected by the size of the reagents and nature of dienes and dienophiles, but it was completed in 8–10 h with high yield.176,188,189 The DA cycloaddition reactions allowed the straightforward synthesis of large peptide–oligonucleotide conjugates, however, maleimide was prone to Michael addition reactions with other nucleophilic centers on peptides or oligonucleotides and may lead to side reactions.190
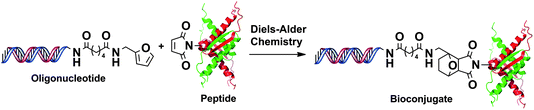 |
| Fig. 13 Preparation of peptide-oligonucleotide bioconjugates by DA “click” reaction. | |
The DA cycloaddition reactions can also offer many possibilities for the modification of peptides and proteins,191–194 due to the compatibility with all amino acid side groups except for the thiol group on cysteine.195 To prove the applicability of DA “click” chemistry, protein–cyclopentadiene conjugates were prepared and subsequently grafted onto maleimide modified glass,196,197 self-assembled monolayer,198–206 dendrimer207 or hydrophilic resin208 to give the cycloaddition product in high yield. The DA cycloaddition reactions have also been recognized as a promising chemoselective methodology to introduce fluorescent labels on proteins,28,29,197,209–212 to prepare neo-glycoproteins,213 or to chemically activated proteins.27,214–216
Recently, the Nolte group reported on an elegant spontaneous copper-free tandem 1,3-dipolar cycloaddition-retro-Diels–Alder (tandem crDA) reaction in which a trifluoromethyl-substituted oxanorbornadiene derivative reacts with an azide to form a stable 1,2,3-triazole linkage. The crDA reaction was successfully applied to the coupling of small molecules with proteins and polymers with peptides in various media at ambient temperature (Fig. 14). The key to the successful bioconjugation of this approach lay in the increased reactivity of the trifluoro-methyl substituted oxanorbornadiene derivatives as compared to the electron deficient alkynes. These molecules tended to react with azide under mild reaction conditions, even in the absence of transition metal catalysts like copper. It was proved that this crDA “click” reaction was faster than classical CuAAC “click” reaction at room temperature. Therefore, this method was successfully used in the modification of peptides and proteins. Later on, the crDA “click” reaction was tested in the biological media, indicating that the reaction was bioorthogonal and underwent independently of pH and also proceeded in serum and blood.212,217,218
An alternative bioorthogonal inverse-electron demand Diels–Alder reaction between strained trans-cyclooctene (dienophile) and s-tetrazine (diene) has been reported by several groups (Fig. 15).27–29,219–223 In this method, first the reaction sites of the diene and the dienophile were reacted in a [4 + 2] cycloaddition and then formation of nitrogen gaseous made this reaction irreversible and produces the stable dihydro-pyridazines. Because of its high reactivity in organic solvents, in water, and in cell media, it may be used as a tool for the covalent and irreversible derivatization of various molecules such as peptides,27 oligonucleotides222 and small-molecule drugs.29,220,223
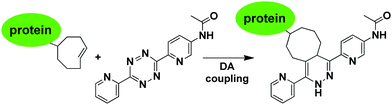 |
| Fig. 15 Functionalization of protein with tetrazole-based diene via inverse-electron demand Diels–Alder reaction. | |
3.3 Modification of sugars, polysaccharides, and glycoconjugates
Other biomaterials including carbohydrates and derivatives have been immobilized onto various substrates (such as SAM,224,225 protein,213,226 human serum albumin227 and glass228) by DA cycloaddition reactions.229,230 Chaikof and co-workers reported on a protocol using sequential double “click” reactions (DA followed by CuAAC) for the immobilization of carbohydrates and proteins onto a solid surface. First, a PEO linker carrying alkyne and cyclodiene end groups was synthesized and attached onto an N-(ε-maleimidocaproyl)-functionalized (EMC) glass plate via an aqueous DA reaction. Subsequently, an alkyne-functionalized surface was provided for the conjugation of azide-containing biomolecules (proteins, biotin, and sugars) via CuAAC “click” chemistry, which proceeded to completion at low temperature and in aqueous solvent. The reactants, azide, alkyne, cyclodiene, and EMC groups were independently stable under bioconjugation conditions and do not react with common organic reagents or with themselves. Thus the potential of this strategy to immobilize a wide range of functionally complex substances onto solid surfaces has been significant.231 Later on, the same group has extended to use of aqueous DA “click” chemistry for the immobilization of diverse biomolecules such as biotin, lactose and protein A onto EMC glass plate.183
DA “click” chemistry has been recently employed for bioconjugation of maleimide-functional antibodies onto furan-functional polymeric nanoparticle surfaces.232–235 The self-assembled polymeric nanoparticles were designed from poly(2-methyl, 2-carboxy trimethylene carbonate-co-D,L-lactide)-graft-poly(ethylene glycol) with furan groups located on the surface of the PEO corona. Coupling of maleimide-functional antibodies on the nanoparticle surface via DA chemistry led to the immuno-nanoparticles, which are promising tools for antibody-mediated targeted drug delivery. The DA “click” coupling reaction was performed in aqueous media (pH 5.5) at 37 °C achieving high coupling efficiency of antibody of up to 100% within several hours.
4 Hybrid materials via DA “click” chemistry
Owing to the efficiency and facile work-up, DA cycloaddition reactions have been extensively used for the functionalization of organic/inorganic nanostructures and surfaces.45,236 The surface modification of divinylbenzene based microspheres and aromatic polyester by grafting 2,4-hexadienoyl-terminated poly(ε-caprolactone) and maleimide-terminated PEO chains afforded functional microspheres42 and hydrophilic polymers.237 In another approach, divinylbenzene based microspheres were firstly functionalized with a highly reactive diene (cyclopentadiene), and subsequently reacted with thiocarbonyl terminated polystyrene through HDA “click” reaction.238
The external graphene planes of carbon black,239,240 nano-tubes241–250 and fibers251–254 make these materials particularly suited for functionalization by DA cycloaddition reactions to their π-electrons. Abetz et al. have also shown that these carbon-rich materials behave both as diene and dienophile, depending on the reaction partner.255 Preliminary results indicated that single walled carbon nanotubes were more reactive than corresponding multiwall tubes while the carbon nanofibers were the least reactive, presumably due to the less available surface area. For example, consecutive DA cycloadditions of 1,3-butadienes onto the carbon nanofiber led to a polymeric layer on the surface (Fig. 16).252–254 This process offers a new and powerful methodology to obtain functional carbon nano-materials with better solubility for the technological applications.
 |
| Fig. 16 Surface modification of carbon nanotubes with organic molecules via Diels–Alder reactions. | |
Similarly, DA and HDA click reactions have been also used for selective modification of fullerenes.240,256–260 For example, Barner-Kowollik and Nebhani reported a direct and rapid functionalization of fullerene with cyclopentadienyl functionalized PEO via DA reaction ambient temperature in the absence of any catalyst (Fig. 17).259 In another case, DA “click” reaction was applied to synthesis of polymer hybrid materials from organic molecules onto silicon wafers,261,262 gold nanoparticles263–265 or tetraethoxysilanes.147,266
5 Conclusions and perspectives
In conclusion, DA “click” reactions show great potential for the construction of tailor-made functional materials such as telechelic polymers, block, graft, star, star-block, H-shaped polymers, dendrimers, bioconjugates and hybrid materials. DA “click” chemistry features several outstanding advantages including water-solubility, high reaction yields, no detectable side-reactions and no requirement for additional catalysts. Moreover, the reactive moieties of this method are inert for most functionalities such as –COOH, –OH and –NH2 groups and biomolecules. It can be applied under particularly mild conditions (aqueous solution without solvents, neutral pH and moderate temperature). Another considerable advantage of these reactions is commercial availability of the suitable dienophiles in the form of maleimide-derivatives.
Overall, the DA “click” chemistry is a simple synthetic route to access organic and hybrid materials. While maleimides are used as dienophiles in most of the DA reactions developing new dienes and dienophiles should upgrade its ability in novel applications. On the other hand, sometimes longer reaction times are required for the complete conversion, which may be overcome by addition of suitable catalysts.
Notes and references
- O. Diels and K. Alder, Justus Liebigs Ann. Chem., 1928, 460, 98–122 CrossRef CAS
.
- J. Sauer and R. Sustmann, Angew. Chem., Int. Ed. Engl., 1980, 19, 779–807 CrossRef
.
- K. C. Nicolaou, S. A. Snyder, T. Montagnon and G. Vassilikogiannakis, Angew. Chem., Int. Ed., 2002, 41, 1668–1698 CrossRef CAS
.
- L. F. Tietze and G. Kettschau, Top. Curr. Chem., 1997, 189, 1–120 CAS
.
- J. L. Ripoll, A. Rouessac and F. Rouessac, Tetrahedron, 1978, 34, 19–40 CrossRef CAS
.
- H. C. Kolb, M. G. Finn and K. B. Sharpless, Angew. Chem., Int. Ed., 2001, 40, 2004–2021 CrossRef CAS
.
- V. V. Rostovtsev, L. G. Green, V. V. Fokin and K. B. Sharpless, Angew. Chem., Int. Ed., 2002, 41, 2596–2599 CrossRef CAS
.
- C. W. Tornoe, C. Christensen and M. Meldal, J. Org. Chem., 2002, 67, 3057–3064 CrossRef CAS
.
- B. S. Sumerlin and A. P. Vogt, Macromolecules, 2010, 43, 1–13 CrossRef CAS
.
- G. Kumaraswamy, K. Ankamma and A. Pitchaiah, J. Org. Chem., 2007, 72, 9822–9825 CrossRef CAS
.
- K. L. Heredia, Z. P. Tolstyka and H. D. Maynard, Macromolecules, 2007, 40, 4772–4779 CrossRef CAS
.
- A. Dirksen and P. E. Dawson, Bioconjugate Chem., 2008, 19, 2543–2548 CrossRef CAS
.
- L. M. Campos, K. L. Killops, R. Sakai, J. M. J. Paulusse, D. Damiron, E. Drockenmuller, B. W. Messmore and C. J. Hawker, Macromolecules, 2008, 41, 7063–7070 CrossRef CAS
.
- K. L. Killops, L. M. Campos and C. J. Hawker, J. Am. Chem. Soc., 2008, 130, 5062–5064 CrossRef CAS
.
- S. P. S. Koo, M. M. Stamenovic, R. A. Prasath, A. J. Inglis, F. E. Du Prez, C. Barner-Kowollik, W. Van Camp and T. Junkers, J. Polym. Sci., Part A: Polym. Chem., 2010, 48, 1699–1713 CrossRef CAS
.
- R. A. Prasath, M. T. Gokmen, P. Espeel and F. E. Du Prez, Polym. Chem., 2010, 1, 685–692 RSC
.
- J. F. Lutz, Angew. Chem., Int. Ed., 2007, 46, 1018–1025 CrossRef CAS
.
- A. J. Inglis and C. Barner-Kowollik, Macromol. Rapid Commun., 2010, 31, 1247–1266 CrossRef CAS
.
- C. Barner-Kowollik, F. E. Du Prez, P. Espeel, C. J. Hawker, T. Junkers, H. Schlaad and W. Van Camp, Angew. Chem., Int. Ed., 2011, 50, 60–62 CrossRef CAS
.
- M. L. Szalai, D. V. McGrath, D. R. Wheeler, T. Zifer and J. R. McElhanon, Macromolecules, 2007, 40, 818–823 CrossRef CAS
.
- A. Robertson, D. Philp and N. Spencer, Tetrahedron, 1999, 55, 11365–11384 CrossRef CAS
.
- J. R. McElhanon and D. R. Wheeler, Org. Lett., 2001, 3, 2681–2683 CrossRef CAS
.
- Y. Chujo, K. Sada and T. Saegusa, Macromolecules, 1990, 23, 2636–2641 CrossRef CAS
.
- H. Durmaz, B. Colakoclu, U. Tunca and G. Hizal, J. Polym. Sci., Part A: Polym. Chem., 2006, 44, 1667–1675 CrossRef CAS
.
- B. Gacal, H. Durmaz, M. A. Tasdelen, G. Hizal, U. Tunca, Y. Yagci and A. L. Demirel, Macromolecules, 2006, 39, 5330–5336 CrossRef CAS
.
- A. J. Inglis, S. Sinnwell, M. H. Stenzel and C. Barner-Kowollik, Angew. Chem., Int. Ed., 2009, 48, 2411–2414 CAS
.
- M. L. Blackman, M. Royzen and J. M. Fox, J. Am. Chem. Soc., 2008, 130, 13518–13519 CrossRef CAS
.
- N. K. Devaraj, R. Weissleder and S. A. Hilderbrand, Bioconjugate Chem., 2008, 19, 2297–2299 CrossRef CAS
.
- N. K. Devaraj, R. Upadhyay, J. B. Hatin, S. A. Hilderbrand and R. Weissleder, Angew. Chem., Int. Ed., 2009, 48, 7013–7016 CrossRef CAS
.
- Z. Li, H. Cai, M. Hassink, M. L. Blackman, R. C. D. Brown, P. S. Conti and J. M. Fox, Chem. Commun., 2010, 46, 8043–8045 RSC
.
- G. Franc and A. K. Kakkar, Chem.–Eur. J., 2009, 15, 5630–5639 CrossRef CAS
.
- A. Sanyal, Macromol. Chem. Phys., 2010, 211, 1417–1425 CrossRef CAS
.
- C. R. Becer, R. Hoogenboom and U. S. Schubert, Angew. Chem., Int. Ed., 2009, 48, 4900–4908 CrossRef CAS
.
- J. F. Lutz, Angew. Chem., Int. Ed., 2008, 47, 2182–2184 CrossRef CAS
.
- R. K. Iha, K. L. Wooley, A. M. Nystrom, D. J. Burke, M. J. Kade and C. J. Hawker, Chem. Rev., 2009, 109, 5620–5686 CrossRef CAS
.
- U. Mansfeld, C. Pietsch, R. Hoogenboom, C. R. Becer and U. S. Schubert, Polym. Chem., 2010, 1, 1560–1598 RSC
.
- S. Sinnwell, A. J. Inglis, T. P. Davis, M. H. Stenzel and C. Barner-Kowollik, Chem. Commun., 2008, 2052–2054 RSC
.
- A. J. Inglis, S. Sinnwell, T. P. Davis, C. Barner-Kowollik and M. H. Stenzel, Macromolecules, 2008, 41, 4120–4126 CrossRef CAS
.
- A. Dag, H. Durmaz, E. Demir, G. Hizal and U. Tunca, J. Polym. Sci., Part A: Polym. Chem., 2008, 46, 6969–6977 CrossRef CAS
.
- O. Altintas, G. Hizal and U. Tunca, J. Polym. Sci., Part A: Polym. Chem., 2008, 46, 1218–1228 CrossRef CAS
.
- O. Altintas, G. Hizal and U. Tunca, J. Polym. Sci., Part A: Polym. Chem., 2006, 44, 5699–5707 CrossRef CAS
.
- L. Nebhani, S. Sinnwell, A. J. Inglis, M. H. Stenzel, C. Barner-Kowollik and L. Barner, Macromol. Rapid Commun., 2008, 29, 1431–1437 CrossRef CAS
.
- H. Durmaz, A. Dag, O. Altintas, T. Erdogan, G. Hizal and U. Tunca, Macromolecules, 2007, 40, 191–198 CrossRef CAS
.
- J. P. Kennedy and G. M. Carlson, J. Polym. Sci., Part A: Polym. Chem., 1983, 21, 3551–3561 Search PubMed
.
- G. W. Goodall and W. Hayes, Chem. Soc. Rev., 2006, 35, 280–312 RSC
.
- C. D. Diakoumakos and J. A. Mikroyannidis, J. Polym. Sci., Part A: Polym. Chem., 1992, 30, 2559–2567 CrossRef CAS
.
- J. A. Mikroyannidis, J. Polym. Sci., Part A: Polym. Chem., 1992, 30, 125–132 CrossRef CAS
.
- C. Gousse and A. Gandini, Polym. Int., 1999, 48, 723–731 CrossRef CAS
.
- K. Kamahori, S. Tada, K. Ito and S. Itsuno, Macromolecules, 1999, 32, 541–547 CrossRef CAS
.
- S. E. Mallakpour, A. R. Hajipour, A. R. Mahdavian and S. Khoee, J. Appl. Polym. Sci., 2000, 76, 240–248 Search PubMed
.
- J. A. Mikroyannidis, J. Polym. Sci., Part A: Polym. Chem., 1992, 30, 2017–2024 Search PubMed
.
- G. Alhakimi, H. Gorls and E. Klemm, Macromol. Chem. Phys., 1994, 195, 1569–1576 CrossRef CAS
.
- U. Kumar and T. X. Neenan, ACS Symp. Ser., 1995, 614, 518–526 Search PubMed
.
- H. Ben Romdhane, M. Baklouti, M. R. Chaabouni, M. F. Grenier-Loustalot, F. Delolme and B. Sillion, Polymer, 2002, 43, 255–268 CrossRef
.
- G. Alhakimi, E. Klemm and H. Görls, J. Polym. Sci., Part A: Polym. Chem., 1995, 33, 1133–1142 CrossRef CAS
.
- J. K. Stille and G. K. Noren, Macromolecules, 1972, 5, 49–55 CrossRef CAS
.
- C. L. Schilling, J. A. Reed and J. K. Stille, Macromolecules, 1969, 2, 85–88 CrossRef CAS
.
- J. K. Stille, R. O. Rakutis, H. Mukamal and F. W. Harris, Macromolecules, 1968, 1, 431–436 CrossRef CAS
.
- H. Mukamal, F. W. Harris and J. K. Stille, J. Polym. Sci., Part A-1, 1967, 5, 2721–2729 CrossRef CAS
.
- A. D. Schluter, Adv. Mater., 1991, 3, 282–291 CrossRef
.
- U. Scherf and K. Mullen, Adv. Polym. Sci., 1995, 123, 1–40 CAS
.
- N. Teramoto, Y. Arai and M. Shibata, Carbohydr. Polym., 2006, 64, 78–84 Search PubMed
.
- J. K. Stille and L. Plummer, J. Org. Chem., 1961, 26, 4026–4029 CrossRef CAS
.
- E. S. Krongauz, Usp. Khim., 1973, 42, 1854–1891 Search PubMed
.
- M. Grigoras, M. Sava, G. Colotin and C. I. Simionescu, J. Appl. Polym. Sci., 2008, 107, 846–853 Search PubMed
.
- T. Brand and M. Klapper, Des. Monomers Polym., 1999, 2, 287–309 Search PubMed
.
- K. B. Wagener and L. P. Engle, J. Polym. Sci., Part A: Polym. Chem., 1993, 31, 865–875 Search PubMed
.
- M. A. Tasdelen, M. U. Kahveci and Y. Yagci, Prog. Polym. Sci., 2011, 36, 455–567 CrossRef CAS
.
- G. Mantovani, F. Lecolley, L. Tao, D. M. Haddleton, J. Clerx, J. Cornelissen and K. Velonia, J. Am. Chem. Soc., 2005, 127, 2966–2973 CrossRef CAS
.
- H. Durmaz, F. Karatas, U. Tunca and G. Hizal, J. Polym. Sci., Part A: Polym. Chem., 2006, 44, 3947–3957 CrossRef CAS
.
- M. Li, P. De, S. R. Gondi and B. S. Sumerlin, J. Polym. Sci., Part A: Polym. Chem., 2008, 46, 5093–5100 CrossRef CAS
.
- H. Akat, B. Gacal, D. K. Balta, N. Arsu and Y. Yagci, J. Polym. Sci., Part A: Polym. Chem., 2010, 48, 2109–2114 Search PubMed
.
- O. Altintas, G. Hizal and U. Tunca, Des. Monomers Polym., 2009, 12, 83–98 Search PubMed
.
- K. Masutani, S. Kawabata, T. Aoki and Y. Kimura, Polym. Int., 2010, 59, 1526–1530 Search PubMed
.
- K. A. Jorgensen, Angew. Chem., Int. Ed., 2000, 39, 3558–3588 CrossRef CAS
.
- H. Waldmann, Synthesis, 1994, 535–551 CrossRef CAS
.
- L. Nebhani, S. Sinnwell, C. Y. Lin, M. L. Coote, M. H. Stenzel and C. Barner-Kowollik, J. Polym. Sci., Part A: Polym. Chem., 2009, 47, 6053–6071 CrossRef CAS
.
- S. Sinnwell, C. V. Synatschke, T. Junkers, M. H. Stenzel and C. Barner-Kowollik, Macromolecules, 2008, 41, 7904–7912 CrossRef CAS
.
- A. J. Inglis and C. Barner-Kowollik, Polym. Chem., 2011, 2, 126–136 RSC
.
- M. Glassner, J. P. Blinco and C. Barner-Kowollik, Polym. Chem., 2011, 2, 83–87 RSC
.
- A. J. Inglis, M. H. Stenzel and C. Barner-Kowollik, Macromol. Rapid Commun., 2009, 30, 1792–1798 CrossRef CAS
.
- H. Durmaz, A. Dag, O. Altintas, T. Erdogan, G. Hizal and U. Tunca, Macromolecules, 2007, 40, 191–198 CrossRef CAS
.
- A. Bousquet, C. Barner-Kowollik and M. H. Stenzel, J. Polym. Sci., Part A: Polym. Chem., 2010, 48, 1773–1781 CrossRef CAS
.
- A. Bousquet, C. Boyer, T. P. Davis and M. H. Stenzel, Polym. Chem., 2010, 1, 1186–1195 RSC
.
- H. Durmaz, A. Dag, N. Cerit, O. Sirkecioglu, G. Hizal and U. Tunca, J. Polym. Sci., Part A: Polym. Chem., 2010, 48, 5982–5991 Search PubMed
.
- A. Dag, H. Sahin, H. Durmaz, G. Hizal and U. Tunca, J. Polym. Sci., Part A: Polym. Chem., 2011, 49, 886–892 Search PubMed
.
- A. Dag, H. Durmaz, G. Hizal and U. Tunca, J. Polym. Sci., Part A: Polym. Chem., 2008, 46, 302–313 CrossRef CAS
.
- H. Durmaz, F. Karatas, U. Tunca and G. Hizal, J. Polym. Sci., Part A: Polym. Chem., 2006, 44, 499–509 CrossRef CAS
.
- H. Durmaz, A. Dag, G. Hizal and U. Tunca, J. Polym. Sci., Part A: Polym. Chem., 2011, 49, 1195–1200 Search PubMed
.
- E. Gungor, G. Hizal and U. Tunca, J. Polym. Sci., Part A: Polym. Chem., 2009, 47, 3409–3418 CrossRef CAS
.
- X. Q. Xiong and Y. H. Xu, Polym. Bull., 2010, 65, 455–463 CrossRef CAS
.
- H. Durmaz, A. Dag, A. Hizal, G. Hizal and U. Tunca, J. Polym. Sci., Part A: Polym. Chem., 2008, 46, 7091–7100 CrossRef CAS
.
- A. Dag, H. Durmaz, U. Tunca and G. Hizal, J. Polym. Sci., Part A: Polym. Chem., 2009, 47, 178–187 CrossRef CAS
.
- H. Durmaz, A. Dag, D. Gursoy, A. L. Demirel, G. Hizal and U. Tunca, J. Polym. Sci., Part A: Polym. Chem., 2010, 48, 1557–1564 CrossRef CAS
.
- S. Sinnwell, A. J. Inglis, M. H. Stenzel and C. Barner-Kowollik, Macromol. Rapid Commun., 2008, 29, 1090–1096 CrossRef CAS
.
- H. Durmaz, A. Dag, C. Onen, O. Gok, A. Sanyal, G. Hizal and U. Tunca, J. Polym. Sci., Part A: Polym. Chem., 2010, 48, 4842–4846 Search PubMed
.
- A. Dag, H. Durmaz, V. Kirmizi, G. Hizal and U. Tunca, Polym. Chem., 2010, 1, 621–623 RSC
.
- S. Sinnwell, M. Lammens, M. H. Stenzel, F. E. Du Prez and C. Barner-Kowollik, J. Polym. Sci., Part A: Polym. Chem., 2009, 47, 2207–2213 CrossRef CAS
.
- H. Durmaz, A. Dag, G. Hizal and U. Tunca, J. Polym. Sci., Part A: Polym. Chem., 2010, 48, 5083–5091 CrossRef CAS
.
- C. Kim, H. Kim and K. Park, J. Organomet. Chem., 2005, 690, 4794–4800 CrossRef CAS
.
- C. Kim, K. I. Lim and C. G. Song, J. Organomet. Chem., 2005, 690, 3278–3285 CrossRef CAS
.
- H. J. Son, W. S. Han, H. Kim, C. Kim, J. Ko, C. Lee and S. O. Kang, Organometallics, 2006, 25, 766–774 CrossRef CAS
.
- C. Kim, H. Kim and K. Park, J. Organomet. Chem., 2003, 667, 96–102 Search PubMed
.
- M. M. Kose, G. Yesilbag and A. Sanyal, Org. Lett., 2008, 10, 2353–2356 CrossRef CAS
.
- M. Tonga, N. Cengiz, M. M. Kose, T. Dede and A. Sanyal, J. Polym. Sci., Part A: Polym. Chem., 2010, 48, 410–416 CrossRef CAS
.
- A. Vieyres, T. Lam, R. Gillet, G. Franc, A. Castonguay and A. Kakkar, Chem. Commun., 2010, 46, 1875–1877 RSC
.
- N. W. Polaske, D. V. McGrath and J. R. McElhanon, Macromolecules, 2010, 43, 1270–1276 CrossRef CAS
.
- A. A. Kavitha and N. K. Singha, ACS Appl. Mater. Interfaces, 2009, 1, 1427–1436 CrossRef CAS
.
- H. L. Wei, Z. Yang, L. M. Zheng and Y. M. Shen, Polymer, 2009, 50, 2836–2840 CrossRef CAS
.
- S. A. Canary and M. P. Stevens, J. Polym. Sci., Part A: Polym. Chem., 1992, 30, 1755–1760 CrossRef CAS
.
- E. Goiti, M. B. Huglin and J. M. Rego, Eur. Polym. J., 2004, 40, 219–226 CrossRef CAS
.
- C. Gousse and A. Gandini, Polym. Bull., 1998, 40, 389–394 CrossRef CAS
.
- F. Ghezzo, D. R. Smith, T. N. Starr, T. Perram, A. F. Starr, T. K. Darlington, R. K. Baldwin and S. J. Oldenburg, J. Compos. Mater., 2010, 44, 1587–1603 Search PubMed
.
- K. Inoue, M. Yamashiro and M. Iji, J. Appl. Polym. Sci., 2009, 112, 876–885 CrossRef CAS
.
- B. J. Adzima, H. A. Aguirre, C. J. Kloxin, T. F. Scott and C. N. Bowman, Macromolecules, 2008, 41, 9112–9117 CrossRef CAS
.
- T. Nemoto and G.-i. Konishi, Polym. J., 2008, 40, 651–656 Search PubMed
.
- H. Laita, S. Boufi and A. Gandini, Eur. Polym. J., 1997, 33, 1203–1211 CrossRef
.
- C. Gousse, A. Gandini and P. Hodge, Macromolecules, 1998, 31, 314–321 CrossRef CAS
.
- S. Magana, A. Zerroukhi, C. Jegat and N. Mignard, React. Funct. Polym., 2010, 70, 442–448 Search PubMed
.
- Y. L. Liu, C. Y. Hsieh and Y. W. Chen, Polymer, 2006, 47, 2581–2586 CrossRef
.
- Y. Imai, H. Itoh, K. Naka and Y. Chujo, Macromolecules, 2000, 33, 4343–4346 CrossRef CAS
.
- A. A. Kavitha and N. K. Singha, Macromolecules, 2010, 43, 3193–3205 CrossRef CAS
.
- R. Gheneim, C. Perez-Berumen and A. Gandini, Macromolecules, 2002, 35, 7246–7253 CrossRef CAS
.
- M. Watanabe and N. Yoshie, Polymer, 2006, 47, 4946–4952 CrossRef CAS
.
- Z. W. Shi, W. Liang, J. D. Luo, S. Huang, B. M. Polishak, X. S. Li, T. R. Younkin, B. A. Block and A. K. Y. Jen, Chem. Mater., 2010, 22, 5601–5608 Search PubMed
.
- Q. A. Tian, M. Z. Rong, M. Q. Zhang and Y. C. Yuan, Polym. Int., 2010, 59, 1339–1345 Search PubMed
.
- J. Canadell, H. Fischer, G. De With and R. A. T. M. van Benthem, J. Polym. Sci., Part A: Polym. Chem., 2010, 48, 3456–3467 CrossRef CAS
.
- B. J. Adzima, C. J. Kloxin and C. N. Bowman, Adv. Mater., 2010, 22, 2784–2787 Search PubMed
.
- A. M. Peterson, R. E. Jensen and G. R. Palmese, ACS Appl. Mater. Interfaces, 2010, 2, 1141–1149 CrossRef CAS
.
- K. Ishida and N. Yoshie, Macromol. Biosci., 2008, 8, 916–922 CrossRef CAS
.
- A. Amalin Kavitha and N. K. Singha, J. Polym. Sci., Part A: Polym. Chem., 2007, 45, 4441–4449 CrossRef CAS
.
- Y.-L. Liu and Y.-W. Chen, Macromol. Chem. Phys., 2007, 208, 224–232 CrossRef CAS
.
- W. H. Heath, F. Palmieri, J. R. Adams, B. K. Long, J. Chute, T. W. Holcombe, S. Zieren, M. J. Truitt, J. L. White and C. G. Willson, Macromolecules, 2008, 41, 719–726 CrossRef CAS
.
- J. A. Syrett, G. Mantovani, W. R. S. Barton, D. Price and D. M. Haddleton, Polym. Chem., 2010, 1, 102–106 RSC
.
- X. X. Chen, M. A. Dam, K. Ono, A. Mal, H. B. Shen, S. R. Nutt, K. Sheran and F. Wudl, Science, 2002, 295, 1698–1702 CrossRef CAS
.
- H.-L. Wei, Z. Yang, Y. Chen, H.-J. Chu, J. Zhu and Z.-C. Li, Eur. Polym. J., 2010, 46, 1032–1039 Search PubMed
.
- H.-L. Wei, Z. Yang, L.-M. Zheng and Y.-M. Shen, Polymer, 2009, 50, 2836–2840 CrossRef CAS
.
- H.-L. Wei, Z. Yang, H.-J. Chu, J. Zhu, Z.-C. Li and J.-S. Cui, Polymer, 2010, 51, 1694–1702 Search PubMed
.
- A. J. Inglis, L. Nebhani, O. Altintas, F. G. Schmidt and C. Barner-Kowollik, Macromolecules, 2010, 43, 5515–5520 CrossRef CAS
.
- J. A. Syrett, C. R. Becer and D. M. Haddleton, Polym. Chem., 2010, 1, 978–987 RSC
.
- Y. Zhang, A. A. Broekhuis and F. Picchioni, Macromolecules, 2009, 42, 1906–1912 CrossRef CAS
.
- A. M. Peterson, R. E. Jensen and G. R. Palmese, ACS Appl. Mater. Interfaces, 2010, 2, 1141–1149 CrossRef CAS
.
- A. Gandini, D. Coelho and A. J. D. Silvestre, Eur. Polym. J., 2008, 44, 4029–4036 CrossRef CAS
.
- A. Gandini, A. J. D. Silvestre and D. Coelho, J. Polym. Sci., Part A: Polym. Chem., 2010, 48, 2053–2056 CrossRef CAS
.
- X. X. Chen, F. Wudl, A. K. Mal, H. B. Shen and S. R. Nutt, Macromolecules, 2003, 36, 1802–1807 CrossRef CAS
.
- Y. L. Liu and C. Y. Hsieh, J. Polym. Sci., Part A: Polym. Chem., 2006, 44, 905–913 CrossRef CAS
.
- B. Gotsmann, U. Duerig, J. Frommer and C. J. Hawker, Adv. Funct. Mater., 2006, 16, 1499–1505 CrossRef CAS
.
- J. S. Park, K. Takahashi, Z. H. Guo, Y. Wang, E. Bolanos, C. Hamann-Schaffner, E. Murphy, F. Wudl and H. T. Hahn, J. Compos. Mater., 2008, 42, 2869–2881 CrossRef CAS
.
- A. S. Jones, J. D. Rule, J. S. Moore, S. R. White and N. R. Sottos, Chem. Mater., 2006, 18, 1312–1317 CrossRef CAS
.
- J. D. Rule, E. N. Brown, N. R. Sottos, S. R. White and J. S. Moore, Adv. Mater., 2005, 17, 205 CrossRef CAS
.
- J. W. C. Pang and I. P. Bond, Compos. Sci. Technol., 2005, 65, 1791–1799 CrossRef CAS
.
- E. N. Brown, S. R. White and N. R. Sottos, J. Mater. Sci., 2004, 39, 1703–1710 CrossRef CAS
.
- E. B. Murphy, E. Bolanos, C. Schaffner-Hamann, F. Wudl, S. R. Nutt and M. L. Auad, Macromolecules, 2008, 41, 5203–5209 CrossRef CAS
.
- J. P. Kennedy and K. F. Castner, J. Polym. Sci., Part A: Polym. Chem., 1979, 17, 2055–2070 Search PubMed
.
- J. P. Kennedy and K. F. Castner, J. Polym. Sci., Part A: Polym. Chem., 1979, 17, 2039–2054 Search PubMed
.
- J. R. Jones, C. L. Liotta, D. M. Collard and D. A. Schiraldi, Macromolecules, 1999, 32, 5786–5792 CrossRef CAS
.
- S. D. Bergman and F. Wudl, J. Mater. Chem., 2008, 18, 41–62 RSC
.
- R. P. Wool, Adv. Polym. Sci., 2008, 4, 400–418 Search PubMed
.
- D. Y. Wu, S. Meure and D. Solomon, Prog. Polym. Sci., 2008, 33, 479–522 CrossRef CAS
.
- S. Burattini, B. W. Greenland, D. Chappell, H. M. Colquhoun and W. Hayes, Chem. Soc. Rev., 2010, 39, 1973–1985 RSC
.
- T. D. Kim, J. D. Luo, Y. Q. Tian, J. W. Ka, N. M. Tucker, M. Haller, J. W. Kang and A. K. Y. Jen, Macromolecules, 2006, 39, 1676–1680 CrossRef CAS
.
- Z. W. Shi, J. D. Luo, S. Huang, Y. J. Cheng, T. D. Kim, B. M. Polishak, X. H. Zhou, Y. Q. Tian, S. H. Jang, D. B. Knorr, R. M. Overney, T. R. Younkin and A. K. Y. Jen, Macromolecules, 2009, 42, 2438–2445 CrossRef CAS
.
- B. Gacal, H. Akat, D. K. Balta, N. Arsu and Y. Yagci, Macromolecules, 2008, 41, 2401–2405 CrossRef CAS
.
- A. S. Hoffman and P. S. Stayton, Macromol. Symp., 2004, 207, 139–152 CrossRef CAS
.
- R. Duncan, Nat. Rev. Drug Discovery, 2003, 2, 347–360 CrossRef CAS
.
- F. M. Veronese, Biomaterials, 2001, 22, 405–417 CrossRef CAS
.
- S. H. Weisbrod and A. Marx, Chem. Commun., 2008, 5675–5685 RSC
.
- D. Graham and A. Enright, Curr. Org. Synth., 2006, 3, 9–17 Search PubMed
.
- F. Buller, L. Mannocci, Y. X. Zhang, C. E. Dumelin, J. Scheuermann and D. Neri, Bioorg. Med. Chem. Lett., 2008, 18, 5926–5931 Search PubMed
.
- K. W. Hill, J. Taunton-Rigby, J. D. Carter, E. Kropp, K. Vagle, W. Pieken, D. P. C. McGee, G. M. Husar, M. Leuck, D. J. Anziano and D. P. Sebesta, J. Org. Chem., 2001, 66, 5352–5358 CrossRef CAS
.
- G. M. Husar, D. J. Anziano, M. Leuck and D. P. Sebesta, Nucleosides, Nucleotides Nucleic Acids, 2001, 20, 559–566 CrossRef CAS
.
- D. Graham, L. Fruk and W. E. Smith, Analyst, 2003, 128, 692–699 RSC
.
- L. Fruk, A. Grondin, W. E. Smith and D. Graham, Chem. Commun., 2002, 2100–2101 RSC
.
- V. Borsenberger and S. Howorka, Nucleic Acids Res., 2009, 37, 1477–1485 CrossRef CAS
.
- R. Tona and R. Haner, Chem. Commun., 2004, 1908–1909 RSC
.
- R. Tona and R. Haner, Bioconjugate Chem., 2005, 16, 837–842 CrossRef CAS
.
- D. Graham, A. Grondin, C. McHugh, L. Fruk and W. E. Smith, Tetrahedron Lett., 2002, 43, 4785–4788 CrossRef CAS
.
- A. Okamoto, T. Taiji, K. Tainaka and I. Saito, Bioorg. Med. Chem. Lett., 2002, 12, 1895–1896 CrossRef CAS
.
- K. Stevens and A. Madder, Nucleosides, Nucleotides Nucleic Acids, 2007, 26, 1359–1362 CrossRef CAS
.
- E. Anderson and D. Picken, Nucleosides, Nucleotides Nucleic Acids, 2005, 24, 761–765 CrossRef CAS
.
- B. Seelig and A. Jaschke, Tetrahedron Lett., 1997, 38, 7729–7732 CrossRef CAS
.
- M. Proupin-Perez, R. Cosstick, L. M. Liz-Marzan, V. Salgueirino-Maceira and M. Brust, Nucleosides, Nucleotides Nucleic Acids, 2005, 24, 1075–1079 Search PubMed
.
- X. L. Sun, L. C. Yang and E. L. Chaikof, Tetrahedron Lett., 2008, 49, 2510–2513 Search PubMed
.
- H. A. Latham-Timmons, A. Wolter, J. S. Roach, R. Giare and M. Leuck, Nucleosides, Nucleotides Nucleic Acids, 2003, 22, 1495–1497 CrossRef CAS
.
- C. Heise and F. F. Bier, Top. Curr. Chem., 2005, 261, 1–25 CAS
.
- M. Abramov, G. Schepers, A. Van Aerschot, P. Van Hummelen and P. Herdewijn, Biosens. Bioelectron., 2008, 23, 1728–1732 Search PubMed
.
- R. Schlapak, P. Pammer, D. Armitage, R. Zhu, P. Hinterdorfer, M. Vaupel, T. Fruhwirth and S. Howorka, Langmuir, 2006, 22, 277–285 CrossRef CAS
.
- V. Marchan, S. Ortega, D. Pulido, E. Pedroso and A. Grandas, Nucleic Acids Res., 2006, 34, 1668–1668 Search PubMed
.
- V. Steven and D. Graham, Org. Biomol. Chem., 2008, 6, 3781–3787 RSC
.
- K. Lu, Q.P. Duan, L. Ma and D. X. Zhao, Bioconjugate Chem., 2010, 21, 187–202 CrossRef CAS
.
- C. P. R. Hackenberger and D. Schwarzer, Angew. Chem., Int. Ed., 2008, 47, 10030–10074 CrossRef CAS
.
- M. Zerial and H. McBride, Nat. Rev. Mol. Cell Biol., 2001, 2, 107–117 CrossRef CAS
.
- P. C. Lin, D. Weinrich and H. Waldmann, Macromol. Chem. Phys., 2010, 211, 136–144 CrossRef CAS
.
- J. M. Palomo, Eur. J. Org. Chem., 2010, 6303–6314 CrossRef CAS
.
- M. A. Gauthier and H. A. Klok, Chem. Commun., 2008, 2591–2611 RSC
.
- A. D. de Araujo, J. M. Palomo, J. Cramer, M. Kohn, H. Schroder, R. Wacker, C. Niemeyer, K. Alexandrov and H. Waldmann, Angew. Chem., Int. Ed., 2006, 45, 296–301 CrossRef CAS
.
- A. D. de Araujo, J. M. Palomo, J. Cramer, O. Seitz, K. Alexandrov and H. Waldmann, Chem.–Eur. J., 2006, 12, 6095–6109 CrossRef CAS
.
- M. N. Yousaf and M. Mrksich, J. Am. Chem. Soc., 1999, 121, 4286–4287 CrossRef CAS
.
- M. N. Yousaf, E. W. L. Chan and M. Mrksich, Angew. Chem., Int. Ed., 2000, 39, 1943–1946 CrossRef CAS
.
- E. W. L. Chan, M. N. Yousaf and M. Mrksich, J. Phys. Chem. A, 2000, 104, 9315–9320 CrossRef CAS
.
- M. N. Yousaf, B. T. Houseman and M. Mrksich, Angew. Chem., Int. Ed., 2001, 40, 1093–1096 CrossRef CAS
.
- M. N. Yousaf, B. T. Houseman and M. Mrksich, Proc. Natl. Acad. Sci. U. S. A., 2001, 98, 5992–5996 CrossRef CAS
.
- W. S. Yeo, M. N. Yousaf and M. Mrksich, J. Am. Chem. Soc., 2003, 125, 14994–14995 CrossRef CAS
.
- W. S. Dillmore, M. N. Yousaf and M. Mrksich, Langmuir, 2004, 20, 7223–7231 CrossRef CAS
.
- B. T. Houseman, J. H. Huh, S. J. Kron and M. Mrksich, Nat. Biotechnol., 2002, 20, 270–274 CrossRef CAS
.
- B. T. Houseman and M. Mrksich, Trends Biotechnol., 2002, 20, 279–281 CrossRef CAS
.
- G. Mihov, D. Grebel-Koehler, A. Lubbert, G. W. M. Vandermeulen, A. Herrmann, H. A. Klok and K. Mullen, Bioconjugate Chem., 2005, 16, 283–293 CrossRef CAS
.
- A. Graven and M. Meldal, J. Chem. Soc., Perkin Trans. 1, 2001, 3198–3203 RSC
.
- A. Doerr, Nat. Methods, 2006, 3, 77–77 Search PubMed
.
- Y.-W. Wu, H. Waldmann, R. Reents, F. H. Ebetino, R. S. Goody and K. Alexandrov, ChemBioChem, 2006, 7, 1859–1861 Search PubMed
.
- A. D. de Araújo, J. M. Palomo, J. Cramer, M. Köhn, H. Schröder, R. Wacker, C. Niemeyer, K. Alexandrov and H. Waldmann, Angew. Chem., 2006, 118, 302–307 Search PubMed
.
- S. S. van Berkel, A. T. J. Dirks, M. F. Debets, F. L. van Delft, J. J. L. M. Cornelissen, R. J. M. Nolte and F. P. J. T. Rutjes, ChemBioChem, 2007, 8, 1504–1508 CrossRef CAS
.
- V. Pozsgay, N. E. Vieira and A. Yergey, Org. Lett., 2002, 4, 3191–3194 Search PubMed
.
- J. M. Hooker, E. W. Kovacs and M. B. Francis, J. Am. Chem. Soc., 2004, 126, 3718–3719 CrossRef CAS
.
- U. T. T. Nguyen, J. Cramer, J. Gomis, R. Reents, M. Gutierrez-Rodriguez, R. S. Goody, K. Alexandrov and H. Waldmann, ChemBioChem, 2007, 8, 408–423 CrossRef CAS
.
- R. Rossin, P. Renart Verkerk, S. M. van den Bosch, R. C. M. Vulders, I. Verel, J. Lub and M. S. Robillard, Angew. Chem., Int. Ed., 2010, 49, 3375–3378 CAS
.
- S. S. van Berkel, A. J. Dirks, S. A. Meeuwissen, D. L. L. Pingen, O. C. Boerman, P. Laverman, F. L. van Delft, J. Cornelissen and F. Rutjes, ChemBioChem, 2008, 9, 1805–1815 CrossRef CAS
.
- P. Laverman, S. A. Meeuwissen, S. S. van Berkel, W. J. G. Oyen, F. L. van Delft, F. Rutjes and O. C. Boerman, Nucl. Med. Biol., 2009, 36, 749–757 CrossRef CAS
.
- M. Wiessler, W. Waldeck, C. Kliem, R. Pipkorn and K. Braun, Int. J. Med. Sci., 2010, 7, 19–28 Search PubMed
.
- R. Pipkorn, W. Waldeck, B. Didinger, M. Koch, G. Mueller, M. Wiessler and K. Braun, J. Pept. Sci., 2009, 15, 235–241 CrossRef CAS
.
- M. Wiessler, W. Waldeck, R. Pipkorn, C. Kliem, P. Lorenz, H. Fleischhacker, M. Hafner and K. Braun, Int. J. Med. Sci., 2010, 7, 213–223 Search PubMed
.
- J. Schoch, M. Wiessler and A. Jäschke, J. Am. Chem. Soc., 2010, 132, 8846–8847 CrossRef CAS
.
- N. K. Devaraj, S. Hilderbrand, R. Upadhyay, R. Mazitschek and R. Weissleder, Angew. Chem., Int. Ed., 2010, 49, 2869–2872 CAS
.
- B. T. Houseman and M. Mrksich, Chem. Biol., 2002, 9, 443–454 CrossRef CAS
.
- J. Zhu, M. D. Ganton, M. A. Kerr and M. S. Workentin, J. Am. Chem. Soc., 2007, 129, 4904–4905 Search PubMed
.
- N. Horan, L. Yan, H. Isobe, G. M. Whitesides and D. Kahne, Proc. Natl. Acad. Sci. U. S. A., 1999, 96, 11782–11786 CrossRef CAS
.
- A. Berkin, B. Coxon and V. Pozsgay, Chem.–Eur. J., 2002, 8, 4424–4433 CrossRef CAS
.
- S. J. Park and I. J. Shin, Angew. Chem., Int. Ed., 2002, 41, 3180–3182 CrossRef CAS
.
- A. Monzo and A. Guttman, QSAR Comb. Sci., 2006, 25, 1033–1038 Search PubMed
.
- K. R. Love and P. H. Seeberger, Angew. Chem., Int. Ed., 2002, 41, 3583–3586 CrossRef CAS
.
- X. L. Sun, C. L. Stabler, C. S. Cazalis and E. L. Chaikof, Bioconjugate Chem., 2006, 17, 52–57 CrossRef CAS
.
- M. Shi, J. H. Wosnick, K. Ho, A. Keating and M. S. Shoichet, Angew. Chem., Int. Ed., 2007, 46, 6126–6131 CrossRef CAS
.
- M. Shi and M. S. Shoichet, J. Biomater. Sci., Polym. Ed., 2008, 19, 1143–1157 CrossRef CAS
.
- M. Shi, K. Ho, A. Keating and M. S. Shoichet, Adv. Funct. Mater., 2009, 19, 1689–1696 CrossRef CAS
.
- M. Shi, J. Lu and M. S. Shoichet, J. Mater. Chem., 2009, 19, 5485–5498 RSC
.
- L. Nebhani and C. Barner-Kowollik, Adv. Mater., 2009, 21, 3442–3468 CrossRef CAS
.
- M. Vargas, R. M. Kriegel, D. M. Collard and D. A. Schiraldi, J. Polym. Sci., Part A: Polym. Chem., 2002, 40, 3256–3263 Search PubMed
.
- L. Nebhani, D. Schmiedl, L. Barner and C. Barner-Kowollik, Adv. Funct. Mater., 2010, 20, 2010–2020 Search PubMed
.
- X. J. Zhou, Q. Y. Li and C. F. Wu, Appl. Organomet. Chem., 2008, 22, 78–81 Search PubMed
.
- M. T. Beck, G. Mandy, S. Papp and I. Dekany, Colloid Polym. Sci., 2004, 283, 237–242 Search PubMed
.
- C. Menard-Moyon, F. Dumas, E. Doris and C. Mioskowski, J. Am. Chem. Soc., 2006, 128, 14764–14765 CrossRef CAS
.
- N. Salah, S. S. Habib, Z. H. Khan, S. Al-Hamedi and F. Djouider, Radiat. Phys. Chem., 2009, 78, 910–913 Search PubMed
.
- J. L. Delgado, P. de la Cruz, F. Langa, A. Urbina, J. Casado and J. T. L. Navarrete, Chem. Commun., 2004, 1734–1735 RSC
.
- A. Gergely, J. Telegdi, E. Meszaros, Z. Paszti, G. Tarkanyi, F. H. Karman and E. Kalman, J. Nanosci. Nanotechnol., 2007, 7, 2795–2807 Search PubMed
.
- F. Mercuri and A. Sgamellotti, Phys. Chem. Chem. Phys., 2009, 11, 563–567 RSC
.
- M. T. Beck, J. Szepvolgyi, P. Szabo and E. Jakab, Carbon, 2001, 39, 147–149 Search PubMed
.
- H. Hayden, Y. K. Gun'ko and T. S. Perova, Chem. Phys. Lett., 2007, 435, 84–89 Search PubMed
.
- L. Zhang, J. Z. Yang, C. L. Edwards, L. B. Alemany, V. N. Khabashesku and A. R. Barron, Chem. Commun., 2005, 3265–3267 RSC
.
- X. Lu, F. Tian, N. Q. Wang and Q. N. Zhang, Org. Lett., 2002, 4, 4313–4315 CrossRef CAS
.
- C.-M. Chang and Y.-L. Liu, Carbon, 2009, 47, 3041–3049 CrossRef CAS
.
- B. Xu, X. S. Wang and Y. Lu, Appl. Surf. Sci., 2006, 253, 2695–2701 Search PubMed
.
- F. M. Fernandes, R. Araujo, M. F. Proenca, C. J. R. Silva and M. C. Paiva, J. Nanosci. Nanotechnol., 2007, 7, 3514–3518 Search PubMed
.
- M. F. Proenca, R. F. Araujo, M. C. Paiva and C. J. R. Silva, J. Nanosci. Nanotechnol., 2009, 9, 6234–6238 Search PubMed
.
- M. C. Paiva, R. M. Novais, R. F. Araujo, K. K. Pederson, M. F. Proença, C. J. R. Silva, C. M. Costa and S. Lanceros-Méndez, Polym. Compos., 2010, 31, 369–376 Search PubMed
.
- S. Munirasu, J. Albuerne, A. Boschetti-de-Fierro and V. Abetz, Macromol. Rapid Commun., 2010, 31, 574–579 Search PubMed
.
- K. I. Guhr, M. D. Greaves and V. M. Rotello, J. Am. Chem. Soc., 1994, 116, 5997–5998 CrossRef CAS
.
- J. Y. Yao, Z. Xiao, J. X. Zhang, X. B. Yang, L. B. Gan and W. X. Zhang, Chem. Commun., 2009, 401–403 RSC
.
- A. Kraus and K. Mullen, Macromolecules, 1999, 32, 4214–4219 CrossRef CAS
.
- L. Nebhani and C. Barner-Kowollik, Macromol. Rapid Commun., 2010, 31, 1298–1305 CrossRef CAS
.
- Y. Vida, R. Suau, J. Casado, A. Berlin, J. T. L. Navarrete and E. Pérez-Inestrosa, Macromol. Rapid Commun., 2007, 28, 1345–1349 CrossRef CAS
.
- L. Nebhani, P. Gerstel, P. Atanasova, M. Bruns and C. Barner-Kowollik, J. Polym. Sci., Part A: Polym. Chem., 2009, 47, 7090–7095 CrossRef
.
- C. Wendeln, A. Heile, H. F. Arlinghaus and B. J. Ravoo, Langmuir, 2010, 26, 4933–4940 CrossRef CAS
.
- X. F. Liu, M. Zhu, S. H. Chen, M. J. Yuan, Y. B. Guo, Y. L. Song, H. B. Liu and Y. L. Li, Langmuir, 2008, 24, 11967–11974 CrossRef CAS
.
- X. F. Liu, H. B. Liu, W. D. Zhou, H. Y. Zheng, X. D. Yin, Y. L. Li, Y. B. Guo, M. Zhu, C. B. Ouyang, D. B. Zhu and A. D. Xia, Langmuir, 2010, 26, 3179–3185 CrossRef CAS
.
- J. Zhu, A. J. Kell and M. S. Workentin, Org. Lett., 2006, 8, 4993–4996 CrossRef CAS
.
- K. Adachi, A. K. Achimuthu and Y. Chujo, Macromolecules, 2004, 37, 9793–9797 Search PubMed
.
|
This journal is © The Royal Society of Chemistry 2011 |