DOI:
10.1039/C0PY00364F
(Paper)
Polym. Chem., 2011,
2, 862-867
Extraction of proteins with temperature sensitive and reversible phase change of ionic liquid/water mixture
Received
5th November 2010
, Accepted 13th December 2010
First published on 20th January 2011
Abstract
Tetrabutylphosphonium N-trifluoromethanesulfonyl leucine ([P4444][Tf-Leu], IL) shows phase separation with water at 25 °C, but is miscible at 20 °C. Such a reversible phase transition, called lower critical solution temperature (LCST) behaviour, has been utilised to extract proteins. An aqueous solution of cytochrome c (Cyt. c) was mixed with an equal volume of the IL and the solution was cooled down to 20 °C to obtain a homogeneous solution. After phase separation was induced by heating it at 25 °C, all the Cyt. c was found in the IL phase. The IL, containing at least 21 wt% of water, was effective to extract Cyt. c. The distribution ratio (D) of several proteins in the IL/water mixture depended strongly on the isoelectric point (pI) of the corresponding proteins. Based on the difference in the D value, separation of a target protein from their mixtures was successfully carried out using the LCST behaviour of the IL/water mixture.
Introduction
While it is generally accepted that water is the best solvent for biomolecules especially biopolymers, a water-based system has a few drawbacks for the practical use of those biopolymers. For example, both volatility and narrow temperature range to keep protein activity are inconvenient. A tiny volume of protein aqueous solution would lead to deactivation or denaturation of proteins because of rapid water vaporisation. Against this, ionic liquids (ILs) have excellent physicochemical properties such as negligible vapor pressure and high thermal stability.1–4 There are increasing studies on the biological reactions in ILs in spite of the simple belief that the aqueous phase is the best medium for biological systems. A number of biopolymers such as proteins have been introduced into ILs and their activity has been studied.5–7 There are two major approaches to apply ILs as solvents for proteins. One is the use of pure IL. Some reports suggested that pure ILs display useful properties including an enhancement of catalytic activity8,9 and thermal stability of proteins.10 In pure ILs, most proteins were dispersed but not homogeneously dissolved. Pure ILs have almost no ability to solubilise proteins without denaturation.11,12 The other is the ILs containing a small amount of water.13 They are classified as “hydrated ILs”. A few groups have reported an extraordinary stability of proteins in the hydrated ILs that cannot be realised in an aqueous solution.14–17
ILs have wide structural diversity and it is easy to modify their physicochemical properties by changing their ionic structure.18 We have reported some ILs prepared from amino acids.19–21 Since amino acids contain not only an amino group and carboxylic acid residue but also various side groups in a single molecule, amino acids should be excellent starting materials for preparing functionalised ILs.22 ILs composed of amino acids having a trifluoromethanesulfonyl group on the amino group exhibited unique phase behaviour with water.23 Upon heating the homogeneously mixed solution was phase separated into two phases, and it became miscible again upon cooling. Such reversible phase behaviour, with a lower critical solution temperature (LCST) of the IL/water mixture, had not previously been reported. Fig. 1 schematically shows the LCST-type response of an IL/water mixture. When tetrabutylphosphonium N-trifluoromethanesulfonyl leucine (IL1) was mixed with an equal amount of water (50 wt%), phase separation started at 22 °C. A clear phase separation was found after storing without any disturbance at 25 °C for 10 min. Since the phase can be controlled by changing the temperature for a few degrees, the LCST-type response of IL/water mixture will be used in various applications, especially in extraction of biopolymers such as proteins.
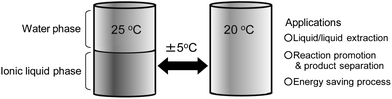 |
| Fig. 1 Schematic diagram of LCST-type response of IL/water mixture. | |
Experimental
Materials
L-Leucine was purchased from Wako Chem. Co. Trifluoromethanesulfonic anhydride and tributyloctylphosphonium bromide were purchased from Tokyo Chem. Ind. Co. Tetrabutylphosphonium hydroxide was a gift from Hokko Chem. Co. Cytochrome c from horse heart, myoglobin (Mb) from horse heart, hemoglobin (Hb) from bovine blood, lysozymefrom chicken egg white, and albumin from bovine serum were purchased from Sigma Aldrich. Laccasefrom Trametes versicolor and α-chymotrypsin from bovine pancreas were purchased from Fluka and Wako Chem. Co., respectively. Horseradish peroxidase (HRP) was donated from Toyobo Co. All proteins were used without further purification.
Synthesis of tetrabutylphosphonium N-trifluoromethanesulfonyl leucine (IL1) and tributyloctylphosphonium N-trifluoromethanesulfonyl leucine (IL2)
Both IL1 and IL2 (Fig. 2) were synthesised and purified using the procedure reported previously.23N-Trifluoromethanesulfonyl leucine methyl ester was synthesised by a reaction of trifluoromethanesulfonic anhydride with leucine methyl ester. The leucine methyl ester was suspended in dry dichloromethane, and bimolar triethylamine was then added under gentle stirring. A solution of trifluoromethanesulfonic anhydride was added to the mixture under dry N2 atmosphere at −78 °C. The resulting product was extracted to the diethyl ether and purified on silica gel (MeOH/CHCl3 = 1
:
5) to provide N-trifluoromethanesulfonyl leucine methyl ester. To hydrolyse the methyl ester group, the prepared leucine derivative was treated with 1.0 N NaOH solution at 0 °C for 10 h, and the sodium ions were removed by passing through a column filled with proton-exchange resign (Amberlite IRN 77). The product was washed with hexane to give N-trifluoromethanesulfonyl leucine. As a counter cation to N-trifluoromethanesulfonyl leucine, tetrabutylphosphonium cation ([P4444]), which provided a hydrophobic amino acid ILs,21 was introduced to prepare IL1. A tributyloctylphosphonium cation ([P4448]) was used to make more hydrophobic IL (i.e., IL2). In the case of [P4448], an aqueous solution of [P4448][OH] was prepared by passing an aqueous solution of [P4448][Br] through a column filled with the anion-exchange resin (Amberlite IRN 78). These phosphonium hydroxide solutions were mixed with a slightly less amount of trifluoromethanesulfonyl leucine, and the ILs were extracted with chloroform. After evaporation, the products were dried in vacuo for at least 24 h at 80 °C. The structure of ILs was confirmed by 1H NMR. IL1: 1H NMR (400 MHz, CDCl3, δ/ppm relative to TMS): 0.94 (m, J = 44.9 Hz, 18H, CH2CH3), 1.39 (m, J = 185.1 Hz, 18H, CH2CH3), 1.88 (m, J = 27.0 Hz, 1H, CHCH3), 2.26 (m, J = 29.3 Hz, 8H, PCH2), 3.67 (q, J = 12.8 Hz, 1H, NCH). IL2: 1H NMR (500 MHz, CDCl3, δ/ppm relative to TMS): 0.93 (m, J = 58.4 Hz, 18H, CH2CH3), 1.29 (m, J = 15.5 Hz, 8H, CH2CH3), 1.56 (m, J = 51.0 Hz, 16H, CH2CH3), 1.69 (m, J = 26.3 Hz, 2H, CH2CH), 1.92 (m, J = 20.1 Hz, 1H, CHCH3), 2.32 (m, J = 39.0 Hz, 8H, PCH2), 3.89 (t, J = 12.6 Hz, 1H, NCH).
Determination of distribution ratio (D)
Pure water and IL1 were mixed (50/50 wt%) and heated to 35 °C to induce phase separation. The phase separated IL1 was confirmed to have 30 wt% of water by Karl Fischer titration method (using a Kyoto Electronics MKC-510N). Then, thus 1.0 ml of phase-separated IL1 was pipetted out and mixed with 1.0 ml of protein aqueous solution (1.0 mg ml−1). The resulting IL1/water solution was phase-separated at room temperature, but it formed a single phase instantly under stirring and cooling in a water bath. This homogeneous solution was then heated to 25 °C and left to stand until the separated water phase became clear. The phase behaviour of the IL1/water mixture was confirmed by naked eye, and the temperature was controlled using a water bath attached with a digital thermometer (Fine Thermo F002DN). The distribution ratio (D) was calculated from the absorbance of proteins in the water phase, using the equation; D = (Absw-before − Absw-after)/Absw-before, where Absw-before and Absw-after corresponded to the absorbance of aqueous phase before and after phase separation, respectively. For heme proteins, the maximum absorption of the Soret band around 408.0 nm was used for Cyt. c, Mb, Hb, and HRP, and the absorbance at 280 nm was used for the other proteins. The absorbance of proteins was determined with a UV-vis spectrometer (Shimadzu UV-2450) at 25 °C. Resonance Raman spectra of Cyt. c in an aqueous solution and in the IL1 phase were obtained by a Jasco NRS-1000 spectrometer with a Kaiser Optical holographic notch-plus filter and a liquid N2-cooled charge-coupled device (CCD) detector. The excitation source was a Coherent Innova 90C Kr laser with a 20 mW beam at a 413.1 nm excitation wavelength. The peak frequencies were calibrated relative to an indene standard.
Results and discussion
Extraction of Cyt. c from aqueous phase to IL1 phase
First, an aqueous solution (pH 7.0) of cytochrome c (Cyt. c) was added to IL1 at 25 °C (Fig. 3a). Once the solution had been cooled to 20 °C, the two separated phases immediately became a homogeneous solution (Fig. 3b). Phase separation was then induced by gentle heating to 25 °C and left it to stand until the clear phase was obtained. Cyt. c moved into the IL1 (bottom) phase from the aqueous (upper) phase (Fig. 3c). According to UV-vis spectroscopy, less than 0.1% of the Cyt. c remained in the aqueous phase after phase separation; this value was smaller than the lower detection limit. This process was irreversible, and the Cyt. c remained in the IL phase even after repeating the phase separation process again.
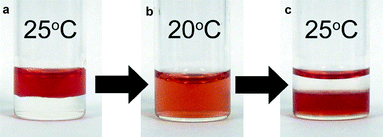 |
| Fig. 3
Extraction of Cyt. c from aqueous to IL1 phase by changing the temperature by 5 °C: (a) an aqueous solution of Cyt. c was added to IL1 at 25 °C; (b) the IL/aqueous solution at 20 °C; (c) the solution was heated again to 25 °C to separate both phases. The red colour at the top of the phase is merely a reflection of the IL/water interface. | |
Spectroscopic characterisation of Cyt. c in IL1 phase
We next spectroscopically confirmed the structure of the Cyt. c extracted in the IL1 phase. Heme proteins generally show the Soret band with λmax = 408 nm deriving from the π–π* transition of the porphyrin ring, which is a good indicator of the chemical environment around heme. In the case of Cyt. c extracted in the IL phase, the Soret band was observed at 407.2 nm (Fig. 4a(3)), which is shifted slightly from 408.0 nm observed in the aqueous phase (Fig. 4a(1)). The difference in the maximum absorption wavelengths should be due to the different polarities of the solvents rather than denaturation of the Cyt. c. For the aqueous phase after phase separation, no definitive absorbance from Cyt. c was observed. Accordingly, the Cyt. c can be considered to be fully extracted in the IL phase (Fig. 4a(2)). The extracted Cyt. c in the IL phase was confirmed to have almost exactly the same structure as that in aqueous solution.
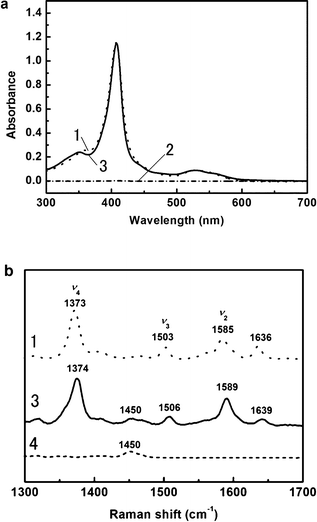 |
| Fig. 4 (a) UV-vis absorption spectra of Cyt. c in aqueous solution (dotted line, 1), in the IL1 phase (solid line, 3), and in the aqueous phase after phase separation (dashed dotted line, 2); (b) resonance Raman spectra of Cyt. c in aqueous solution (dotted line, 1), in the IL1 phase (solid line, 3), and the IL1 phase without Cyt. c (dashed line, 4). | |
Resonance Raman spectroscopy was also carried out on Cyt. c to study the conformational changes around the heme. In the Cyt. c extracted from the IL phase, ν4, ν2, and ν3 bands were found at 1374, 1589, and 1506 cm−1, respectively (Fig. 4b(3)). In the Raman spectrum of Cyt. c in aqueous solution at pH 7.0 (Fig. 4b(1)), the ν4 band around 1373 cm−1 represents the valency of heme iron. Both ν2 (1585 cm−1) and ν3 (1503 cm−1) bands are useful in estimating both the coordination state and spin state, and these bands indicate a coordination number of six and a low spin state (6cLS). A small shift of each band would be due to the polarity difference of the solvents, and have been observed in non-aqueous solutions such as acetonitrile.24
Several methods have already been proposed for dissolving proteins in ILs. For dissolving proteins in ordinary hydrophobic ILs, modification of the proteins is necessary with amphiphilic polymers such as poly(ethylene oxide) (PEO).25,26 It has been reported that the addition of cyclic polyethers such as crown ether is also effective for tentative dissolution of proteins.27,28 The chemical modification involves multi-step reactions and purification steps. When proteins are extracted with excess amount of extractants relative to proteins, sonication is usually applied in spite of a fear of protein decomposition. In all methods, some complicated and time-consuming treatments are required, which easily cause denaturation of proteins. These treatments limited the application of hydrophobic ILs as solvents for proteins. In our methods, the proteins were immediately transported from aqueous to IL phase simply by changing the temperature for only a few degrees. The LCST-type phase behaviour is an effective treatment with little fear of protein denaturation. It should be mentioned that Cyt. c would be transferred into the IL1 phase by simple mixing for a long time. This is because the partition coefficient of Cyt. c in the IL1 phase was not the function of LCST behaviour. The use of the phase change considerably accelerated to reach the equilibrium. In spite of a lack of exact reason why this IL1 phase is more suitable for Cyt. c than an aqueous phase, this LCST-type phase transition process is very beneficial for protein extraction.
In the IL1/water mixture, the amount of water in the IL1 phase is expected to depend on the temperature.23 However, it is not easy to determine the water content at every temperature. We then synthesised ILs containing different water contents in the IL phase at the same temperature. To investigate the effect of the water in the IL phase on the extraction of Cyt. c, we mixed IL1 and more hydrophobic tributyloctylphosphonium N-trifluoromethanesulfonyl leucine (IL2). Both IL1 and IL2 were water saturated (30 and 13 wt%, respectively). By changing the mixing ratio of the IL1/IL2, we controlled the water content in the IL phase. The water content of the IL phase was determined by Karl Fischer titration. The water content of ILs at and around the phase transition temperature is fluctuated and is not easy to figure out. We used the water content of the ILs at 35 °C where the phase was stable and showed the accurate water content. The water content in the IL phase increased from 13 wt% (IL2 alone) to 21 wt% (1
:
1 by volume of IL1
:
IL2) (Fig. 5a). We used this mixture to extract Cyt. c with the same procedure as shown in Fig. 3. The distribution ratio (D) of Cyt. c in the mixed IL phase was also calculated from the absorbance of Cyt. c. The relation between the water content of the IL phase and the D value of Cyt. c is shown in Fig. 5b. This figure clearly shows that the Cyt. c was not extracted in the IL phase when the water content of the IL phase was below 13 wt%. Above this value, the D value gradually increased when 15 wt% or more of water was present in the IL phase. These results suggested that the water in the IL phase was closely related to the dissolution and stabilisation of proteins. Fujita et al. reported that a mixture of small amount of water and hydrophilic ILs having oxo acid residues was effective in dissolving proteins.13,14 Furthermore, these “hydrated ionic liquids” retained the stability of their secondary structure and prevented denaturation.16 One point we should mention here is that the IL1 having water less than 15 wt% still has the ability to solubilise Cyt. c. For example, the IL1 containing 6.5 wt% of water (two water molecules per ion pair) can dissolve Cyt. c. Transport of Cyt. c into the IL1 phase from the aqueous phase started when the water content of the IL1 was above 15 wt% as seen in Fig.5b. This is the matter of competitive solvation of Cyt. c in both phases. In the present study, a suitable amount of water (at least 21 wt%) is necessary to fully extract Cyt. c, and the hydrated state of ions may give an important role to control the affinity with proteins.
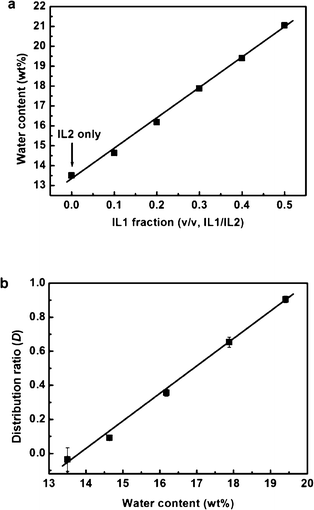 |
| Fig. 5 (a) Effect of ion species on the water content in the IL phase; (b) plot of the distribution ratio of Cyt. c as a function of the water content in the IL phase, using the mixture of IL1 and IL2. | |
Distribution ratio (D) of several proteins in IL1/water mixture
We confirmed that the IL1/water mixture was an optimal system for extracting Cyt. c stably in the IL phase from the aqueous phase. This IL1/water mixture was then examined for proteins. We examined typical heme proteins such as hemoglobin (Hb), myoglobin (Mb), and peroxidase (HRP) as well as several other proteins such as lysozyme (Lyz), chymotrypsin (Cht), laccase (Lac) and albumin (BSA). Heme proteins are used because they are readily detectable with visible spectroscopy. For these proteins, the distribution ratio varied from 1.0 to 0.01. Lyz, Cyt. c, Cht, Hb, and BSA were extracted into the IL1 phase, whereas Lac and HRP remained in the aqueous phase even after mixing with IL1 (Table 1).
Protein
a |
D
b
|
pI |
M
w/kDa |
Protein isoelectric point (pI) and molecular weight (Mw) were obtained from commercial source except for TEG-modified Cyt. c.
Calculated from the equation; D = (Absw-before − Absw-after)/Absw-before, where Absw-before and Absw-after denote the absorbance in an aqueous phase before and after the phase separation of IL1/water mixture, respectively.
Determined by an isoelectric focusing electrophoresis with PhastSystem (Pharmacia LKB Biotechnology) using PhastGel IEF 3–9.
Calculated according to the modification number of TEG on Cyt. c.25
|
Peroxidase (HRP) |
<0.01 |
7.2 |
44 |
TEG-modified Cyt. c |
0.02 ± 0.01 |
3.6c |
17d |
Laccase (Lac) |
0.11 ± 0.08 |
4.8 |
45 |
Myoglobin (Mb) |
0.91 ± 0.04 |
7.0 |
17 |
Albumin (BSA) |
>0.99 |
4.9 |
66 |
Hemoglobin (Hb) |
>0.99 |
7.0 |
65 |
Chymotrypsin (Cht) |
>0.99 |
8.6 |
25 |
Cytochrome c (Cyt. c) |
>0.99 |
10.2 |
12 |
Lysozyme (Lyz) |
>0.99 |
11.4 |
14 |
Since electrostatic interactions between the proteins and the IL1 phase are expected to contribute to the solubility of the protein, the surface charge of the proteins and their isoelectric point (pI) should influence the D value. To confirm the effect of the pI on the D value, the D value of the chemically modified protein was compared with that of native one. Polyethylene glycol (PEG) is an effective chemical modifier for improving the solubility of Cyt. c in molecular solvents including ILs without denaturation.25 Long PEG chains may change the solubility of the proteins, however, and these well-used long PEG chains are not suitable for this study. Instead, short PEG chains such as triethylene glycol (TEG) were selected to change the pI without affecting the solubility of Cyt. c. Thus prepared TEG-modified Cyt. c, approximately 12 out of 19 amino groups on the surface were modified by TEG, had lower pI value than that of native one. The D value of this TEG-modified Cyt. c lowered significantly (Table 1, TEG-modified Cyt. c), strongly suggesting that the pI of proteins is an important factor for the degree of extraction with IL1.
There have been a lot of reports evaluating the solubility of proteins using aqueous biphasic systems such as polyethylene glycol (PEG)/inorganic salt solution systems29 and IL/inorganic salt solution systems.30–32 In both cases, there are discussions on only partition coefficient of proteins in these biphasic systems. In the PEG/inorganic salt solution systems, Asenjo et al. suggested that the hydrophobicity of protein surface is one of major factors to affect the partition coefficient.29 Recently, Kragl et al. have also investigated the partition coefficient of several proteins in IL/inorganic salt solution systems.32 They suggested that the charges on the proteins acted as a major factor for the dissolution in the IL-rich phase. However, protein extraction in our biphasic system is not so simple. For example, HRP, pI 7.2, was expected to be extracted into the IL1 phase, but it was not (see Table 1). The difference in the partition coefficient between HRP and Hb cannot be explained only by pI value. This shows that there are other factors to govern the solubility in IL1 or partition coefficient of proteins. The HRP is known to have hydrophilic glycoside chains, which should tend to cause the HRP to remain in the aqueous phase. Since there are many proteins with different pI values or molecular weight, IL1 should be effective in extracting these proteins. Further study of the relation between the D value and other properties of proteins is now under way.
Separation of target protein from mixtures
Table 1 reveals that the distribution ratio (D) of the proteins in the IL1 phase varies widely. This observation led us to set up a protein separation process using this unique phase behaviour of the IL1/water mixture. We used Cyt. c and HRP, which have the highest and lowest D values respectively. We mixed 250 μl of Cyt. c and 250 μl of HRP to obtain 500 μl of mixed aqueous solution, to which 500 μl of the IL1 was added, and the resulting 1 ml of mixture was cooled to 20 °C to generate a homogeneous solution. The solution was then heated to 25 °C to induce phase separation. The proteins in the aqueous phase were dispensed with a pipette, and proteins dissolved in the IL1 phase were extracted again into the deionised water by adding dichloromethane.
Addition of hydrophobic organic solvent such as dichloromethane enhances the solubility of the proteins in an aqueous phase. This treatment is effective to take back the extracted protein(s) from the IL1 phase to an aqueous phase. The proteins isolated from each phase were analysed by 10% of SDS-PAGE, and the gel was stained with Coomassie brilliant blue R-250 (Wako Chem.). As shown in Fig. 6, both phases exhibited a single band, but in different positions. The lane for the IL1 phase (Fig. 6A) showed a band at 12.5 kDa, which we assigned to Cyt. c, whereas the lane for the water phase (Fig. 6B) showed a band at 44 kDa, corresponding to HRP. This shows that both Cyt. c and HRP were fully separated with the IL1/water mixture.
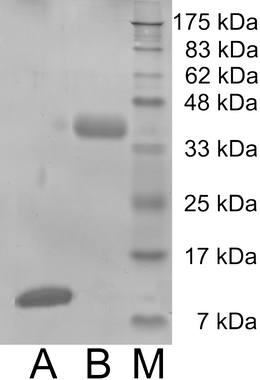 |
| Fig. 6
SDS-PAGE analysis of the IL bottom phase (B), the aqueous upper phase (A) after separation of Cyt. c and HRP. The band at 12.5 kDa was assigned to Cyt. c and the band at 44 kDa to HRP. Lane (M) shows molecular mass markers. | |
Since the IL1/water mixture was in a homogeneous phase at low temperature, and phase separation was induced by a small temperature increase, protein separation was successfully performed under mild conditions with little fear of thermal denaturation of the proteins. Several proteins could be separated at once by designing ILs having different affinities for proteins. The temperature sensitive phase change of the IL/water mixture permits the design of a novel reaction or extraction solvents for biopolymers, and widens the potential applicability of ILs in diverse biomedical and pharmaceutical fields. It should be noted here that not all of ILs show such a temperature sensitive and reversible phase behaviour after mixing with water. Furthermore, extraction of proteins cannot always be carried out with IL/water mixtures even though the water content of IL was around 21%. These are very structure-oriented properties.
Conclusion
Cyt. c was readily extracted from the aqueous phase to the ionic liquid (IL) phase. The IL contained at least 21 wt% of water after phase separation effectively solubilised Cyt. c. This hydrated IL was also effective for solubilising lysozyme, chymotrypsin, hemoglobin, albumin, and myoglobin. The distribution ratio (D) of proteins in IL/water mixture depended on the isoelectric point (pI) of proteins and other factors as well. Considering the difference in the D value, selective extraction of proteins from mixtures was carried out using the IL/water mixture.
Acknowledgements
This study was supported by a Grant-in-Aid for Scientific Research from Japan Society for the Promotion of Science (No. 21225007).
References
- J. S. Wilkes and M. J. Zaworotko, J. Chem. Soc., Chem. Commun., 1992, 965 RSC.
- T. Welton, Chem. Rev., 1999, 99, 2071 CrossRef CAS.
-
P. Wasserscheid and T. Welton, Ionic Liquids in Synthesis, Wiley-VCH, 2003 Search PubMed.
- M. J. Earle, J. M. S. S. Esperanca, M. A. Gilea, J. N. C. Lopes, L. P. N. Rebelo, J. W. Magee, K. R. Seddon and J. A. Widegren, Nature, 2006, 439, 831 CrossRef CAS.
- R. A. Sheldon, R. M. Lau, M. J. Sorgedrager, F. van Rantwijk and K. R. Seddon, Green Chem., 2002, 4, 147 RSC.
- J. L. Kaar, A. M. Jesionowski, J. A. Berberich, R. Moulton and A. J. Russell, J. Am. Chem. Soc., 2003, 125, 4125 CrossRef CAS.
- F. van Rantwijk and R. A. Sheldon, Chem. Rev., 2007, 107, 2757 CrossRef.
- R. Mareria Lau, F. van Rantwijik, K. R. Seddon and R. A. Sheldon, Org. Lett., 2000, 2, 4189 CrossRef CAS.
- U. Kragl, M. Eckstein and N. Kaftzik, Curr. Opin. Biotechnol., 2002, 13, 565 CrossRef CAS.
- S. N. Baker, T. M. McCleskey, S. Pandey and G. A. Baker, Chem. Commun., 2004, 940 RSC.
- M. B. Turner, S. K. Spear, J. G. Huddleston, J. D. Holbrey and R. D. Rogers, Green Chem., 2003, 5, 443 RSC.
- D. Sate, M. H. A. Janssen, G. Stephens, R. A. Sheldon, K. R. Seddon and J. R. Lu, Green Chem., 2007, 9, 859 RSC.
- K. Fujita, D. R. MacFarlane and M. Forsyth, Chem. Commun., 2005, 4804 RSC.
- K. Fujita, M. Forsyth, D. R. MacFarlane, R. W. Reid and G. D. EIliott, Biotechnol. Bioeng., 2006, 94, 1209 CrossRef CAS.
- N. Byrne, L. M. Wang, J. P. Belieres and C. A. Angell, Chem. Commun., 2007, 2714 RSC.
- K. Fujita, D. R. MacFarlane, M. Forsyth, M. Yoshizawa-Fujita, K. Murata, N. Nakamura and H. Ohno, Biomacromolecules, 2007, 8, 2080 CrossRef CAS.
- K. Fujita, N. Nakamura, K. Igarashi, M. Samejima and H. Ohno, Green Chem., 2009, 11, 351 RSC.
- M. Armand, F. Endres, D. R. MacFarlane, H. Ohno and B. Scrosati, Nat. Mater., 2009, 8, 621 CrossRef CAS.
- K. Fukumoto, M. Yoshizawa and H. Ohno, J. Am. Chem. Soc., 2005, 127, 2398 CrossRef CAS.
- J. Kagimoto, K. Fukumoto and H. Ohno, Chem. Commun., 2006, 2254 RSC.
- K. Fukumoto and H. Ohno, Chem. Commun., 2006, 3081 RSC.
- H. Ohno and K. Fukumoto, Acc. Chem. Res., 2007, 40, 1122 CrossRef CAS.
- K. Fukumoto and H. Ohno, Angew. Chem., Int. Ed., 2007, 46, 1852 CrossRef CAS.
- S. G. Sivakolundu and P. A. Mabrouk, J. Am. Chem. Soc., 2000, 122, 1513 CrossRef CAS.
- N. V. Kawahara and H. Ohno, Solid State Ionics, 1998, 115, 161 CrossRef.
- K. Nakashima, T. Maruyama, N. Kamiya and M. Goto, Chem. Commun., 2005, 4297 RSC.
- K. Shimojo, K. Nakashima, N. Kamiya and M. Goto, Biomacromolecules, 2006, 7, 2 CrossRef CAS.
- K. Shimojo, N. Kamiya, F. Tani, H. Naganawa, Y. Naruta and M. Goto, Anal. Chem., 2006, 78, 7735 CrossRef CAS.
- B. A. Andrews, A. S. Schmidt and J. A. Asenjo, Biotechnol. Bioeng., 2005, 90, 380 CrossRef CAS.
- Z. Du, Y. L. Yu and J. H. Wang, Chem.–Eur. J., 2007, 13, 2130 CrossRef CAS.
- S. Dreyer and U. Kragl, Biotechnol. Bioeng., 2008, 99, 1416 CrossRef CAS.
- S. Dreyer, P. Salim and U. Kragl, Biochem. Eng. J., 2009, 46, 176 CrossRef CAS.
|
This journal is © The Royal Society of Chemistry 2011 |
Click here to see how this site uses Cookies. View our privacy policy here.