DOI:
10.1039/C0PY00348D
(Paper)
Polym. Chem., 2011,
2, 672-678
Dual-stimuli sensitive nanogels fabricated by self-association of thiolated hydroxypropyl cellulose
Received
22nd October 2010
, Accepted 15th November 2010
First published on 25th November 2010
Abstract
A new type of cellulose derivative was synthesized by means of conjugating cysteamine to hydroxypropyl cellulose (HPC) and the degree of thiol groups could be controlled by the feed ratio of the reactants. The thiolated HPC (HPC–SH) maintains the thermosensitivity of HPC and the thiol groups on the HPC chain can be oxidized to disulfide bonds. Cytotoxity tests performed on MG-63 cells proved that HPC–SH is not harmful to the cells. Nanogels can be fabricated by the self-association of HPC–HS in the solution at 45 °C and then oxidation of thiol groups to disulfide bonds occurs to stabilize the associated structure. The crosslinking degree of the nanogels could be controlled by the substitution degree of thiol groups (–SH) in the thiolated HPC. The hydrodynamic radius of the nanogels can be tuned by adjusting the degree of crosslinking and the concentration of the initial thiolated HPC solution in the self-association process. The hydrodynamic radius of the nanogels can be changed with the temperature and the dissociation process can happen by adding the reducing agent dithiothreitol (DTT). The dual-stimuli sensitive nanogels may have potential applications in controlled drug release, transfer switch device and sensors.
1. Introduction
Nanogels contain interior networks and can be swollen in water or physiological fluid with the characteristics of both hydrogels and nanoparticles.1 The small size of the nanogels offers some additional properties, such as a large surface area for bioconjugation, the ability to trap biomolecules (e.g. drugs, proteins, carbohydrates and DNA inside the gels) and quick response to external stimuli.1–3 The specific properties offer the potential applications of nanogels in drug and gene delivery,1–8 bio-imaging,9 catalysis,10–12 nanoreactors,13 sensing devices,14 and optical switching.15
Generally, free radical copolymerization of monomers with divinyl monomers and crosslinking of macromolecules with crosslinking agents such as glutaraldehyde and divinylsulfone were used for the preparation of nanogels.1,2,16–20 However, the crosslinking agents are generally cytotoxic and difficult to eliminate completely from the resultant nanogels. Moreover, most of the nanogels that are reported in the literature deal with response to a single stimulus and are not dissociable. All these factors go against further biomedical applications.
In recent years, disulfide bonds/thiols have attracted increasing attentions as their important role in biology. For example, disulfide bonds can contribute to a protein's tertiary structure or contribute to the quaternary structure of multi-unit proteins by forming fairly strong covalent bonds between different peptide chains. Moreover, the disulfide bond can be reduced to thiol in mild conditions by dithiothreitol (DTT)21 or glutathione.22 Meanwhile, thiol can also be re-oxidized to form disulfide bonds by oxygen23 or dimethyl sulfoxide.24–26 There is a high redox potential difference (∼100–1000 folds) between the reducing intracellular space and oxidizing extracellular space that largely developed by redox status of glutathione.27–29 The redox-sensitive properties of disulfide bonds/thiols provides a new promising physiologically compatible strategy for preparing dissociable materials, such as hydrogels and micelles for drug and gene delivery.23,29–34
Hydroxypropyl cellulose (HPC) is a nontoxic and biodegradable alkyl-substituted cellulose derivative and has been approved by the United States Food and Drug Administration (FDA) to be used widely in medicine, food and cosmetics. HPC has a LCST at around 41–45 °C which is due to the degree of subustitution of hydroxypropyl groups.18,35 Above the lower critical solution temperature (LCST), HPC chains will collapse to nanospheres. HPC nanogels have been prepared by chemically crosslinking the collapsed HPC chains by using divinylsulfone at pH 12–13.18–20 Large amounts of hydroxy groups on the HPC chains offer the chance of further modification, which provide the potential to fabricate new functional materials by using HPC. However, the crosslinked structures in the resultant HPC microgels are not dissociable and toxic crosslinker has been used, which may result in problems for further application of the HPC nanogels. In this work, thiolated hydroxypropyl cellulose derivatives (HPC-SH) were synthesized without destroying the thermo-sensitive property of HPC and HPC nanogels were prepared from the thiolated HPC by the self-association of HPC chains and crosslinked by disulfide bonds of thiolated HPC. Neither monomer nor crosslinker were used in the preparation of HPC nanogels in present approach and the resultant nanogels show the thermo- and redox-sensitivities.
2. Experimental
2.1. Materials
Hydroxypropyl cellulose (Aldrich. Mw = 3.9 × 104 g mol−1, Mw/Mn = 2.8, estimated by using GPC) was dried in vacuum over phosphorus pentoxide at 40 °C for three days. 4-Nitrophenyl chloroformate (4-NC) (Alfa Aesar), cysteamine (Fluka), DL-dithiothreitol (DTT) (Amresco), 5,5′-dithiobis-(2-nitrobenzoic acid) (DTNB, Ellman's reagent) (Alfa Aesar), dimthyl sulfoxide (DMSO) (Alfa Aesar) and 2-mercaptoethanol (Aladdin) were used as received. Dichlormethane, pyridine and dimethylformamide (DMF) were dried over calcium hydride and distilled prior to use. Methylthiazolyldiphenyl-tetrazolium bromide (MTT) (5 mg mL−1, Sigma) was maintained in 4 °C. Dulbecco's modified Eagle's medium (DMEM), fetal bovine serum (FBS), phosphate-buffered saline (PBS, pH = 7.4), penicillin and streptomycin were purchased from Gibico. MG-63 human osteosarcoma cells were provided by Graduate University of Chinese Academy of Sciences.
2.2. Synthesis of thiolated HPC derivatives
Thiolated hydroxypropyl cellulose (HPC-SH) was synthesized by a two-step reaction and the synthesis route is shown schematically in Scheme 1. In detail, HPC was first activated by 4-NC to obtain 4-nitrophenyl carbonate group substituted HPC (HPC–4-NC). The typical procedure for the activation of HPC by 4-NC is as follows. HPC (2.5 g) was first dissolved in 80 mL CH2Cl2 and then a certain amount of 4-NC and the catalyst pyridine (20 mL) were added. The reaction mixture was stirred for 4 h at 0 °C to obtain HPC–4-NC. Thereafter, the resultant HPC–4-NC was purified by means of precipitation in anhydrous diethyl ether three times to remove the pyridine and unreacted 4-NC. The degree of substitution with the nitrophenyl group can be controlled by varying the feed ratio of HPC and 4-NC. The purified HPC–4-NC (2 g) was then dissolved in 80 mL DMF and cysteamine was added. The molar ratio of cysteamine to 4-nitrophenyl carbonate groups in HPC–4-NC is 10. The reaction mixture was stirred for 24 h at room temperature under N2 atmosphere and then precipitated in diethyl ether three times and dried under vacuum overnight to obtain thiolated HPC (HPC–SH). In order to eliminate the formation of disulfide bonds during the synthesis and purification procedure, the purified HPC–SH was deoxidized by DTT (molar ratio of DTT to original nitrophenyl groups is 20) in 80 mL DMF at 40 °C under N2 atmosphere for 8 h and then dialyzed in water under the protection of N2 for three days and finally lyophilized.
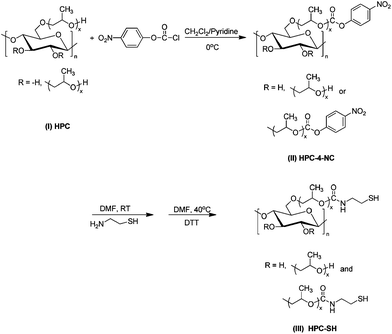 |
| Scheme 1 The synthetic route for the thiolated HPC derivatives. | |
2.3. Preparation of disulfide-crosslinked nanogels
A certain amount of HPC–SH was dissolved in 40 mL DMF with the addition of 0.015 g DTT. The solution was agitated at 40 °C for 24 h to eliminate the disulfide bonds that formed during the storage of HPC–SH. The HPC–SH solution was then dialyzed against water under the protection of N2 for two days to get the HPC–SH aqueous solution. The HPC–SH aqueous solution was heated to 45 °C, above the LCST of HPC, by which the HPC chains were collapsed to a globule. Subsequently, dimethyl sulfoxide (DMSO) (1%, v/v) was added to the solution as an oxidant and the solution was reacted at 45 °C for 4 days, during which disulfide bonds were formed to crosslink the collapsed HPC chains to nanogels. The resultant nanogels were dialyzed in water for 2 days to get rid of DMSO. To trace the content of the residual thiol groups in solution, 1 mL sample solution was taken every 12 h and then analyzed by Ellman's method.36,37 The final conversion of the thiol groups can be estimated by means of the amount of the dialyzed nanogels. By changing initial concentration and substitution degree of thiol groups (DSSH), various nanogels were prepared in our research.
2.4 Cytotoxicity tests for the HPC–SH
The culture medium of cells is DMEM with 10% FBS and 1% penicillin and streptomycin. 200 μL of MG-63 human osteosarcoma cells in the culture medium with a concentration of 5.0 × 104 cells mL−1 was added to the wells in a 96-well plate and incubated for 24 h. Then 0.8 mg HPC–SH0.078 was added to the culture medium, corresponding to the polymer concentration of 4 mg mL−1. The cells were treated in the culture medium for 24 h, 48 h and 72 h respectively. After incubation for the given time, the supernatants were removed and washed by PBS for three times, and then 200 μL culture medium and 20 μL 5 mg mL−1 MTT solution were added and incubated for 4 h at 37 °C in 5% CO2. Cell viability was measured by the methylthiazolyl-diphenyl-tetrazolium bromide (MTT, 5 mg mL−1) method. Thereafter the supernants was removed and 150 μL of DMSO was added to dissolve precipitated formazan crystals. The optical density (OD) value was measured by the microplate reader (MULTISKAN MK3, Thermo Electron Corporation) at wavelength of 570 nm immediately. Percentage of cell viability was calculated by comparison the OD value of treated cells to untreated cells. All experiments were tested in triplicate.
2.5. Characterization
1H NMR analysis was carried out with a Bruker DMX 400 NMR spectrometer. The molecular weight distribution of derivatives was measured by means of gel permeation chromatography (GPC) using THF as the eluent and PS as the standard. GPC experiments were carried out on a system equipped with a Waters 515 HPLC pump, a Waters 2410 differential refractive index detector, and three Waters Styragel columns (HR1, HR3, HT4).
The degree of substitution (DS) of thiol groups was measured by 1HNMR and Ellman's method.36,37 In Ellman's method, an absorption peak at 412 nm would be recorded on a Shimadzu UV-1601PC spectrophotometer for diluted thiolated HPC solutions in pH = 7.4 phosphate buffer solution. The concentration of free thiol groups can be calculated by using a calibration curve, which was derived from mercaptoethanol standard solutions.36,371H NMR analysis was carried out with a Bruker DMX 400 NMR spectrometer. To determine the LCST of HPC and HPC–SH, HPC and HPC–SH (2 mg mL−1) solutions were prepared under the protection of N2 and transferred to a stoppered cuvette and the solution was sealed. The optical transmittance of 2 mg mL−1 HPC and HPC–SH solutions at a wavelength of 500 nm were recorded as a function of temperature with a heating rate of 0.2 °C min−1 on a Shimadzu UV-2450 spectrophotometer with a temperature control device.
Dynamic light scattering (DLS) experiments were carried out on a commercial spectrometer (AlV/SP-150) equipped with an ALV-5000 multi-τ digital time correlator and a solid-state laser (ADLS DPY 425II, output power about 400 mW at λ = 632.8 nm) was used as the light source. All the copolymer solutions were filtered through the waterman nylon filter (0.45 μm) before DLS experiments. All of the DLS experiments were carried out at a scattering angle of 90°. The hydrodynamic radius (<Rh>) was obtained by the CONTIN program. The thermosensitivity was characterized by multistep heating of the solution from 25 to 50 °C. The samples were equilibrated for at least 30 min at each temperature that measured. The reduction-sensitivity of the nanogels was characterized by monitoring the scattering intensity of the nanogels in deionized water or in phosphate buffered saline at the scattering angle of 90° at 25 °C after adding DTT with 10 mg mL−1 concentration.
3. Results and discussion
3.1. Synthesis of the thiolated HPC
The synthesis route of the thiolated HPC (HPC–SH) is shown schematically in Scheme 1. The first step is to synthesise 4-nitrophenyl carbonate substituted HPC (HPC–4-NC) by activating the hydroxy group of HPC with 4-nitrophenyl chloroformate (4-NC). The DS of 4-nitrophenyl carbonate group (DS4-NC) can be controlled by modulating the weight ratio of HPC and 4-NC.37–40 Then, the aminolysis of HPC–4-NC in DMF was carried out by adding 10 fold cysteamine in solution to obtain HPC–SH.37–40 The disulfide bonds formed in case during the synthesis and purifying procedure of the HPC–SH were reduced into thiol groups by adding DTT. Fig. 1 shows the 1H NMR spectra of the HPC–4-NC and HPC–SH with different degree of substitution. The successful synthesis of HPC–4-NC can be confirmed by 1H NMR (CDCl3) spectra as shown in Fig. 1a. The peaks at δ = 7.4 and 8.3 ppm are attributed to the aromatic protons of the nitrophenyl group. The peaks at δ of 8.0, 8.5 and 8.9 ppm come from the pyridinium chloride that formed during the synthesis of HPC–4-NC, which is hard to eliminate from the system by precipitating the reaction mixture in diethyl ether. Moreover HPC–4-NC can be easily hydrolyzed in water, it is impossible to eliminate the pyridinium chloride by dialyzing HPC–4-NC in water. Fortunately, the pyridinium chloride in HPC–4-NC has no influence on the following reaction and can be eliminated completely from the final HPC–SH derivatives. The 1H NMR spectra of the HPC–SH with different degree of substitution of thiol groups are shown in Fig. 1b. It shows that the peaks from the aromatic protons were disappeared, which indicates the complete remove of the 4-NC groups and the elimination of pyridinium salts. Meanwhile, the new peak at δ = 2.6 ppm comes from the methylene group of –CH2–SH, confirms the success of the synthesis of HPC–SH. HPC–SH with low DSSH can dissolve in water, D2O was chosen as a deuterated reagent while HPC–SH with high DSSH doesn't dissolve in water. The CDCl3 with trace D2O was chosen as deuterated reagent in which trace D2O was used to eliminate the peaks of active hydrogen of HPC–SH.
DSSH of HPC-SH can be estimated by 1H NMR and Ellman's method.36,37 In the case of estimating DSSH using 1H NMR, the DS of the 4-nitrophenyl carbonate groups in HPC–4-NC can be estimated by means of the ratio of integral peak area of the methyl group of HPC at δ = 1.1 ppm and aromatic protons of the nitrophenyl group or by comparing the integral peak area of the –CH2– protons of –CH2–SH groups with that of the –CH3 groups of hydroxypropyl groups on HPC chains. The results of the feed ratio and the DS of resultant HPC–SH are listed in Table 1. The results show that the DS4-NC of HPC–4-NC varied with the initial feed ratios of HPC and 4-NC and the DSSH of HPC–SH determined by 1H NMR are similar and close to those DS4-NCs of HPC–4-NC under a certain feed ratio. The DSSH measured by Ellman's method is adopted in the context of this paper and denoted as HPC–SHx, where x is the average number of thiol groups per glucose ring of HPC. The HPC–SH0.015 and HPC–SH0.078 can dissolve in water well, but the HPC–SH with high DSSH is hard to dissolve in water because the thiol group is more hydrophobic than hydroxy group. The molecular weight of HPC–SH with low DSSH was estimated by GPC. The results show that the molecular weight and its distribution of the thilated HPC are similar to those of HPC.
Table 1 The feed ratio of synthesis of HPC–4-NC and degree of substation of 4-nitrophenyl carbonate groups and thiol groups
m
HPC/g |
m
4-NC/g |
DS4-NCa |
DSSHa |
DSSHb |
M
n/g mol−1 |
M
w/Mn |
Determined by 1H NMR.
Determined by Ellman's assay.
|
2.5 |
0.45 |
0.014 |
0.02 |
0.015 |
4.0 × 104 |
3.0 |
2.5 |
0.68 |
0.073 |
0.09 |
0.078 |
4.7 × 104 |
2.4 |
2.5 |
0.90 |
0.24 |
0.24 |
0.24 |
— |
— |
2.5 |
1.35 |
0.51 |
0.54 |
0.57 |
— |
— |
It is well-known that HPC is a thermally sensitive polymer and has a low critical solution temperature (LCST) of ∼41 °C at the dilute concentration of ∼1 × 10−4 g mL−1 in water.18 In this work, we estimated the LCST of the HPC–SH and compared it to HPC. Fig. 2 shows the transmittance of the HPC–SH solutions as a function of temperature. The results show that the substitution of thiol groups leads to a slightly decrease in LCST compared with HPC. Moreover, a higher DSSH corresponds to a lower LCST. This is because the hydrogen bonds between thiol groups and water are much weaker than those between –OH groups and water, which leads to the decrease in LCST with increasing DSSH. It should be noted that the increasing tendency of transmittance at around 60 °C is because the flocculated polymer starts to precipitate during the measurement.
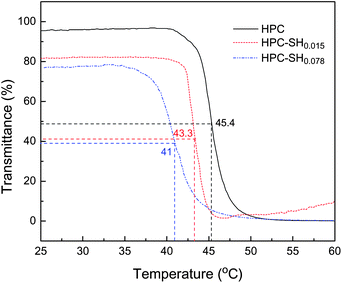 |
| Fig. 2 The transmittance of HPC and HPC–SH with different DSSH as a function of temperature. | |
3.2.
In vitro cytotoxicity of HPC–SH
The cytotoxicity of HPC–SH was valued on MG-63 osteosarcoma cells by MTT assay. 0.8 mg HPC–SH0.078 was used to treat the cells in every well. The proportion of viable cells in the treated group was compared to that of the negative control. Fig. 3 shows the cell viability (%) as a function of time. The results indicate that the cell viability is about 100% after 48 h incubation and 75% after 72 h incubation, which suggests that HPC is not harmful to the cells.
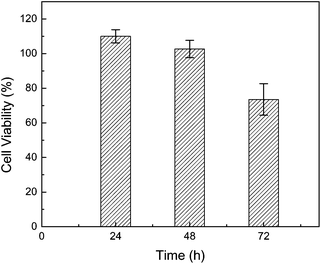 |
| Fig. 3 Percentage of cell viability as a function of time. | |
3.3. Preparation of HPC Nanogels
The above results indicate HPC–SH0.015 and HPC–SH0.078 derivatives have a similar thermosensitivity to HPC. In this work, HPC nanogels were prepared by the self-crosslinking of HPC–SH with the formation of disulfide bonds. The preparation and thermal sensitivity of the resultant HPC nanogels are shown schematically in Scheme 2. Firstly, the HPC–SH derivatives were heated to a temperature above their LCST, by which the HPC–SH chains collapsed to globules. Then, the oxidant DMSO was added to the HPC–SH solutions and the solutions were kept at the temperature above the LCST of HPC–SH, during which the –SH groups were oxidized to disulfide bonds and the globule HPC chains were crosslinked to form the nanogels.24–26,41,42 The conversion of –SH groups to disulfide bonds during the crosslinking procedure were estimated by measuring the concentration of –SH groups in the system by Ellman's method. Fig. 4 shows the conversion of –SH groups of HPC–SH0.078 as a function of temperature at different polymer concentration. The results indicate that the conversion of the –SH groups to disulfide bonds is faster in the first 48 h and become slower thereafter. After oxidized with DMSO for 4 days and dialysis for 2 days, about 80% of –SH groups were conversed into disulfide bonds. It seems that further conversion of thiol groups to disulfide bonds is quite difficult, which may be attributed to that the left –SH groups are embedded in the nanogels and protect them to be oxidized. The polymer concentration of the HPC–SH0.078 solutions has slight influence on the hydrodynamic radius (<Rh>) of the resultant nanogels. When the polymer concentration increased from 0.19 mg mL−1 to 0.70 mg mL−1, the <Rh> of the nanogels increased from 72 nm to 88 nm (Fig. 5) at 25 °C.
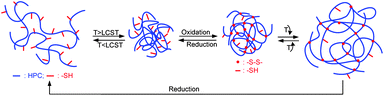 |
| Scheme 2 The fabrication of HPC nanogels from HPC–SH and the dual-stimuli sensitivity. | |
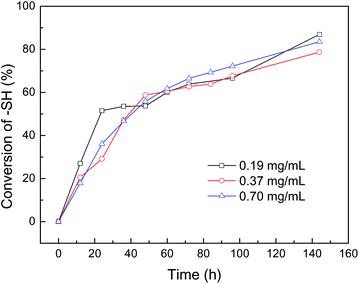 |
| Fig. 4 The conversion of thiol groups to disulfide bonds as a function of oxidizing during the preparation of HPC nanogels from HPC–SH. | |
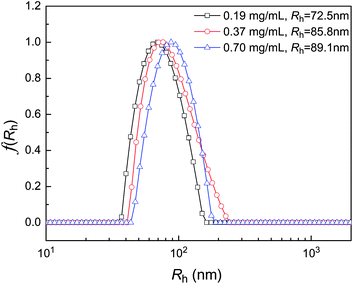 |
| Fig. 5 Hydrodynamic radius distribution and <Rh> of HPC nanogels prepared from HPC–SH0.078 at different initial polymer concentration. | |
When the HPC nanogels were prepared from HPC–SH0.015, the concentration of the –SH groups is quite low and it is hard to estimate the conversion of thiol groups to disulfide bonds by Ellman's method. Anyway, it can be imagined that the crosslink density of the HPC nanogels from HPC–SH0.015 should be lower than those prepared from HPC–SH0.078 due to the lower content of –SH groups in the precursor polymer. Fig. 6 shows the hydrodynamic radius distribution of HPC nanogels prepared from HPC–SH0.015 and HPC–SH0.078 solutions with similar polymer concentration at 25 °C. The results show that the <Rh> of HPC nanogels prepared from HPC–SH0.078 has a narrowly unimodal distribution with the average hydrodynamic radius of 86 nm. Whereas the <Rh> of HPC nanogels prepared from HPC–SH0.015 shows a two peaks modal distribution with a <Rh> of around 162 nm. The peaks at the position of 11 nm and 165 nm come from the unimolecule of HPC and multi-molecule crosslinked HPC–SH nanogels, respectively. The <Rh> and its distribution of the disulfide bond crosslinking HPC hydro-nanogels are similar to those self-associate HPC nanogels.18–20
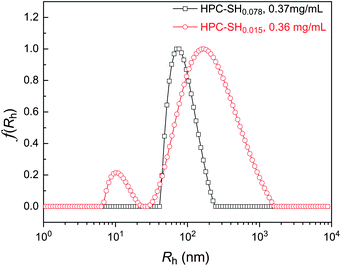 |
| Fig. 6 Hydrodynamic radius distribution of the HPC nanogels prepared from HPC–SH with different DSHS at similar initial concentration. | |
3.3. Thermo- and redox-sensitivity of HPC naonogels
The HPC nanogels prepared from HPC–SH show similar thermosensitivity to that of HPC. Fig. 7 shows the <Rh> of the HPC–SH nanogels as a function of temperature. The nanohydrogels prepared from HPC–SH0.078 have a high crosslink density compared to that of the nanogel prepared from HPC–SH0.015 as discussed above. Therefore, the change of <Rh> of the HPC–SH0.078 nanogels as a function of temperature is less obvious than those HPC–SH0.015 nanogels. Moreover, the <Rh> of HPC–SH0.015 and HPC–SH0.078 nanogels reaches its minimum at about 44 and 46 °C, respectively. Below these temperatures, the changes of the <Rh> are mainly attributed to the collapse of the HPC chains. Further increase in the temperature leads to the aggregation of the HPC nanogels, which results the increase in the <Rh>. It was found that the thermally induced change in <Rh> is reversible. Fig. 8 shows the <Rh> for 25 ↔ 45 °C cycles. The results show that <Rh> remains at almost constant values at 25 and 45 °C.
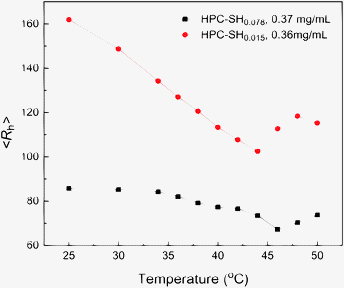 |
| Fig. 7 The average hydrodynamic radius of HPC nanogels prepared from HPC–SH0.078 and HPC–SH0.015 as a function of temperature. | |
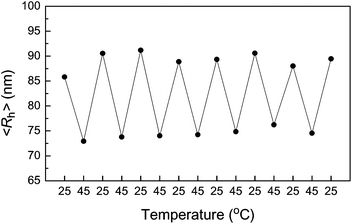 |
| Fig. 8 The average hydrodynamic radius of HPC–SH0.078 nanogels in 25 ↔ 45 °C cycles. | |
The thiol/disulfide redox is important in biology and can be used in redox switches and signaling.43–46 The disulfide bonds crosslinked HPC nanogels should have redox-sensitivity. Fig. 9 shows the scattering light intensity of the nanogel solution as a function of reduction time by using DTT in either deionized water or phosphate buffered saline (PBS) solution (pH =7.4). The concentration of the DTT is 10 mg mL−1. DTT could reduce the disulfide bonds to thiols, which resulted in the dissociation of the nanogels. The decrease in the scattering intensity at the detection angle of 90° in Fig. 9 indicated the cleavage of disulfide bonds and the dissociation of the nanogels. Moreover, the dissociation rate of the disulfide bonds crosslinking HPC nanogels in PBS buffer is much faster than that in water, which is due to that the reducing property of DTT depends on the pH of the solution, for example, the redox potential of DTT is −0.332 V at pH = 7.0 and −0.366 V at pH 8.1.21 The reducing procedure of the HPC nanogels by DTT could be completed within 20 min in PBS buffer (pH = 7.4), whereas it took about 10 h in water. The result indicates disulfide bonds crosslinked micelles could dissociate under reductive conditions and physiological environment such as the intracellular environment.
The preparation and dual-stimuli sensitive properties of the HPC nanogels are shown schematically in Scheme 2. The first step for the preparing HPC nanogels from HPC–SH was to heat the HPC–SH dilute solution to a temperature above its LCST, during which HPC polymer chains associated and collapsed to globules. This step is similar to Hu et al.'s work.18–20 The HPC globules were then crosslinked by directly oxidizing the thiol groups to disulfide bonds, avoiding the use of toxic crosslinkers. The HPC nanogels have the redox-sensitivity and reversible thermosensitivity.
4. Conclusion
The thiolated hydrodroxypropyl cellulose (HPC-SH) has been designed and synthesized. The degree of substitution of –SH groups slightly influences the LCST. The LCST is decreased from 45 °C for HPC to 43 and 41 °C for the HPC–SH with DSSH of 0.015 and 0.078, respectively. Cytotoxity tests performed on MG-63 cells proved that HPC–SH is not harmful for the cells. The thermal and redox sensitive HPC nanogels were prepared without using toxic crosslinkers and the correlated sensitivities of the HPC nanogels have been investigated. The size of the HPC nanogels depends on the HPC–SH concentration and the crosslink density of the nanogels. The <Rh> of the HPC nanogel increases with increasing HPC–SH concentration and decreases with increasing crosslink density. The HPC nanogels have the reversible thermal and redox sensitivities, which have potential stimuli-responsive applications in the drug controlled release, transfer switch device and sensors.
Acknowledgements
The financial support of the National Natural Science Foundation of China (Grant No. 20974114, 20774105, and 50821062) and the Knowledge Innovation Program of the Chinese Academy of Sciences (Grant No. KJCX2-YW-H19) is greatly appreciated.
Notes and references
- J. K. Oh, R. Drumright, D. J. Siegwart and K. Matyjaszewski, Prog. Polym. Sci., 2008, 33, 448–477 CrossRef CAS.
- J. K. Oh, D. J. Siegwart and K. Matyjaszewski, Biomacromolecules, 2007, 8, 3326–3331 CrossRef CAS.
- K. Raemdonck, J. Demeester and S. De Smedt, Soft Matter, 2009, 5, 707–715 RSC.
- Y. S. Shin, J. H. Chang, J. Liu, R. Williford, Y. K. Shin and G. J. Exarhos, J. Controlled Release, 2001, 73, 1–6 CrossRef CAS.
- S. V. Vinogradov, E. V. Batrakova and A. V. Kabanov, Bioconjugate Chem., 2004, 15, 50–60 CrossRef CAS.
- J. I. Lee and H. S. Yoo, Eur. J. Pharm. Biopharm., 2008, 70, 506–513 CrossRef CAS.
- L. J. Shi, S. Khondee, T. H. Linz and C. Berkland, Macromolecules, 2008, 41, 6546–6554 CrossRef CAS.
- W. H. Blackburn, E. B. Dickerson, M. H. Smith, J. F. McDonald and L. A. Lyon, Bioconjugate Chem., 2009, 20, 960–968 CrossRef CAS.
- U. Hasegawa, S. I. M. Nomura, S. C. Kaul, T. Hirano and K. Akiyoshi, Biochem. Biophys. Res. Commun., 2005, 331, 917–921 CrossRef CAS.
- M. Yan, Z. X. Liu, D. N. Lu and Z. Liu, Biomacromolecules, 2007, 8, 560–565 CrossRef CAS.
- D. Carboni, K. Flavin, A. Servant, V. Gouverneur and M. Resmini, Chem.–Eur. J., 2008, 14, 7059–7065 CAS.
- S. Kinge, M. Crego-Calama and D. N. Reinhoudt, ChemPhysChem, 2008, 9, 20–42 CrossRef CAS.
- M. Oishi, H. Hayashi, T. Uno, T. Ishii, M. Iijima and Y. Nagasaki, Macromol. Chem. Phys., 2007, 208, 1176–1182 CrossRef CAS.
- C. Gota, K. Okabe, T. Funatsu, Y. Harada and S. Uchiyama, J. Am. Chem. Soc., 2009, 131, 2766–2767 CrossRef CAS.
- C. E. Reese, A. V. Mikhonin, M. Kamenjicki, A. Tikhonov and S. A. Asher, J. Am. Chem. Soc., 2004, 126, 1493–1496 CrossRef CAS.
- C. Chang, Z. C. Wang, C. Y. Quan, H. Cheng, S. X. Cheng, X. Z. Mang and R. X. Zhuo, J. Biomater. Sci., Polym. Ed., 2007, 18, 1591–1599 CAS.
- M. Oishi, H. Hayashi, I. D. Michihiro and Y. Nagasaki, J. Mater. Chem., 2007, 17, 3720–3725 RSC.
- J. Gao, G. Haidar, X. H. Lu and Z. B. Hu, Macromolecules, 2001, 34, 2242–2247 CrossRef CAS.
- Z. B. Hu, X. H. Lu, J. Gao and C. J. Wang, Adv. Mater., 2000, 12, 1173–1176 CrossRef CAS.
- X. H. Lu, Z. B. Hu and J. Gao, Macromolecules, 2000, 33, 8698–8702 CrossRef CAS.
- W. W. Cleland, Biochemistry, 1964, 3, 480–482 CrossRef CAS.
- H. Ostergaard, C. Tachibana and J. R. Winther, J. Cell Biol., 2004, 166, 337–345 CrossRef CAS.
- Y. Kakizawa, A. Harada and K. Kataoka, J. Am. Chem. Soc., 1999, 121, 11247–11248 CrossRef CAS.
- T.
J. Wallace, J. Am. Chem. Soc., 1964, 86, 2018–2021 CrossRef CAS.
- T. J. Wallace and J. J. Mahon, J. Am. Chem. Soc., 1964, 86, 4099–4103 CrossRef CAS.
- T. J. Wallace and J. J. Mahon, J. Org. Chem., 1965, 30, 1502–1506 CAS.
- D. P. Jones, J. L. Carlson, V. C. Mody, J. Cai, M. J. Lynn and P. Sternberg, Free Radical Biol. Med., 2000, 28, 625–635 CrossRef CAS.
- G. Saito, J. A. Swanson and K. D. Lee, Adv. Drug Delivery Rev., 2003, 55, 199–215 CrossRef CAS.
- S. Ganta, H. Devalapally, A. Shahiwala and M. Amiji, J. Controlled Release, 2008, 126, 187–204 CrossRef CAS.
- X. Z. Shu, Y. C. Liu, Y. Luo, M. C. Roberts and G. D. Prestwich, Biomacromolecules, 2002, 3, 1304–1311 CrossRef CAS.
- T. Schmitz, I. Bravo-Osuna, C. Vauthier, G. Ponchel, B. Loretz and A. Bernkop-Schnurch, Biomaterials, 2007, 28, 524–531 CrossRef CAS.
- S. Cerritelli, D. Velluto and J. A. Hubbell, Biomacromolecules, 2007, 8, 1966–1972 CrossRef CAS.
- S. Matsumoto, R. J. Christie, N. Nishiyama, K. Miyata, A. Ishii, M. Oba, H. Koyama, Y. Yamasaki and K. Kataoka, Biomacromolecules, 2009, 10, 119–127 CrossRef CAS.
- A. Klaikherd, C. Nagamani and S. Thayumanavan, J. Am. Chem. Soc., 2009, 131, 4830–4838 CrossRef CAS.
- E. D. Klug, J. Polym. Sci., Part C: Polym. Symp., 1971, 36, 491–508 Search PubMed.
- G. L. Ellman, Arch. Biochem. Biophys., 1959, 82, 70–77 CrossRef CAS.
- C. Hiemstra, L. J. van der Aa, Z. Y. Zhong, P. J. Dijkstra and J. Feijen, Biomacromolecules, 2007, 8, 1548–1556 CrossRef CAS.
- D. Bruneel and E. Schacht, Polymer, 1993, 34, 2633–2637 CrossRef CAS.
- J. C. Ramirez, M. Sanchezchaves and F. Arranz, Angew. Makromol. Chem., 1995, 225, 123–130 CrossRef CAS.
- T. Ooya and N. Yui, J. Controlled Release, 1999, 58, 251–269 CrossRef CAS.
- Y. Lee, H. Koo, G. W. Jin, H. J. Mo, M. Y. Cho, J. Y. Park, J. S. Choi and J. S. Park, Biomacromolecules, 2005, 6, 24–26 CrossRef CAS.
- J. P. Tam, C. R. Wu, W. Liu and J. W. Zhang, J. Am. Chem. Soc., 1991, 113, 6657–6662 CrossRef CAS.
- M. A. Wouters, S. W. Fan and N. L. Haworth, Antioxid. Redox Signaling, 2010, 12, 53–91 Search PubMed.
- H. J. Forman, M. Maiorino and F. Ursini, Biochemistry, 2010, 49, 835–842 CrossRef CAS.
- W. C. Hawkes and Z. Alkan, Biol. Trace Elem. Res., 2010, 134, 235–251 CrossRef CAS.
- M. A. Wouters, R. A. George and N. L. Haworth, Curr. Protein Pept. Sci., 2007, 8, 484–495 CrossRef CAS.
|
This journal is © The Royal Society of Chemistry 2011 |
Click here to see how this site uses Cookies. View our privacy policy here.