DOI:
10.1039/C0PY00387E
(Paper)
Polym. Chem., 2011,
2, 868-872
Charge transport properties of polymer films comprising oligothiophene in silsesquioxane network
Received
25th November 2010
, Accepted 13th December 2010
First published on 20th January 2011
Abstract
Transparent and conductive films were grafted covalently on glass and ITO substrates with polysilsesquioxane having octithiophene, and their charge transport properties, were studied by electrochemical means. The cyclic voltammogram of oligothiophene fixed in silsesquioxane network was found to be broad because the conformations of oligothiophenes are restricted by the rigid polymer network. In situ electrical conductivities of the polymer films were measured simultaneously with doping levels at different potentials to yield charge carrier mobilities as a function of doping level of the polymer.
Introduction
Oligothiophenes have been studied as model compounds of polythiophenes and optical, electrochemical, and electrical properties of conducting polymers have been clarified through intensive studies on oligothiophenes.1 Because of their properties superior to those of polythiophenes due to the well-defined structures, oligothiophenes themselves have been applied to the photo- and electroactive materials such as field-effect transistors, electroluminescent and electrochromic displays, photovoltaic cells, and non-linear optics.2 To extend the field of their applications, oligothiophenes introduced into the inert non-conjugated polymer chain, for example, polyethylenes having pendant oligothiophenes3 and linear polymers including oligothienylene bridged by alkylenes or silylenes,4 have been developed.
As inert polymer backbones, polysilsesquioxanes are of a great interest because they can be readily synthesized by the sol–gel method with the corresponding trialkoxysilane monomers to give a three-dimensional network polymer having a thermally stable siloxane bond.5 Also, silanol groups remaining at the end of polymer chain can react with a metal-hydroxide group. By utilizing the reactivity of polysilsesquioxanes, one can fix oligothiophenes on the surface of metal–oxide substrates such as glass (SiO2), ITO (In2O3–SnO2) and titania (TiO2), if oligothiophenes are chemically bound to polysilsesquioxanes. In order to realize our concept, we have recently synthesized octithiophene (thiophene octamer) introduced into a polysilsesquioxane network (Fig. 1a) and found that the mechanical property of the polymer film fixed on glass and ITO substrates was much superior to those of polymers with no chemical linkage between polymers and substrates.6 We also reported the electrical conductivity of the polymer film doped with FeCl3, although the chemical oxidation was unable to control the doping level and thus the detailed discussion on the transport properties, especially the effect of the chemical structure of the polymer backbone, has not been carried out yet. We have developed an electrochemical technique to evaluate charge carrier mobilities as a function of doping level,7 and applied this technique to linear polymers, in which oligothiophenes (pentamer to tetradecamer) are connected with silylene unit (an example is shown in Fig. 1b), in order to clarify their charge-transport properties.4c,d
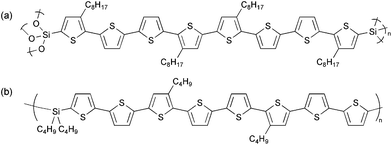 |
| Fig. 1 Chemical structures of (a) polysilsesquioxane (PBS8T) and (b) silylene-bridged linear polymer (LP8T) having octithiophene. | |
In this report, we describe charge-transport properties of polymer films consisting of polysilsesquioxane having octithiophene with a special attention on the difference between silylene-bridged linear polymers and the silsesquioxane network polymer.
Experimental
Instrumentation and materials
Polymerization process and molecular weight of the resulting polymer were analyzed by a gel-permeation chromatography (GPC) coupled with a UV detector. Polystyrene standards in THF were used as references for GPC. IR spectra were recorded on a Perkin Elmer Spectrum One FT-IR spectrophotometer by ATR method. UV-Vis absorption spectra were measured by a Shimadzu UV-3150 spectrophotometer. Cyclic voltammetry and in situ conductivity measurements were carried out using a potentiostat/galvanostat (Hokuto Denko, HAB-151) with an X–Y recorder (Riken Denshi, F-57), a coulometer (homemade), and a function generator (Iwatsu, SG-4105). n-Hexane, toluene, tetrahydrofuran (THF), dichloromethane, and acetonitrile were purified by ordinary methods and used immediately after purification. Tetraethylammonium perchlorate (TEAP) purchased from Tokyo Chemical Industry was purified by recrystallization from ethanol and dried. n-Butyllithium (n-BuLi) in n-hexane was purchased from Kanto Chemicals. 3,3′′′-Dioctyl-2,2′:5′,2′′:5′′,2′′′-quaterthiophene (4T) was synthesized according to the literature.8
3,3′′′,4′′′′,3′′′′′′′-Tetraoctyl-2,2′:5′,2′′:5′′,2′′′:5′′′,2′′′′:5′′′′,2′′′′′:5′′′′′,2′′′′′′:5′′′′′′,2′′′′′′′-octithiophene (8T)
To a 50 mL THF solution of 4T (4.9 g, 8.8 mmol), n-BuLi (1.6 M n-hexane solution, 6 mL, 9.6 mmol) was added dropwise at −78 °C. The reaction mixture was stirred at −78 °C for 30 min and kept at room temperature for 1 h. CuCl2 (1.5 g, 11 mmol) was then added to the solution. The reaction mixture was stirred at room temperature for 24 h, then poured into water (100 mL), and extracted three times with dichloromethane (100 mL each). The combined organic extracts were washed with NH4Cl aq (2 × 100 mL) and dried over Na2SO4. The solvent was removed by a rotary evaporation. 8T (0.65 g, 0.6 mmol, 13%) was obtained as a red solid after a column chromatography (SiO2, n-hexane/dichloromethane = 19/1 (v/v), then n-hexane). 1H NMR (CD2Cl2, 270 MHz) δ ppm: 0.88 (t, J = 7.6 Hz, 12H, CH3), 1.2–1.5 (broad, 40H, CH2), 1.67 (tt, J = 7.6, 7.6 Hz, 8H, thienyl-CH2CH2), 2.79 (t, J = 7.6 Hz, 8H, thienyl-CH2), 6.97 (d, J = 5.5 Hz, 2H, thienyl-H), 7.05 (d, J = 3.8 Hz, 2H, thienyl-H), 7.06 (s, 2H, thienyl-H), 7.08 (d, J = 3.8 Hz, 2H, thienyl-H), 7.17 (d, J = 3.8 Hz, 4H, thienyl-H), 7.21 (d, J = 5.5 Hz, 2H, thienyl-H). FT-IR (ATR)
cm−1: 3062, 2953, 2920, 2850, 1460, 785. FAB-MS 1106.3 (M+).
3,3′′′,4′′′′,3′′′′′′′-Tetraoctyl-5,5′′′′′′′-bis(triethoxysilyl)-2,2′:5′,2′′:5′′,2′′′:5′′′,2′′′′:5′′′′,2′′′′′:5′′′′′,2′′′′′′:5′′′′′′,2′′′′′′′-octithiophene (BS8T)
To a 50 mL THF solution of 8T (62 mg, 56 μmol), n-BuLi (2.7 M n-hexane solution, 0.1 mL, 0.3 mmol) was added dropwise at −78 °C. The reaction mixture was stirred at −78 °C for 30 min and an excess amount of (EtO)3SiCl (370 mg, 1.8 mmol) was then added to the solution. The reaction mixture was stirred at −78 °C for 1 h and kept at room temperature for 3 h. The solvent and remaining (EtO)3SiCl were removed at 100 °C for 3 h in vacuo. Polymeric and/or oligomeric compounds in the residue were removed by a preparative GPC. Finally, BS8T (50 mg, 35 μmol, 63%) was obtained as a red solid. 1H NMR (CD2Cl2, 270 MHz) δ ppm: 0.88 (t, J = 7.7 Hz, 6H, CH3), 0.90 (t, J = 7.7 Hz, 6H, CH3), 1.26 (t, J = 6.8 Hz, 18H, SiOCH2CH3), 1.2–1.5 (broad, 40H, CH2), 1.67 (tt, J = 7.6, 7.6 Hz, 4H, thienyl-CH2CH2), 1.68 (tt, J = 7.6, 7.6 Hz, 4H, thienyl-CH2CH2), 2.79 (t, J = 7.6 Hz, 4H, thienyl-CH2), 2.81 (t, J = 7.6 Hz, 4H, thienyl-CH2), 3.89 (q, J = 6.8 Hz, 12H, SiOCH2CH3), 7.06 (s, 2H, thienyl-H), 7.09 (d, J = 3.9 Hz, 2H, thienyl-H), 7.11 (d, J = 3.9 Hz, 2H, thienyl-H), 7.18 (d, J = 3.9 Hz, 4H, thienyl-H), 7.26 (s, 2H, thienyl-H). FT-IR (ATR)
cm−1: 3065, 2960, 2926, 2855, 1463, 1261, 1167, 1102, 1082, 1024, 793. FAB-MS 1431.5 (M+).
Polymerization of BS8T and anchoring of PBS8T
Polymerization of BS8T was carried out in THF at room temperature for 6 h using dilute hydrochloric acid (0.1 M) as a polymerization catalyst and the reaction process was monitored by GPC (Scheme 1). A solution containing the resulting polymer (PBS8T) (Mn ≈ 2500, estimated by GPC using polystyrene standard) was directly spin-coated on glass or ITO substrate (3000 rpm for 20 s and then 5000 rpm for 10 s). The spun film was cured at 100 °C for 30 min and sonicated in acetone to remove the unreacted BS8T. The resulting anchored film on a substrate is named ancanc-PBS8T. The thicknesses of ancanc-PBS8T films were estimated to be ca. 20–80 nm by an atomic force microscopy (Agilent Technologies, 5500 Scanning Probe Microscope). The FT-IR spectrum of PBS8T showed a broad and strong peak at 1000–1200 cm−1 due to the Si–O–Si stretching vibration, which was not observed in the spectrum of BS8T. This result suggests the formation of siloxane-bond network by the polymerization.
In situ conductivity measurement
In situ conductivity measurements were carried out in TEAP (0.1 M)/acetonitrile by a two-probe method7 with an ancanc-PBS8T film coated on a micro-array Pt electrode (ALS Co., Ltd., 65 lines, separation distance = 5 μm, total width = 260 mm). The amounts of charges generated by electrochemical oxidation (doping) and reduction (dedoping) of the polymer film were measured with a coulometer by stepping a potential from −0.1 V to a desired potential and back to −0.1 V, respectively. Doping levels, defined as the number of charges per thiophene ring, were estimated from the doping/dedoping charges, a weight of an ancanc-PBS8T film and a molecular weight of the monomer unit, (8T)(SiO1.5)2. Apparent mobilities (μ) of charge carriers in ancanc-PBS8T at various doping levels (electrode potentials) were calculated from the following relation:
where σ, n, and e denote the electrical conductivity at an electrode potential, the number of charge carriers estimated from the doping/dedoping charges, and the elementary electric charge, respectively.
Results and discussion
Polycondensation process of BS8T monitored by GPC is shown in Fig. 2. When dilute hydrochloric acid (0.1 M) as a polymerization catalyst was added into the THF solution of BS8T, the molecular weight of the formed polymer started to increase soon after the addition of the acid catalyst and leveled off at 6 h, although the reaction solution remained homogenous. However, once the solvent was removed from the polymer solution, a reddish orange solid which was insoluble in common organic solvents was obtained. This finding suggests that the PBS8T in THF still has reactive sites which can react partly with hydroxyl groups on the surface of glass or ITO substrate to form an insoluble polymer (ancanc-PBS8T) film fixed covalently on the substrate. The insoluble nature of ancanc-PBS8T film is of a great use for the fabrication of multi-layered molecular devices by a wet process.
Electrochemical properties
Cyclic voltammetry (CV) of an ancanc-PBS8T film grafted on ITO was investigated using Pt wire and Ag/Ag+ as a counter and reference electrodes, respectively (Fig. 3). Two oxidation peaks were observed at 0.6 and 0.8 V, corresponding to the first and second oxidation steps of octithienylene moiety in ancanc-PBS8T, respectively. Even after the potential was cycled between 0.5 and 0.9 V over 300 times, the shape of the CV curve was similar to that in the first cycle. In the case of a silylene-bridged 8T-containing linear polymer4c (LP8T, Fig. 1b), it is known that the CV curve of the LP8T film shows only one sharp peak at 0.5 V, compared with the broad CV curve for the ancanc-PBS8T film. A possible reason for the difference in shape of the CV curves is a distribution in conformation of oligothienylene units in the polymer chains, which is discussed in the later part of this report. Corriu et al. have synthesized polysilsesquioxanes having short oligothiophenes (monomer to trimer), and studied their electrochemical properties.9 When these polymers were chemically or electrochemically oxidized, oligothiophenes were eliminated and polymerized because the oxidized state of short oligothiophenes is not stable. In contrast to their results, the ancanc-PBS8T film exhibited a good electrochemical stability due to the delocalization of the formed charge over the longer π-conjugation unit, and a good adhesivity of the polymer to the ITO substrate.
Spectroelectrochemical properties
To identify the redox states of ancanc-PBS8T, spectroelectrochemistry of the ancanc-PBS8T film on ITO was investigated (Fig. 4). As shown in the inset of Fig. 4, the neutral ancanc-PBS8T film showed a single absorption band at 450 nm due to π–π* transition of a neutral octithienylene moiety. When the ancanc-PBS8T film was oxidized at 0.6 V, the intensity of the 450 nm band decreased, and instead two absorption bands appeared at 750 and 1200 nm, which were ascribed to the one-electron oxidized species (monocation radical or π-dimer) of octithienylene unit. The ancanc-PBS8T film oxidized at 0.9 V showed a single broad band at 950 nm, being ascribed to the two-electron oxidized species (dication) of octithienylene unit, and became almost colorless. These spectroelectrochemical changes are similar to those of LP8T, suggesting that the spectroscopic properties are not affected by the polymer structure.
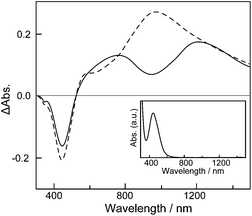 |
| Fig. 4 Difference absorption spectra of the ancanc-PBS8T film on ITO biased at 0.6 V (solid) and 0.9 V (dashed), referred to the spectrum of the neutral ancanc-PBS8T film (inset). | |
Electrical properties
In our previous communication, the electrical conductivity of an ancanc-PBS8T film oxidized with FeCl3 was reported to be around 10−3 S cm−1,6a where the extent of oxidation of the polymer film (doping level) was not clarified. In order to investigate the electrical properties of the ancanc-PBS8T film in more detail, doping levels and electrical conductivities were measured in the potential range between 0.3 and 0.85 V by using an in situ electrochemical technique.7Fig. 5 depicts semilogarithmic plots of doping level and conductivity against potential for the ancanc-PBS8T film. In concert with the electrochemical oxidation of the ancanc-PBS8T film (Fig. 3), the doping level gradually increased and finally reached around 25% at 0.9 V, which corresponds to 200% doping per octithienylene unit and suggests that all octithienylene units are completely two-electron oxidized. The plot of log (doping level) vs. potential fits a straight line in a low doping region and its slope value is around 100 mV per decade, which is larger than 60 mV per decade for a common one-electron transfer process at room temperature. We have already clarified that the slope value is a measure of distribution of effective π-conjugation length in conjugated oligomers and polymers, and wider distribution leads to a larger slope value.7c,10 In the ancanc-PBS8T film, since both terminal thiophene rings of octithienylene unit are tightly fixed with silsesquioxane network, their conformation is partially restricted not to spread the π-conjugation all over the oligothienylene unit. Thus, the ancanc-PBS8T film may include octithienylene units with different effective π-conjugation lengths, so that the slope of log (doping level) vs. potential is larger than that for the LP8T film. This may also explain the broad CV curve of the ancanc-PBS8T film on ITO (Fig. 3).
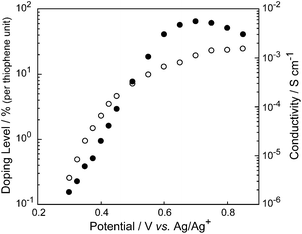 |
| Fig. 5
In situ electrical conductivity (solid circle) and doping level (open circle) of the ancanc-PBS8T film plotted against electrode potential. | |
The electrical conductivity also increased with increasing the electrode potentials from 0.3 to 0.7 V, showed a maximum value of 5.6 × 10−3 S cm−1, and then decreased to 3.0 × 10−3 S cm−1 at 0.9 V (Fig. 5). This trend is similar to those of silylene-bridged linear polymers.4c,d We reported that the electrical conductivities of silylene-bridged linear polymers are affected by the difference in distribution of chain length of the polymer, and that the conductivities of LP8Ts with Mw/Mn = 1.68 and 5.67 are 1.0 × 10−1 and 5.0 × 10−3 S cm−1, respectively. The conductivity of ancanc-PBS8T is close to that of LP8T with larger distribution of chain length. This fact may be caused by the disordered structure of oligothienylene unit in the ancanc-PBS8T film.
To further discuss the charge transport properties, the apparent mobilities of charge carriers were calculated by the equation μ = σ/(ne) and the relationship between the apparent charge carrier mobilities and the doping levels was plotted (Fig. 6). At low doping levels below 1%, charge carrier mobilities for ancanc-PBS8T are almost constant at 1 × 10−6 cm2 V−1s−1, which is similar to those of polythiophenes and silylene-bridged linear polymers having oligothiophenes including LP8T.4c,d,7 In the case of silylene-bridged polymers, the mobility at lower doping level was explained as the interchain hopping transport mechanism of localized monocation radical (polaron) species.4c,d Since each octithienylene unit in ancanc-PBS8T is also isolated in the inert silsesquioxane network, it is reasonable to consider that a similar hopping transport mechanism is available. The charge carrier mobility showed a maximum value of 4.8 × 10−5 cm2 V−1s−1 at the doping level of 15% (120% doping per octithienylene unit), in which one- and two-electron oxidized states coexist. At the doping of 25% (200% doping per octithiophene), all octithienylene units were two-electron oxidized, so that it is difficult for the positive charge to move among oligothienylene units because of a Coulombic repulsion. The charge carrier mobilities of ancanc-PBS8T are found to be by two orders of magnitude lower than those of LP8T with smaller distribution of chain length (Mw/Mn = 1.68). These results suggest that the charge transport properties of oligothiophenes in polymers will be strongly affected by their environments such as the rigidity of polymer network and the distribution of polymer chains. However, this is not an essential problem, because the rigidity of polymer network and the distribution of polymer chains can be tuned by polymerization conditions. Thus, it is expected that the electrical properties of polysilsesquioxanes having oligothiophenes will be improved by the optimization of the rigidity of polymer network.
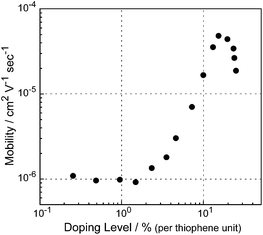 |
| Fig. 6 Apparent charge carrier mobilities of the ancanc-PBS8T film plotted against doping level. | |
We also found that the maximum values of charge carrier mobility of silylene-bridged linear polymers having oligothiophenes (thiophene pentamer to tetradecamer) increased with increasing the conjugation length of oligothienylene unit, and that the polymers having dodecamer and tetradecamer showed mobilities similar to those of polythiophenes. Thus, polysilsesquioxanes having longer oligothiophenes will become excellent candidates of conductive materials suitable for the fabrication of multi-layered molecular devices by a wet process.
Conclusions
A polysilsesquioxane having an octithiophene was synthesized by polycondensation reaction of a corresponding monomer, a bis(triethoxysilyl)-substituted octithiophene. The resulting polymer was obtained as homogenous solution, but became insoluble due to the post-polymerization once it was spin-coated and annealed. Cyclic voltammogram of the polymer film on ITO was broad because of the conformational distribution of oligothienylene unit due to the rigid polymer network. In situ measurement of electrical conductivity suggested that the charge transport properties are strongly affected by the environment of oligothiophenes in polymers. Silsesquioxane network can give an advantage to produce the printable electronic devices by optimizing the rigidity of polymer network.
Acknowledgements
This work was supported in part by a grant-in-aid for scientific research from the Japan Society for the Promotion of Science (JSPS) (no. 22550198, I.I. and no. 19350094, Y.H.). The authors are grateful to Dr Daisuke Kajiya, the Natural Science Center for Basic Research and Development (N-BARD), Hiroshima University for the measurement of FAB-MS.
References
- For example: P. Bäuerle, Adv. Mater., 1992, 4, 102 Search PubMed.
-
(a)
D. Fichou, Handbook of Oligo- and Polythiophenes, Wiley-VCH, Weinheim, 1999 Search PubMed;
(b)
P. Bäuerle, Oligothiophenes, in Electronic Materials: the Oligomer Approach, ed. K. Müllen and G. Wegner, Wiley-VCH, Weinheim, 1998, ch. 2, section 1 Search PubMed;
(c) A. Mishra, C.-Q. Ma and P. Bäuerle, Chem. Rev., 2009, 109, 1141 CrossRef CAS.
-
(a) K. Nawa, K. Miyawaki, I. Imae, N. Noma and Y. Shirota, J. Mater. Chem., 1993, 3, 113 RSC;
(b) I. Imae, K. Nawa, Y. Ohsedo, N. Noma and Y. Shirota, Macromolecules, 1997, 30, 380 CrossRef CAS;
(c) Y. Ohsedo, I. Imae and Y. Shirota, J. Polym. Sci., Part B: Polym. Phys., 2003, 41, 2471 CrossRef CAS.
-
(a) X. Jiang, Y. Harima, L. Zhu, Y. Kunugi, K. Yamashita, M. Sakamoto and M. Sato, J. Mater. Chem., 2001, 11, 3043 RSC;
(b) X. Jiang, Y. Harima, K. Yamashita, A. Naka, K. K. Lee and M. Ishikawa, J. Mater. Chem., 2003, 13, 785 RSC;
(c) Y. Harima, X. Jiang, Y. Kunugi, K. Yamashita, A. Naka, K. K. Lee and M. Ishikawa, J. Mater. Chem., 2003, 13, 1298 RSC;
(d) Y. Harima, D.-H. Kim, Y. Tsutitori, X. Jiang, R. Patil, Y. Ooyama, J. Ohshita and A. Kunai, Chem. Phys. Lett., 2006, 420, 387 CrossRef CAS.
- R. H. Baney, M. Itoh, A. Sakakibara and T. Suzuki, Chem. Rev., 1995, 95, 1409 CrossRef CAS.
-
(a) I. Imae, S. Takayama, D. Tokita, Y. Ooyama, K. Komaguchi, J. Ohshita, T. Sugioka, K. Kanehira and Y. Harima, Polymer, 2009, 50, 6198 CrossRef CAS;
(b)
Y. Harima, J. Ohshita, I. Imae, T. Sugioka and K. Kanehira, Jpn. Kokai Tokkyo Koho, 2010-266727 Search PubMed.
-
(a) Y. Harima, T. Eguchi and K. Yamashita, Synth. Met., 1998, 95, 69 CrossRef CAS;
(b) Y. Harima, T. Eguchi, K. Yamashita, K. Kojima and M. Shiotani, Synth. Met., 1999, 105, 121 CrossRef CAS;
(c) Y. Harima, Y. Kunugi, K. Yamashita and M. Shiotani, Chem. Phys. Lett., 2000, 317, 310 CrossRef CAS;
(d) H. Tang, L. Zhu, Y. Harima and K. Yamashita, Synth. Met., 2000, 110, 105 CrossRef CAS.
- P. Bäuerle, T. Fischer, B. Bidlingmeier, A. Stabel and J. P. Rabe, Angew. Chem., Int. Ed. Engl., 1995, 34, 303 CrossRef.
- R. J. P. Corriu, J. J. E. Moreau, P. Thepot and M. W. C. Man, Chem. Mater., 1994, 6, 640 CrossRef CAS.
-
(a) J. Yano, M. Kobayashi, S. Yamasaki, Y. Harima and K. Yamashita, Synth. Met., 2001, 119, 315 CrossRef CAS;
(b) Y. Harima, T. Eguchi and K. Yamashita, Synth. Met., 1998, 95, 69 CrossRef CAS.
|
This journal is © The Royal Society of Chemistry 2011 |