Effect of phosducin silencing on the photokinetic motile response of Blepharisma japonicum
Received
15th July 2010
, Accepted 27th September 2010
First published on 26th October 2010
Abstract
The coloured ciliate Blepharisma japonicum changes swimming velocity (positive photokinesis) and elongates its body in response to a prolonged illumination. We have recently proposed that alterations in the phosphorylation level of the ciliate phosducin (Pdc) may be involved in light-induced cell elongation, which in turn affects the interaction of βγ-dimer of G-proteins (Gβγ) with β-tubulin and subsequent cytoskeletal remodelling. The cellular mechanism that governs the photokinetic effect in this ciliate has not been elucidated. In the present study, we utilise real-time PCR to demonstrate that the levels of ciliate Pdc mRNA are significantly reduced in Pdc-RNAi-treated cells compared to cells fed with bacteria carrying the empty vector (control cells). Using western immunoblotting, we confirmed that these cells treated with Pdc-RNAi expressed a substantially lower level of the Pdc protein. The assay also revealed that in ciliates treated with Pdc-RNAi and exposed to light, the cytosolic level of Gβ (∼36 kDa) was reduced, whereas the level of Gβ localized to the membrane (∼32 kDa) was increased compared to control cells. In addition, behavioural analysis of the cells indicated a substantial reduction of photokinesis. The findings in this study provide additional characterization of the functional properties of the ciliate Pdc protein and we discuss a likely role for this phosphoprotein in the photokinetic phenomenon of the ciliate protist Blepharisma.
Introduction
Phosducin (Pdc) and the phosducin-like proteins (PhLPs) comprise a protein family that appears to have ancient origins, as its members are widely expressed in almost all eukaryotes, ranging from ciliates to plants and animals.1–3 These proteins can be divided into three main subgroups.2 Subgroup I includes the founding members of the family, Pdc and PhLP1, which have been shown to bind Gβγ subunits with high affinity.4–6Pdc, a soluble phosphoprotein of about 33 kDa, is highly abundant in the retina and pineal gland4,7 and has been most thoroughly investigated in the visual system. PhLP1 is expressed in most cell types and tissues,2,8,9 indicating a more general function. Subgroup II consists of two proteins that were recently discovered in humans, PhLP2A and PhLP2B.2,10,11 The ortholog of PhLP2 in Saccharomyces cerevisiae lacks the ability to bind Gβγ, but is essential for cell growth in this yeast,12 indicating a vital function that is independent of G-protein signalling. Subgroup III is composed of a single protein member, designated PhLP3.2 The yeast ortholog of PhLP3 can bind Gβγ poorly,13 but mutants of Saccharomyces and Dictyostelium lacking PhLP32 display no obvious phenotype. Based on the current literature, it appears that the members of the Pdc gene family play highly diverse physiological roles with no single unifying cellular function.
Proteins displaying high homology to Pdc and PhLP are also found in the light-sensitive ciliate protozoan, Blepharisma japonicum.14 As in other organisms, the ciliate Pdc (28 kDa) is dephosphorylated in a light-dependent manner, while the identified PhLP homologue (40 kDa) exhibits no detectable alteration in phosphorylation status upon illumination. Phosphorylation of Pdc in this ciliate is controlled by cyclic nucleotide dependent kinases, Ca2+ and calmodulin. In cells exposed to light, dephosphorylation of Pdc occurs as a result of the activation of protein phosphatases.15,16 The light-dependent changes in the levels of Pdc phosphorylation in Blepharisma coincide with cell body elongation as well as with a distinct increase in swimming velocity (positive photokinesis) when lasting light is applied.15,17–19 Short light stimuli (stepwise increase in light intensity) elicit in the protozoan a motile photophobic response (transient reversal of the direction of cilia beats).15,17 Our recent study showed that the modification of Blepharisma body length during cellular illumination may be due to changes in Pdc dephosphorylation.20 The Pdc-mediated behavioural responses initiated by light-activated G-proteins may include the functional coupling of G-protein subunits and tubulin, which subsequently controls microtubule assembly and stability.20,21 Because both photokinesis and cellular elongation occur under the same light conditions in our model, it would be of great interest to examine whether similar light-dependent molecular processes are responsible. To estimate the potential role of Pdc in the photokinetic motile responses in Blepharisma, RNAi method was applied to produce partial silencing of cell Pdc expression and the levels of Pdc mRNA, Pdc and Gβ were determined using appropriate experimental assays. In addition, a phenotypic analysis was performed to examine the patterns of the motile photobehaviour (photophobic and photokinetic responses) in control and RNAi-treated cells.
Results
Inhibition of Pdc expression with RNAi
To investigate a possible role for ciliate Pdc in the light responses of Blepharisma, we used RNAi to knock-down the expression of Pdc, which involved feeding the ciliates double-stranded RNA-producing bacteria to selectively eliminate expression of the target gene. Real-time PCR (RT-PCR) analysis showed that levels of Pdc mRNA were reduced in RNAi-treated cells to 16% of the control cells (ciliates fed with bacteria carrying empty vector) (Fig. 1). Western blot analyses of Pdc protein levels in control and RNAi-treated cells confirmed the RT-PCR data (Fig. 2B, C). Levels of Pdc protein in ciliates treated with Pdc RNAi were decreased to about 30% of those in control cells (Fig. 2B, C). Under these experimental conditions the levels of tubulin in control or treated cells did not change. Recently, it was reported that phosducin-like proteins PhLP2 and PhLP3 may control activity of the chaperonin CCT, which is responsible for folding various proteins, including tubulin.13,22
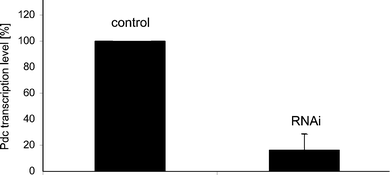 |
| Fig. 1 Changes in the levels of Pdc mRNA in RNAi-treated Blepharisma as determined by real-time PCR. Cells were fed with bacteria containing the Pdc-RNAi construct or the empty RNAi vector. The levels of Pdc transcript in cells fed with bacteria carrying the empty vector (control cells) were set as 100%. Bars represent mean values ± S.E.M. of at least three experiments. | |
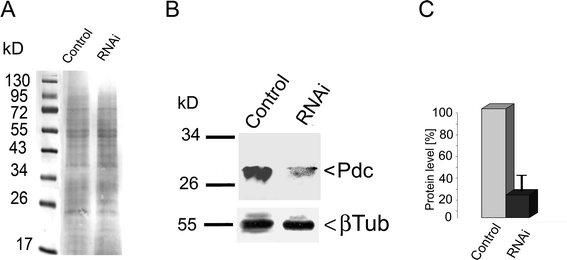 |
| Fig. 2 Alterations in Pdc expression in Blepharisma with RNAi-silenced gene as determined by western immunoblotting of whole-cell lysates. The result is representative of three independent experiments. A – the Ponceau S stained proteins were monitored as a loading control; B – immunoblot analysis with antiserum raised against Pdc detected a protein of 28 kDa in control cells, which almost disappeared following treatment of cells with siRNA targeted to Pdc; C – relative changes in Pdc levels estimated by a comparison to the constant level of tubulin expression in the tested cells. The levels of Pdc in control cells were set as 100%. Bars represent mean values ± S.E.M. of at least three experiments. | |
Effect of knock-down Pdc on level and localization of Gβγ
Our previous results revealed that Blepharisma, like other cells,23,24 contained two different pools of Gβγ. One existed in a membrane-bound fraction (32 kDa), and the other was dispersed throughout the cytoplasm (36 kDa).3 During cell illumination, the amount of Gβγ in the cytoplasm (36 kDa) was shown to increase. To examine the influence of Pdc-RNAi on the expression levels and localization of the Gβ subunit in cells under light illumination, we performed immunoblot analysis of the protein in RNAi-treated and control cells. This analysis demonstrated that the levels of cytoplasmic Gβ were reduced by about 40% in Pdc knock-down cells when compared to control cells (Fig. 3B, C). In contrast, levels of membrane-localized Gβ (32 kDa) were much higher in cells with reduced Pdc expression than in control cells. In cells adapted to darkness Pdc-RNAi silencing procedure alone did not affect noticeably both the levels of Gβγ and localization of these proteins (Fig. 3B, C).
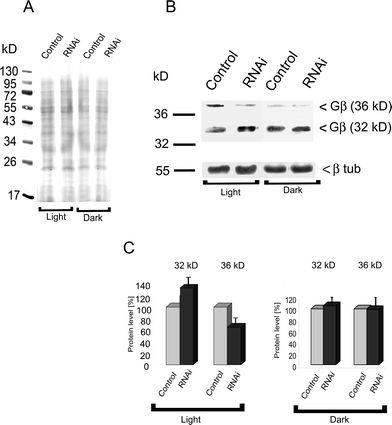 |
| Fig. 3 Expression of Gβ in control cells and in Blepharisma ciliates treated with siRNA targeted to Pdc as determined by western immunoblotting. The result is representative of three independent experiments. A – the Ponceau S stained proteins were monitored as a loading control; B – an antibody against Gβ detected two protein bands in whole-cell lysates, a 32 kDa membrane bound protein and a 36 kDa cytoplasmic protein in dark-adapted and illuminated cells; C – densitometric analysis of distribution of the Gβ amount in the cytoplasmic and membrane fractions. The level of Gβ in control cells was set at 100%. Bars represent mean values ± S.E.M. of three experiments. | |
Phenotypic effects of reduced Pdc expression
To test whether reduced Pdc expression affected ciliate photobehaviour, Blepharisma control cells and treated with Pdc RNAi were analyzed using dark-field and low-magnification photography. This method has been used successfully in previous studies to monitor ciliate responses to light stimulation.25,26 In darkness, ciliates treated with RNAi and also from normal cultures behaved similarly, i.e. they did not swim or moved very slowly. By applying light, cells showed the typical photophobic responses, the pattern of movement for both control and RNAi-treated cells was also quite similar (see inserts in Fig. 5). However, when cells with lower levels of Pdc were exposed to prolonged illumination, they exhibited significantly diminished swimming velocity in comparison with untreated ciliates, reflective of reduced positive photokinesis (Fig. 4A, B). As shown in Table 1, the estimated mean value of the steady-state forward swimming speed of treated with RNAi cells exposed to applied white light was lowered to 44,1% of that for control ciliates under the same light conditions. In addition, a smaller proportion of treated cells showed a decreased photokinetic effect (Fig. 4D) compared to control cells (Fig. 4C).
Table 1 The swimming velocity of control and RNAi-treated ciliates when exposed to lasting intense light. The data are given as the mean values ± S.E.M of measurements made for 13 to 23 cells
Experimental light condition (1.5 ×103 lux) |
Control cells |
RNAi-treated cells |
Steady-state swimming speed (μm s−1) |
385.7 ± 12.9 |
170.7 ± 9.1 |
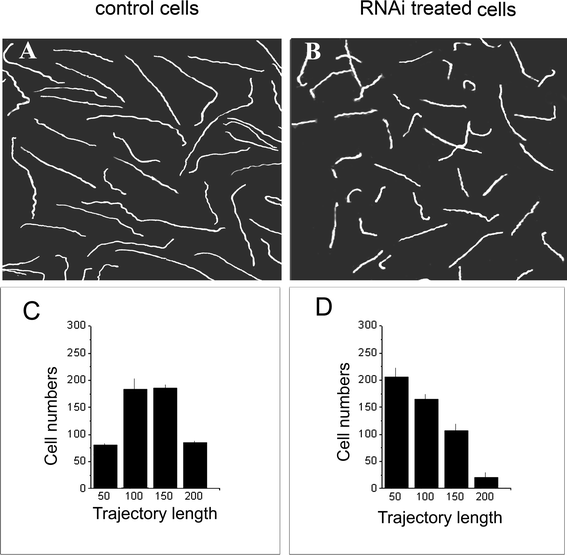 |
| Fig. 4 Effect of partial silencing of Pdc expression on photokinesis of Blepharismacells. Swimming patterns (A, B) and the distribution of trajectory lengths in arbitrary units (C, D) in cells adapted to light of 1.5 × 103 lux are shown. The images of the cell swimming trajectories were made using dark-field and low-magnification photography with an exposure of 15 s. A, C – control cells; B, D – cells treated with RNAi targeted to Pdc. Bars in C and D represent mean values ± S.E.M of three experiments. | |
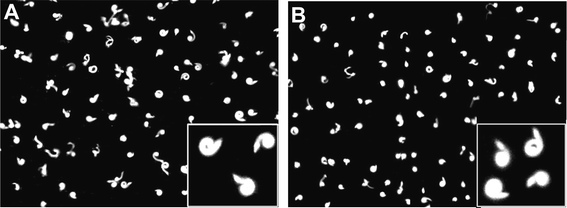 |
| Fig. 5 Photophobic behaviour of Blepharismacells to a sudden increase of light intensity. The pictures of control cells (A) and cells treated with RNAi (B) were taken by 3 s exposure under dark-field conditions. | |
Discussion
It was initially proposed that members of the phosducin (Pdc) gene family down-regulate G-protein signalling by binding to Gβγ and subsequently inhibiting the reassembly of G-proteins.1 However, recently it was reported that Pdc in photoreceptor cells could assist in the important process of shutting down rod responsiveness during prolonged exposure to high intensity light.27 In addition, studies on PhLP1, a close homolog of Pdc (65% homology), have indicated that this protein is a co-chaperone in the folding and assembly of Gβγ.28
As previously reported, the Pdc gene identified in Blepharisma belongs to subgroup I of the Pdc family14 and is similar to the phosphoprotein discovered in photoreceptor cells. Recently, we also presented evidence that Pdc and Gβγ interact in Blepharismacells exposed to light, and this interaction is dependent on the phosphorylation status of Pdc.3 The formation of the light-dependent Pdc-Gβγ complex is followed by its translocation to the cytoplasm, however, in dark-adapted ciliates, the Gβγ protein is mainly localized in the membrane fraction. Cytoplasmic Gβγ is characterized by reduced electrophoretic mobility and is recognized as a 36 kDa polypeptide, whereas the membrane-bound form migrates as a protein of 32 kDa. In the present study, we have found that in ciliates with reduced Pdc expression (Fig. 1, Fig. 2B), the cytosolic Gβγ (36 kDa) fraction was significantly diminished (Fig. 3B). However, the pool of membrane-bound Gβγ (32 kDa) was markedly elevated (Fig. 3B). These observations clearly indicate that reducing the expression of Pdc in Blepharisma may decrease the ability of Gβγ to move within the illuminated cells. In photoreceptor cells of higher organisms, similar phenomena were observed, and it was shown that the association of the Gβγ dimer and the photoreceptor membrane is due to membrane penetration by a farnesyl group attached to Gγ.29 Conversely, binding of Pdc to Gβγ causes the dissociation of Gβγ from the membrane.30
Because formation of the Pdc-Gβγ complex in Blepharisma is light-dependent,3,14 the lower levels of cytoplasmic Gβγ in the RNAi-treated cells may influence photobehaviour in this ciliate, such as photophobic or photokinetic motile responses. Indeed, in comparison to control cells (Fig. 4A, C), the photobehaviour pattern31 in ciliates with reduced Pdc expression displayed a markedly lower photokinetic effect when exposed to prolonged light (Fig. 4B, D). This difference was evident from both the shorter swimming trajectories and the lower proportion of cells demonstrating distinct positive photokinesis under the applied experimental conditions. No changes were observed in the pattern of photophobic responses between RNAi-treated and control cells (Fig. 5).
The photoreceptor cells characterized by the dark- and light-adapted response properties in knock-down Pdc in rod photoreceptors were found to be notably less sensitive to light than wild-type rods. This reduced amplification correlated with a 36% reduction in the expression level of the βγ-subunit of the G-protein (transducin). However, commonly studied forms of light adaptation were normal in the absence of Pdc. These findings suggest that Pdc does not dynamically regulate the availability of transducin and therefore does not control the light sensitivity of the rods. It is more likely that the role of Pdc in photoreceptor physiology is geared towards longer-term regulatory functions rather than short-term signalling.32 This latter possibility might explain why observed reduction in the level of Pdc in Blepharismacells did not produce any noticeable changes in the motile photophobic response to light (short-term signalling), however the changes were clearly detected in photokinesis (long-term signalling).
Materials and methods
Cell culture
Cells of Blepharisma japonicum33 were grown in semi-darkness at room temperature in culture medium (Pringsheim solution) containing 0.5 mM CaCl2, 1.0 mM MgSO4, 1.0 mM NaNO3, 0.1 mM NaH2PO4 and 1.0 mM Tris-HCl at a pH of approximately 7.1.34 The ciliates were fed with bacteria which grow around a few wheat grains added to the culture medium. For experiments, cells were collected by low speed centrifugation, washed in fresh culture medium with no nutritional components and then transferred to the fresh culture medium.
Total RNA isolation
Total RNA was isolated from 300 mL samples of Blepharismacell culture (∼100 cells mL−1) using TRI Reagent (Mol. Res. Center Inc., USA) according to the manufacturer's instructions.
cDNA synthesis and cloning
The synthesis and cloning of cDNAs were performed using the Universal RiboClone cDNA Synthesis System (Promega, Germany) according to the manufacturer's instructions. cDNA fragments were amplified by PCR using oligonucleotide primers (Sigma-Genosis, Germany) designed against the Blepharisma Pdc sequence (GenBank No. EF 198414): 5′-CATATCTGCAGACCGGCGTTAAGG-3′ and 5′-GCACTAAGCTTAACGAAGTTGCCTATC-3′). The PCR analysis was performed in a PTC-100 microcycler (MJ Research, USA), and it started with an initial denaturation period of 7 min at 94 °C, which was followed by 35 cycles of 1 min at 94 °C, 1 min at 62 °C and 1 min at 72 °C, with final extension period of 10 min at 72 °C. The amplified products were analyzed by gel electrophoresis and cDNA fragments were purified from gels using a NucleoSpin kit (Macherey-Nagel, Germany) according to the manufacturer's instructions. The purified Pdc (380 bp) cDNA fragments were partially sequenced (GATC, Konstanz, Germany) to confirm their identity, and they were then cloned into the vector L4440.35,36
RNAi
gene silencing
RNAi experiments were performed as previously described36 using the double-T7-promoter vector L4440 and the RNase III-deficient feeding strain of E. coli, HT115 (DE3), which contained an isopropyl β-D-1-thiogalactopyranoside (IPTG)-inducible T7 polymerase. Genomic fragments of Pdc (400 base pairs obtained by PCR were cloned into the L4440 vector between the double T7 promoter and transformed into HT115 (DE3). After selection, one plasmid-containing HT115 colony was inoculated into LB medium with 50 μg mL−1ampicillin (LBA) and grown overnight. Next, the culture was used to inoculate LBA (1/100 v/v), and it was grown until the culture reached an OD of about 0.4 at 600 nm. Addition of IPTG to a final concentration of 0.4 mM induced dsRNA expression for 3 h. After centrifuging the culture, the bacterial pellet was taken up in sterile cerophyl culture medium. This feeding stock solution for gene silencing experiments was used at a 1/10 (v/v) dilution.
Blepharisma
cells used for the RNAi experiments were taken from fresh culture medium, washed twice in sterile Pringsheim buffer and starved for at least 2 h in Pringsheim buffer at room temperature. Next, samples of cells (100 cells mL−1) were added to the appropriate feeding solution and cultured at 25 °C. They were then transferred 10 times every 24 h to freshly prepared feeding solutions. Finally, suspensions of control cells and RNAi-treated cells were harvested, washed in fresh culture medium and divided into three parts for the real-time PCR, western immunoblot and phenotype analyses.
Real-time PCR
The expression of Pdc RNA in cells was estimated using quantitative RT-PCR method. Each reaction was composed of cDNA template DynamoHS SYBR Green qPCR mixture (Finnzyme, Finland) and the forward and reverse primers (Sigma-Genosis, Germany) specific for the Blepharisma Pdc (5′-ATTGGGCTGCCTTGCTAT-3′ and 5′-CTTGTCTATAACTTA-3′) gene. The PCR analysis was performed using a Quantica real-time nucleic acid detection system (Techne, London, UK). The thermal conditions for RT-PCR were 95 °C for 15 min denaturation followed by 55 cycles of 20 s at 94 °C, 20 s at 61 °C and 20 s at 72 °C All reactions were performed in triplicate. Quantification analysis was performed by the comparative CT method, mathematically transforming the threshold cycle (CT) into the relative expression levels of genes.20,37 Data were analyzed using QPCR software (Rotor-Gene) and Microsoft Excel 2003.
Protein
electrophoresis was carried out according to a standard protocol.38 The protein concentration in each tested cell sample was calculated using BSA as a standard.39 Equal amounts of proteins were loaded into the 10% SDS-polyacrylamide gels, which were resolved using a Hoefer Electrophoresis System (Amersham Pharm. Biotechnol., Little Chalfont, UK). The separated proteins were transferred to a nitrocellulose membrane (Bio-Rad, USA) in transfer buffer40 using a Hoefer system (model TE 22; Amersham Pharm. Biotechnol., Little Chalfont, UK). After blocking in 2% BSA in TBS for 2 h blots were incubated overnight at 4 °C with primary antibodies raised against Pdc or Gβ (Santa Cruz Biotechnol., USA) diluted 1
:
1000 in TBS-BSA. After several washes the blots were incubated for 1 h at room temperature with anti-rabbit IgG-HRP conjugate secondary antibody diluted at 1
:
10000 (Calbiochem, USA) or anti-goat IgG-HRP conjugate secondary antibody diluted at 1
:
5000 (Rockland Inc, USA) in TBS-Tween-BSA. Specific antibody binding was visualized using a Pierce ECL detection system (Thermo Fisher Scientific Inc, USA). Equal protein loadings were confirmed by Ponceau S staining of the membranes (Fig. 2A, 3A) and by tubulin immunolabelling with an anti-β-tubulin antibody (Fig. 2B, 3B) (Sigma, Germany). The intensities of immunoreactive protein bands were quantified using the InGenius system (Syngene BioImaging, UK).
Phenotypic assay
Phenotypic analysis was performed by assessing Blepharismacell motility under different experimental conditions using dark-field and low-magnification photography.41,40 The samples of both control (empty plasmid-treated) and RNAi-treated cells that had been kept in darkness were placed in a thin layer of Pringsheim solution in a Petri dish and then adapted for 10 min to standard light intensity (1.5 ×103 lux). Occurrence of photophobic responses was observed at the onset of light stimulation while the increased swimming velocity (photokinesis) was measured after a 10 min adaptation to applied light intensity, i.e. after the saturation of speeding up cell reaction was reached.43 The saturation levels of the cell velocity after 10 min light application were confirmed by estimation of the same cell speed resulting from cell exposition to light for longer time (15–25 min). The pattern of ciliate motile behaviour under different experimental conditions was recorded with a digital camera (Olympus, USA) and the cell movement parameters (swimming trajectory length or velocity) were estimated using the ImageJ 1.37a software (Wayne Rasband, Natl. Inst. Health, USA; http://rsb.info.nih.gov/ij/).
Acknowledgements
This study was supported by statutory funding for the Nencki Institute of Experimental Biology in Warsaw and the Polish Network for Visualization of Biomedical Events (BioImaging). We thank Bozena Groszynska for help in preparation of experiments.
References
- R. Schulz, The pharmacology of phosducin, Pharmacol. Res., 2001, 43, 1–10 CrossRef CAS.
- M. Blaauw, J. C. Knol, A. Kortholt, J. Roelofs, A. Ruchira, M. Posma, A. J. W. G. Visser and P. J. M. Van Haaster, Phosducin-like proteins in Dictyostelium discoideum: implications for the phosducin family of proteins, EMBO J., 2003, 22, 5047–5057 CrossRef CAS.
- K. Sobierajska, H. Fabczak and S. Fabczak, Phosducin interacts with the G-protein beta-gamma-dimer of ciliate protozoan Blepharisma japonicum upon illumination, J. Exp. Biol., 2007, 210, 4213–4223 CrossRef CAS.
- R. H. Lee, B. S. Liebermann and R. N. Lolley, A novel complex from bovine visual cells of a 33 kDa phosphoprotein with β- and γ-transducin: purification and subunit structure, Biochemistry, 1987, 26, 3983–3990 CrossRef CAS.
- C. Thibault, M. V. Sganga and M. F. Miles, Interaction of phosducin-like protein with G-protein beta-gamma subunits, J. Biol. Chem., 1997, 272, 12253–12256 CrossRef CAS.
- J. R. Savage, J. N. McLaughlin, N. P. Skiba, H. E. Hamm and B. M. Willardson, Functional roles of the two domains of phosducin and phosducin-like proteins, J. Biol. Chem., 2000, 275, 30399–30407 CrossRef CAS.
- J. A. Reig, L. Yu and D. C. Klein, Pineal transduction. Adrenergic-cyclic AMP-dependent phosphorylation of cytoplasmic 33-kDa protein (MEKA) which binds beta-gamma-complex of transducin, J. Biol. Chem., 1990, 265, 5816–5824 CAS.
- M. F. Miles, F. Barhite, S. Sganaga and M. Elliott, Phosducin-like protein: an ethanol-responsive potential modulator of guanine nucleotide-binding protein function, Proc. Natl. Acad. Sci. U. S. A., 1993, 90, 10831–10835 CrossRef CAS.
- S. Kasahara, P. Wang and D. L. Nuss, Identification of bdm-1, a gene involved in G- proteinβ-subunit function and α-subunit accumulation, Proc. Natl. Acad. Sci. U. S. A., 2000, 97, 412–417 CrossRef CAS.
- P. Lopez, R. Yaman, L. A. Lopez-Fernandez, F. Vidal, D. Puel, P. Clertant, F. Cuzin and M. Rassoulzadegan, A novel germ line-specific gene of the phosducin-like protein (PhLP) family. A meiotic function conserved from yeast to mice, J. Biol. Chem., 2003, 278, 1751–1757 CrossRef CAS.
- J. C. Wilkinson, B. W. Richter, A. S. Wilkinson, E. Burstein, J. M. Rumble, B. Balliu and S. C. Duckett, VIAF, a conserved inhibitor of apoptosis (IAP)-interacting factor that modulates caspase activation, J. Biol. Chem., 2004, 279, 51091–51099 CrossRef CAS.
- P. L. Flanary, P. R. DiBello, P. Estrada and H. G. Dohlman, Functional analysis Plp1 and Plp2, two homologues of phosducin in yeast, J. Biol. Chem., 2000, 275, 18462–18469 CrossRef CAS.
- P. C. Stirling, M. Srayko, K. S. Takhar, A. Pozniakovsky, A. A. Hyman and M. Leroux, Functional interaction between phosducin-like protein 2 and cytosolic chaperonin is essential for cytoskeletal protein function and cell cycle progression, Mol. Biol. Cell, 2007, 18, 2336–2345 CrossRef CAS.
- H. Fabczak, K. Sobierajska and S. Fabczak, Identification of possible phosducins in the ciliate Blepharisma japonicum, Protist, 2004, 155, 181–192 CrossRef CAS.
- S. Fabczak, H. Fabczak, N. Tao and P.-S. Song, Photosensory transduction in ciliates. III. The, temporal relation between membrane potentials and photomotile responses in Blepharisma japonicum, Photochem. Photobiol., 1993, 57, 872–876 CrossRef.
- K. Sobierajska, H. Fabczak and S. Fabczak, Alterations of ciliate phosducin phosphorylation in Blepharisma japonicum cells, J. Photochem. Photobiol., B, 2005, 79, 135–143 CrossRef CAS.
- T. Matsuoka, Negative phototaxis in Blepharisma japonicum, J. Protozool., 1983, 30, 409–414 Search PubMed.
- T. Matsuoka and Y. Shigenaka, Mechanism of cell elongation in Blepharisma japonicum, with special reference to the role of cytoplasmic microtubules, Cytobios, 1985, 42, 215–226 Search PubMed.
- M. Ishida, Y. Shigenaka and K. Taneda, Studies on the mechanism of cell elongation in Blepharisma japonicum. I. Physiological, mechanism how light stimulation evokes cell elongation, Eur. J. Protistol., 1989, 25, 182–186.
- K. Sobierajska, J. Głos, J. Dąborowska, J. Kucharska, C. Bregier, S. Fabczak and Hanna Fabczak, Visualization of the interaction between Gβγ and tubulin during light-induced cell elongation of Blepharisma japonicum, Photochem. Photobiol. Sci., 2010, 9, 1101–1110 RSC.
- S. Roychowdhury and M. M. Rasenick, Submembraneous microtubule cytoskeleton: regulation of microtubule assembly by heterotrimeric G-proteins, FEBS J., 2008, 275, 4654–4663 CrossRef CAS.
- P. C. Stirling, J. Cuéllar, G. A. Alfaro, F. El Khadali, C. T. Beh, J. M. Valpuesta, R. Melki and M. R. Leroux, PhLP3 modulates CCT-mediated actin and tubulin folding via ternary complexes with substrates, J. Biol. Chem., 2006, 281, 7012–7021 CrossRef CAS.
- F. Chen and R. H. Lee, Phosducin and betagamma-transducin interaction I: effects of post-translational modifications, Biochem. Biophys. Res. Commun., 1997, 233, 370–374 CrossRef CAS.
- T. Jin, N. Zhang, Y. Long, C. A. Parent and P. N. Devreotes, Localization of the G- protein beta-gamma complex in living cells during chemotaxis, Science, 2000, 287, 1034–1036 CrossRef CAS.
- H. Fabczak, Protozoa as a model system for studies of sensory light transduction: Mechanism of photophobic response in the ciliate Stentor and Blepharisma, Acta Protozool., 2000, 39, 171–181 CAS.
- M. Walerczyk, H. Fabczak and S. Fabczak, A videomicroscopic study of the effect of l-cis-diltiazem on Stentor coeruleus photobehavior, Photochem. Photobiol., 2003, 77, 339–342 CrossRef CAS.
- B. M. Willardson and A. C. Howlett, Function of phosducin-like proteins in G- protein signaling and chaperone-assisted protein folding, Cell. Signalling, 2007, 19, 2417–2427 CrossRef CAS.
- G. L. Lukov, C. M. Baker, P. J. Ludtke, T. Hu, M. D. Carter, R. A. Hackett, C. D. Thulin and B. M. Willardson, Mechanism of assembly of G-protein beta-gamma subunits by protein kinase CK2-phosphorylated phosducin-like proteins and the cytosolic chaperonin complex., J. Biol. Chem., 2006, 281, 22261–22274 CrossRef CAS.
- D. Murray, S. Laughlin and B. Honig, The role of electrostatic interactions in the regulation of membrane association of G-protein beta-gamma heterodimers, J. Biol. Chem., 2001, 276, 45153–45159 CrossRef CAS.
- H. Kassai, A. Aiba, K. Nakao, K. Nakamura, M. Katsuki, W. H. Xiong, K. W. Yau, H. Imai, Y. Shichida, Y. Satomi, T. Takao, T. Okano and Y. Fukada, Farnesylation of retinal transducin underlies its translocation during light adaptation, Neuron, 2005, 47, 529–539 CrossRef CAS.
- B. Dhien, M. E. Feinleib, H. Haupt, F. Hildebrandt, F. Lenci and W. Nultch, Terminology of behavioral response of motile organisms, Photochem. Photobiol., 1977, 26, 559–560.
- C. M. Krispel, M. Sokolov, Y. M. Chen, H. Song, R. Herrmann, V. Y. Arshavsky and M. E. Burns, Phosducin regulates the expression of transducin beta gamma subunits in rod photoreceptors and does not contribute to phototransduction adaptation, J. Gen. Physiol., 2007, 130, 303–312 CrossRef CAS.
- S. Suzuki, Taxonomic studies on Blepharisma undulans with special reference to the macronuclear variation, J. Sci. Hiroshima Univ. Div. 1, Ser. B., 1954, 15, 205–220 Search PubMed.
- H. Fabczak, Contribution of the phosphoinositide-dependent signal pathway to photomotility in Blepharisma, J. Photochem. Photobiol., B, 2000, 55, 120–127 CrossRef CAS.
- L. Timmons and A. Fire, Specific interference by ingested dsRNA, Nature, 1998, 395, 854 CrossRef CAS.
- A. Galvani and L. Sperling, RNA interference by feeding in Paramecium, Trends Genet., 2002, 18, 11–12 CrossRef CAS.
- K. J. Livak and T. D. Schmittgen, Analysis of relative gene expression data using real-time quantitative PCR and the 2(-Delta Delta C(T)), Methods, 2001, 25, 402–408 CrossRef CAS.
- U. K. Laemmli, Cleavage of structural proteins during the assembly of the head of bacteriophage T4, Nature, 1979, 277, 680–685.
- M. M. Bradford, A rapid and sensitive method for the quantization of microgram quantities of protein utilizing the principle of protein-dye binding, Anal. Biochem., 1976, 72, 248–254 CrossRef CAS.
- H. Towbin, T. Staehelin and J. Gordon, Electrophoretic transfer of proteins from polyacrylamide gels to nitrocellulose sheet: Procedure and some applications, Proc. Natl. Acad. Sci. U. S. A., 1979, 76, 4350–4354 CAS.
- M. L. Ferguson, Photographic technique for quantitative physiological studies of Paramecium and other motile cells, Physiol. Zool., 1957, 30, 208–215 Search PubMed.
- S. Dryl, Photographic registration of movement of protozoa, Bull. Acad. Pol. Sci., II Ser. Sci. Biol., 1958, 6, 429–430 Search PubMed.
- M. Kraml and W. Marwan, Photomovement responses of the heterotrichous ciliate Blepharisma japonicum., Photochem. Photobiol., 1983, 37, 313–319 CrossRef CAS.
|
This journal is © The Royal Society of Chemistry and Owner Societies 2011 |