DOI:
10.1039/C0NR00644K
(Paper)
Nanoscale, 2011,
3, 654-660
Facile synthesis of highly uniform Mn/Co-codoped ZnO nanowires: Optical, electrical, and magnetic properties
Received
1st September 2010
, Accepted 14th October 2010
First published on 26th November 2010
Abstract
In this article, Co/Mn-codoped ZnO nanowires (NWs) were successfully synthesized on a silicon substrate by the thermal evaporation method with Au catalyst. The X-ray diffraction pattern indicated that the Co/Mn-codoped ZnO NWs are a hexagonal wurtzite structure without a second phase, and energy dispersive X-ray spectroscopy revealed that the Co and Mn ions were introduced into the ZnO NWs with the content of ∼0.8 at% and ∼1.2 at%, respectively. Photoluminescence spectra and Raman spectra showed that the Co/Mn were doped into the NWs and resulted in the shift of the near-band-edge emission. Moreover, the novel Raman peak at 519.3 cm−1 has suggested that the two kinds of cations via doping could affect the local polarizability. Compared with the undoped ZnO NW, the electrical measurement showed that the Co/Mn-codoping enhanced the conductivity by an order of magnitude due to the presence of Co, Mn cations. The electron mobility and carrier concentration of a fabricated field effect transistor (FET) device is 679 cm2 V−1s−1 and 2 × 1018 cm−3, respectively. Furthermore, the M–H curve demonstrated that the Co/Mn-codoped ZnO NWs have obvious ferromagnetic characteristics at room temperature. Our study enhances the understanding of the novel performances of transition-metal codoped ZnO NWs and also provides a potential way to fabricate optoelectronic devices.
1. Introduction
As an n-type semiconductor with the wide band gap (3.37 eV) and large exciton binding energy (60 meV), ZnO has attracted a great deal of attention in science and technology.1 One-dimensional ZnO nanostructures such as nanowires (NWs) and nanobelts are considered important building blocks for fabricating various nanodevices, including nanogenerators,2 field effect transistors (FETs),3 Schottky diodes,4 acoustic resonators,5 and gas sensors.6 Different methods have been reported for the synthesis of well-aligned nanorod,7nanowire,8,9nanotube,10,11 nanobelt,12,13 and nanotetrapod.14 The thermal evaporation method is preferred due to its convenience and low cost. In this process, doping is a very important modification technology for the oxide semiconductor, which can change the optical, electrical and magnetic properties of the semiconductor materials. In particular, a key requirement for many applications of optoelectronic devices is the doping of ZnO with various elements for enhancing and controlling its electrical and optical performance.
Recently, transition-metal (TM)-doped ZnO has been extensively investigated as a promising dilute magnetic semiconductor (DMS) for implementing spintronic device concepts. The ferromagnetism (FM) measurements at various temperatures have been reported in Co-, Ni-, Mn-, and Fe-doped ZnO thin films15–19 as well as nanostructures,20–23 and room temperature FM has been experimentally demonstrated in Co or Mn-doped ZnO24 thin films or nanostructures.25 For example, Djerdj et al.26 demonstrated that the Co-doped ZnO powders are indeed ferromagnets with a Curie temperature above room temperature (RT) and the Mn-doped samples show antiferromagnetic correlations with a possible transition to an antiferromagnetic ground state below TN = 10 K. Coey et al.27 proposed that the ferromagnetic exchange is mediated by shallow donor electrons that form bound magnetic polarons that overlap to create a spin-split impurity band. However, a doped ZnO nanostructure by a controllable method is difficult to prepare.28,29 Most of the researchers reported that they had difficulties in controlling the structure and the composition of the doped ZnO NWs or nanorod arrays. Moreover, there is a great deal of controversy about whether TM-doped ZnO has room temperature FM, and the research on the electrical properties of TM-doped ZnO arrays has scarcely been reported, which could limit the applications of ZnO arrays. In addition, the majority of magneto transport studies on DMS materials have been reported in either the bulk or thin film forms. There are far fewer reports of spin transport in one dimensional DMS materials, such as nanowires.30
In TM-doped ZnO nanostructures, some work have concentrated on the growth of Co or Mn respectively doped ZnO nanostructures and characterization of their structural, magnetic, and electrical properties.23–27,31 However, little attention was paid to (Mn,Co)-codoped ZnO NWs system. Meanwhile, several codoped ZnO film materials have been reported, with the expectation that codoping can lead to remarkable changes in the properties of DMS. For instance, focusing on the magnetic properties of (Co,Mn) codoped ZnO samples, Yan et al.15 reported that Zn0.7(Mn0.15Co0.15)O thin films synthesized by pulsed laser deposition exhibited RT (290 K) FM with a saturated magnetic moment of 0.19μB/(0.5Co + 0.5Mn). Gu et al.32 also observed FM behavior with a coercivity of about 90 Oe and a saturation moment of 0.11μB/(0.3Mn2+ + 0.7Co2+) at 300 K in (Mn0.03Co0.07)Zn0.90O films prepared by radiofrequency magnetron cosputtering. To date, there has been no Co/Mn-codoped ZnO NWs in the literature due to the nonmagnetic host ions being difficult to partially substitute by the magnetic ions. So it is valuable to research the optical, electrical, and magnetic properties of one dimensional Co/Mn-codoped ZnO nanomaterials.
In this letter, we used a simple thermal evaporation method to synthesize Co/Mn-codoped ZnO NWs at low temperature. The optical, electrical and magnetic properties of the codoped NWs have been studied in detail. These results suggest that the Co/Mn-codoped ZnO NWs can be used for visible violet light emitters and optoelectronic devices.
2. Experimental section
Co/Mn-Codoped ZnO NWs were synthesized by the following procedure. First, high purity Zn powders (99.99%), MnCl2·4H2O powders (99.99%), CoCl2·6H2O powders (99.99%) and graphite powders were mixed with mass ratio of 10
:
1
:
1
:
1 as the source materials. The compounds were laid in an alumina boat which was placed at the center of a horizontal tube furnace. A silicon substrate plated by a 20 nm Au layer was placed above the boat. Then, the tube furnace was heated to 500 °C with a constant argon flow of 50 sccm (standard cubic centimetres per minute). After 180 min, a thin layer was deposited on the silicon wafer. Compared to pure ZnO NWs, the color of the as-received sample is yellow. A similar method was used in synthesising the pure ZnO NWs in the previous work.33
The morphologies and structures of the synthesized product were characterized using field emission scanning electron microscopy (FESEM) (Zeiss, SUPRA-55, Germany), X-ray powder diffraction (XRD) (Rigaku DMAX-RB, Japan), and transmission electron microscopy (TEM) (JEOL-2010, Japan).
Raman scattering experiments and photoluminescence (PL) measurements were performed at room temperature using a Raman spectroscope meter (Jobin-Yvon, HR800, France) with the 532 nm line of an Ar+ laser and an excitation wavelength of 325 nm of He–Cd laser, respectively. The electrical characteristics of individual ZnO NW were measured by nano-manipulation (Zyvex S100, American), measurement systems (Keithley 4200-SCS, American) and scanning electron microscopy (SEM, JEOL-6490, Japan). The magnetization behavior of the NWs was investigated using superconducting quantum interference device (SQUID) (Quantum Design, American).
3. Results and discussion
Morphologies and characterization
The as-deposited products were first examined by FESEM. Fig. 1a shows a low-magnification SEM image of Co/Mn-codoped ZnO NWs with uniform diameters in the range of 50–200 nm and lengths up to several tens of microns. From Fig. 1a we can see that the dense and wire-like ZnO NWs were formed on a large and the film thickness is about 1–2 μm. Furthermore, the high-magnification image of Co/Mn-codoped ZnO NWs (inset of Fig. 1a) indicated that these ZnO have high crystal quality and high aspect ratio. HRTEM image for a single NW is shown in Fig. 1b. It was taken from the edge of an individual NW and reveal their single crystalline structure. Although obvious clustering/phase separation can be excluded from the TEM imaging, it is still necessary to determine the sites of the dopants in the ZnO lattice. If the transition metal ions are to occupy the interstitial sites, their possibly non-uniform distribution could lead to locally high concentrations of the ions in the ZnO (although chemical bonding among the transition metal ions and thus a second phase in the format of metal clusters has not formed).34 The XRD pattern taken from the Co/Mn-codoped ZnO NWs is shown in Fig. 1c. The NWs have the wurtzite structure and no impurities were found. The doping causes significant change in lattice constants, resulting in a measurable shift in the (002) peak as seen in the insets in Fig. 1c, corresponding to an expansion in lattice constants by Δc/c = 1.0% after doping. The local chemical compositions of the samples were characterized by energy dispersive X-ray spectroscopy (EDS) in SEM. Fig. 1d shows the existence of zinc, oxygen, manganese and cobalt in the ZnO NWs. From the profile of the Co/Mn signal, the Co/Mn atoms were doped into the volume without significant surface segregation. In the samples, EDS was performed at several microns of length in the same NWs, resulting in the identification of the same chemical compositions, which suggests a fairly uniform distribution of the dopant ions. No clustering of the cobalt or manganese was observed, and their concentrations were estimated at ∼0.8% and ∼1.2%, respectively. In order to check the homogeneity of the Co/Mn distribution within the whole sample, EDS spectra were also recorded at different points along the wire, and the result showed that Co/Mn concentration had a measurable variation less than 0.2%.
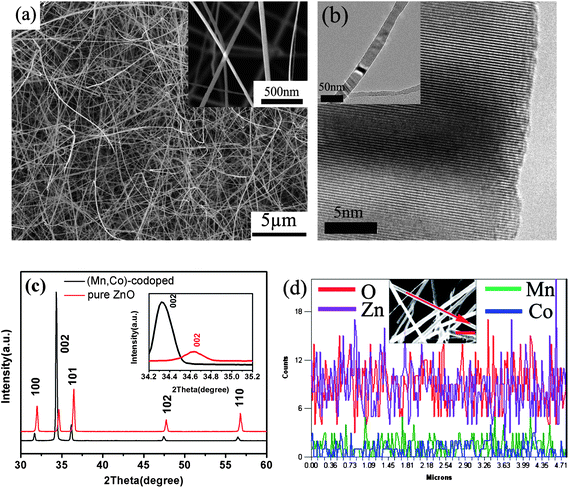 |
| Fig. 1 (a) The low-magnification SEM morphologies of Co/Mn-codoped ZnO NWs, inset: the high-magnification SEM morphologies, (b) HRTEM image of Co/Mn-doped ZnO NW (inset: TEM morphology of individual Co/Mn-codoped ZnO NW), (c) The XRD pattern taken from Co/Mn-codoped ZnO NWs, and (d) EDS line scan of chemical profile reveals a homogeneous distribution of Co and Mn across the ZnO NW. | |
Optical property
Photoluminescence spectra were recorded to get information of undoped and Co/Mn-codoped ZnO NWs at room-temperature (Fig. 2a). The excitation wavelength of PL was 325 nm using a He–Cd laser. All of them are composed of two emission bands: an intensive ultraviolet emission at ∼388 nm and a weak green emission at ∼550 nm were observed. One peak is the ultraviolet emission at ∼3.20 eV (λ = 387 nm) which is near-band-edge exciton-related emission, and the other one is the deep level emission. The UV emission peak with a shoulder peak at 388 nm is explained by a near-band edge emission, which exhibits red shift compared with the PL emission usually received from the undoped ZnO NWs35 and ZnO films.36 The energy gap narrowing down in Co/Mn-codoped ZnO may account for the small red shift of the near-band-edge emission. The deep level emission band has previously been attributed to several defects in the crystal structure such as O-vacancy (VO), Zn-vacancy (VZn), O-interstitial (Oi), Zn-interstitial (Zni), and extrinsic impurities. Recently, this deep level emission band had been identified and at least two different defect origins, VO and VZn, with different optical characteristics were claimed to contribute to this deep level emission band.37 The broad green band emission for the Co/Mn-codoped product could be explained in terms of the increased amount of doping created defects in the sample. However, a full understanding of the actual recombination mechanism of the defect-level emission band needs more experiments since their assignments are still under debate due to the complicated microscopic defects in ZnO.38 From the image shown in inset of Fig. 2a, the strong green light by emission of He–Cd laser has been observed.
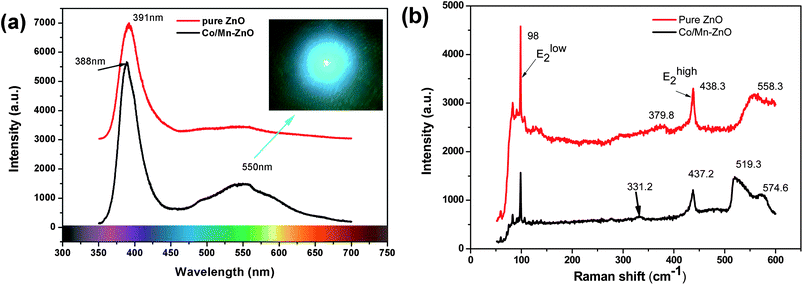 |
| Fig. 2 (a) The PL spectra of ZnO and Co/Mn-codoped ZnO NWs (inset: the image of the green optical pattern), and (b) the Raman shift of ZnO and Co/Mn-codoped ZnO NWs. | |
Raman scattering spectroscopy is an effective tool to investigate the nanoscale structure, secondary phase segregation, and the influence of impurity doping on the lattice vibrational properties of the host materials. Fig. 2b shows the Raman spectra of undoped and Co/Mn-codoped ZnO NWs, respectively. The positions of phonon vibrational frequencies and their assignments are summarized in Table 1 according to the assignments in the literature.38–40 The undoped ZnO nanorods exhibit four Raman-active peaks at 98, 379.8, 438 and 558 cm−1, and the Co/Mn-codoped NWs display five peaks at 98, 331.2, 437.2, 519.3 and 574.6, respectively. The two intensive peaks at 98 cm−1 and 438 cm−1 are indicated to be E2low and E2high, which are the characteristic bands of the wurtzite ZnO. The peaks at 574.6 cm−1 are known to be A1(LO), which is also the first-order optical mode of wurtzite ZnO. And the peak 331.2 cm−1 and 379.8 cm−1 are E2high–E2low and A1(TO), respectively. The peak at 519.3 cm−1 is a novel Raman peak, which suggested that the mixing of two different cations via doping could affect the local polarizability by charge distribution and result in at least one vibrational mode being strongly influenced. The broad band at 520–600 cm−1 is conjectured to be associated with intrinsic lattice defects and often arise by doping.41,42
Table 1 Phonon vibrational frequencies in undoped and doped ZnO nanowires and their assignments according to ref. 38–40
No. |
Frequency/cm−1 |
Assignments |
1 |
96 |
E2L |
2 |
330 |
E2H–E2L |
3 |
379 |
A1T |
4 |
435 |
E2H |
5 |
575 |
A1L or E1L; LO |
Electrical property
The doping element is likely to affect the transport property of the NWs. We have fabricated two-electrode bridged devices using individual NW, and their transport properties have been measured. Fig. 3a shows the SEM image of the device with metal tantalum (Ta) deposited at the contacts using E-beam lithography to reduce the contact resistance. From the SEM image we can see that a single Co/Mn-codoped ZnO NW was in contact with two Ta electrodes and the NW was laid on prefabricated electrodes by nano-manipulation. Fig. 3b gives the current–voltage (I–V) characteristics of a single NW based device. The I–V curve shows an Ohmic behavior of the NW, from the slope of which and the diameter and length of the NW measured from the SEM image, the conductivity of the undoped ZnO NWs and Co/Mn-codoped ZnO NWs is calculated to be ∼0.455 Ω−1 cm−1 and ∼4.43 Ω−1 cm−1, respectively. Through the doped Co/Mn cations, the conductivity of the ZnO NWs increased by an order of magnitude.
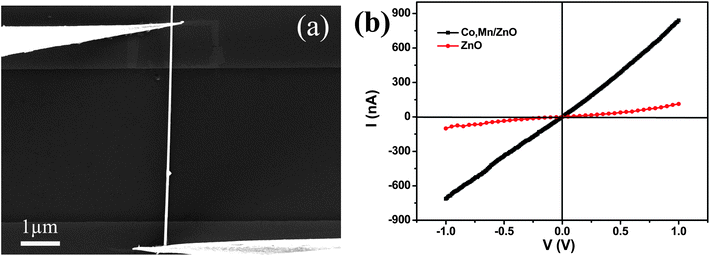 |
| Fig. 3 (a) SEM image of a single NW contacted with two Ta electrodes, and (b) I–V curve of a single Co/Mn-codoped ZnO NW (black) and pure ZnO NWs (red). | |
High electrical resistivity in a single undoped ZnO NW indicates the high quality of the crystalline structure with low oxygen deficiencies. This result has been further confirmed by our PL measurement performed on undoped ZnO NWs (Fig. 2a). In contrast, the I–V curve for a Co/Mn-codoped ZnO NW (Fig. 3b) shows a steep increase in conductance, indicating a great enhancement in conductivity by doping. The current of the Co/Mn-codoped NW changed from about −700 to 700 nA as the voltage V was varied from −1 to 1 V, and the resistivity drops to 0.225 Ω cm. Compared with the pure ZnO NW, whose current changed from about −100 to 100 nA as the voltage V was varied from −1 to 1 V, and whose resistivity was 2.198Ω cm, the resistivity of the Co/Mn-codoped ZnO NWs increased tenfold. For thin films, doping cations with a higher valence state than Zn (Zn2+), such as In, Ga, and Ni, into ZnO leads to an increase in the electrical conductivity. Referring to the pure ZnO NW, the doped Co/Mn enhances the conductivity probably via the presence of different valent Co, Mn cations. 43,44 Furthermore, the different ion state in the nanowires is a crucially important factor for the electrical transport results. Wang et al. successfully identified and positioned three Co dopant states in the host ZnO bands, and the electron extension degrees of the Co dopant states are assessed on the basis of the multiple-atom effect.45 So, the state of complex ion doping and some kinds of defects will affect the electrical performance.
The larger amount of research on ZnO NW FETs makes them attractive building blocks for functional electronic devices. In our experiment, the ZnO NW FET was characterized using a four-probe system (Zyvex S100 nanomanipulator stage) inside an SEM, and the schematic diagram is shown in the inset of Fig. 4b. Two independent manipulator probes were placed in contact with an individual NW. A DC gating voltage was applied on the probe which was on the middle of Co/Mn-doped ZnO NW (gate), and a sweeping voltage was applied between the both ends (source and drain, respectively). I–V curves of the individual Co/Mn-doped ZnO NW are displayed in Fig. 4a, and they show linear behavior within our measured voltage ranges, which suggests that the contacts to the NWs are Ohmic and that contact resistance is negligible. When the gating voltage (Vg) is fixed, the drain current (Id) increased linearly as voltage drain and source (Vds) increased. At the same voltage of Vds, the Id decreased with Vg from −1 V to 0 V. The Vg on the middle of a NW can offset the current in the source and drain, so that the device can function as a current switch.
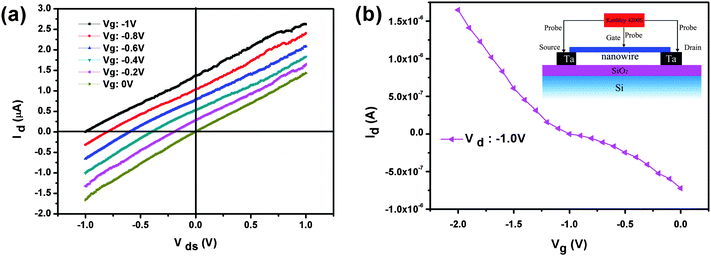 |
| Fig. 4 (a) Id–Vds curves obtained at various gate voltages at room temperature, and (b) Id–Vg curves obtained at various source–drain voltages; the inset shows a schematic diagram of ZnO NW FET. | |
The electron mobility is calculated from the transconductance, dG/dVg, and from the geometry of the NW. We estimate the electron mobility in our Co/Mn-codoped ZnO NWs to be on the order of 679 cm2 V−1s−1. The value is comparable with the reported higher mobilities of n-type ZnO materials. The carrier concentration is on the order of 2 × 1018 cm−3. This suggests that the Co/Mn dopants do not drastically decrease the mobility due to their similar ionic radii.46,47
Magnetism property
The magnetic properties of the Co/Mn-codoped ZnO NWs were investigated using a superconducting quantum interference device magnetometer (SQUID). Fig. 5a presents the M–H curves (field dependence of magnetization) of the measurement at 300 K and 5 K, respectively. The diamagnetic contribution from the sample holder has been subtracted from the data. Hysteresis loops with a coercive field HC ∼151 Oe (1 Oe = 79.57747 Am−1) and 255 Oe can be observed at 300 K and 5 K, respectively, showing obvious ferromagnetic characteristics. The saturation magnetization of Co/Mn-codoped ZnO NWs is about 0.635 emu g−1 and 1.375 emu g−1 at 300 K and 5 K, respectively. The Co/Mn-codoped ZnO NWs have obvious ferromagnetic characteristics at room temperature. Fig. 5b shows the temperature dependence of the magnetization (M–T) during warming up of the sample in a magnetic field of 1000 Oe after cooling without the magnetic field [zero-field cooling (ZFC)]. The contribution from the Si substrate was subtracted from the raw data. The strongly concave M–T behavior is often observed in the carrier localized regime, which is strikingly different from the usual Weiss mean-field prediction. The concave magnetization behavior can be understood in the scheme of polaron percolation. The driving force behind the ferromagnetic transition is the ordering of impurity spins around the localized carriers into magnetic polarons. At a certain temperature, it grows large enough so that the polarons percolate into an infinite cluster. However, since the percolating cluster does not contain a majority of the magnetic impurities and most impurity spins remain free even at a lower temperature, it is the uncoupled spins that give rise to the slow saturation of magnetization.48 On the other hand, the ZFC curve experiences a blocking phenomenon at ∼25 K, which reveals a transition from the ferromagnetic to the superparamagnetic state, with a blocking temperature TB. And the curve displays another transition from the superparamagnetic to the paramagnetic state, with a Curie temperature TC.
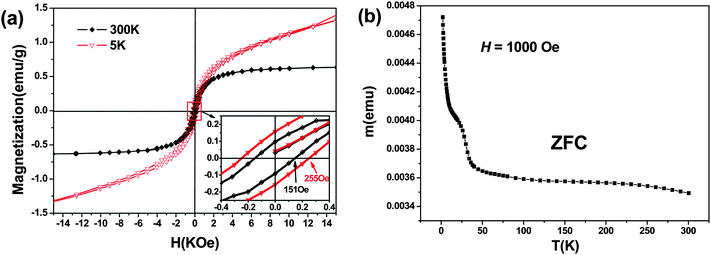 |
| Fig. 5 (a) Magnetization as a function of magnetic field for the as-grown (Mn,Co)-codoped ZnO NWs at 5 K and 300 K, respectively (the inset is the local enlarged image of magnetization as a function of magnetic field), and (b) the zero-field-cooled (ZFC) magnetization as a function of temperature (M–T) measured in a field of 1000 Oe. | |
The defects induced by Co and/or Mn doping are believed to play an important role for FM in diluted magnetic ZnO semiconductors. A general model for describing ZnO-based DMS is the strong electronic coupling between the magnetic ions and the partially delocalized charge carriers at the Fermi level, namely, the dopant-defect hybridization which is pivotal to achieving a high TCDMS.49 At equilibrium, the resonances Co2+ + edonor− ↔ Co+, and Mn2+ + hacceptor+ ↔ Mn3+ describe the dopant-donor/acceptor hybridization in n-type Co-doped and p-type Mn-doped ZnO, respectively. It is well known that the unintentionally doped ZnO is n-type with the intrinsic lattice defect Zni, which is a shallow donor, while the p-type ZnO is hardly realized via native defects Oi and VZn. The Co/Mn doping may change the perfect ZnO structure to make it possible for forming defects or holes, which could be regarded as additional charge carriers.31,50 Additionally, the metallic Co/Mn is an unlikely source of this FM because the XRD and high-resolution TEM results clearly show no metallic Co/Mn clusters in the nanowires. Hence, the origin of ferromagnetic behavior observed in Co/Mn-codoped ZnO NWs is due to the bivalent Co+, Co2+, Mn2+, Mn3+ cations doping into the ZnO NWs.
4. Conclusions
In summary, Co/Mn-codoped ZnO NWs have been synthesized by the thermal evaporation method. The structural analysis shows that the Co and Mn ions were successfully induced into the ZnO NWs with hexagonal wurtzite structure and no second phase exists. In particular, the optical, electrical and magnetic properties of the ZnO NWs were changed by Co and Mn codoping. The novel Raman peak at 519.3 cm−1 suggested that the two different kinds of cations via doping could affect the local polarizability, and the electrical measurement shows that the Co/Mn-codoping enhances the conductivity an order of magnitude compared with undoped ZnO NW due to the presence of Co and Mn cations. In addition, the magnetic measurement indicates that the Co/Mn-codoped ZnO NWs have obvious ferromagnetic characteristics at room temperature. The Co/Mn-codoped NWs show a predominant conducting and room temperature FM, which suggests a potential application in optoelectronic and spintronic devices.
Acknowledgements
This work was supported by the National Basic Research Program of China (Grant No. 2007CB936201), the Funds for International Cooperation and Exchange (Grant Nos. 50620120439, 2006DFB51000), the Science Foundation (Grant Nos. 50772011, NCET-07-0066) and the Fundamental Research Funds for the Central Universities.
References
- Z. W. Pan, Z. R. Dai and Z. L. Wang, Science, 2001, 291, 1947 CrossRef CAS.
- X. D. Wang, J. H. Song, J. Liu and Z. L. Wang, Science, 2007, 316, 102 CrossRef CAS.
- Y. W. Heo, L. C. Tien, Y. Kwon, D. P. Norton, S. J. Pearton, B. S. Kang and F. Ren, Appl. Phys. Lett., 2004, 85, 2274 CrossRef CAS.
- Y. Yang, Q. L. Liao, J. J. Qi, Y. Zhang, L. D. Tang and N. Ye, Appl. Phys. Lett., 2008, 93, 133101 CrossRef.
- B. A. Buchine, W. L. Hughes, F. L. Degertekin and Z. L. Wang, Nano Lett., 2006, 6, 1155 CrossRef CAS.
- Y. W. Heo, L. C. Tien, D. P. Norton, B. S. Kang, F. Ren, B. P. Gila and S. J. Pearton, Appl. Phys. Lett., 2004, 85, 2002 CrossRef CAS.
- S. K. Panda, A. Dev and S. Chaudhuri, J. Phys. Chem. C, 2007, 111, 5039 CrossRef CAS.
- Y. Qin, R. Yang and Z. L. Wang, J. Phys. Chem. C, 2008, 112, 18734 CAS.
- L. E. Greene, M. Law, D. H. Tan, M. Montano, J. Goldberger, G. Somorjai and P. D. Yang, Nano Lett., 2005, 5, 1231 CrossRef CAS.
- L. Li, S. S. Pan, X. C. Dou, Y. G. Zhu, X. H. Huang, Y. W. Yang, G. H. Li and L. D. Zhang, J. Phys. Chem. C, 2007, 111, 7288 CrossRef CAS.
- Q. C. Li, V. Kumar, Y. Li, H. T. Zhang, T. J. Marks and R. P. H. Chang, Chem. Mater., 2005, 17, 1001 CrossRef CAS.
- T. J. Sun, J. S. Qiu and C. H. Liang, J. Phys. Chem. C, 2008, 112, 715 CrossRef CAS.
- G. Z. Shen, Y. Bando and D. Golberg, J. Phys. Chem. C, 2007, 111, 5044 CrossRef CAS.
- H. F. Li, Y. H. Huang, Y. Zhang, J. J. Qi, X. Q. Yan, Q. Zhang and J. Wang, Cryst. Growth Des., 2009, 9, 1863 CrossRef CAS.
- L. Yan, C. K. Ong and X. S. Rao, J. Appl. Phys., 2004, 96, 508 CrossRef CAS.
- K. R. Kittilstved and D. R. Gamelin, J. Appl. Phys., 2006, 99, 08M112 CrossRef.
- P. Sharma, A. Gupta, K. V. Rao, F. J. Owens, R. Sharma, R. Ahuja, J. M. Osorio Guillen, B. Johansson and G. A. Gehring, Nat. Mater., 2003, 2, 673 CrossRef CAS.
- M. Venkatesan, C. B. Fitzgerald, J. G. Lunney and J. M. D. Coey, Phys. Rev. Lett., 2004, 93, 177206 CrossRef CAS.
- S. J. Han, T. H. Jang, Y. B. Kim, B. G. Park, J. H. Park and Y. H. Jeong, Appl. Phys. Lett., 2003, 83, 920 CrossRef CAS.
- U. Philipose, S. V. Nair, S. Trudel, C. F. de Souza, S. Aouba, R. H. Hill and H. E. Ruda, Appl. Phys. Lett., 2006, 88, 263101 CrossRef.
- J. J. Liu, M. H. Yu and W. L. Zhou, Appl. Phys. Lett., 2005, 87, 172505 CrossRef.
- X. H. Zhang, X. Q. Yan, J. Zhao, Z. Qin and Y. Zhang, Mater. Lett., 2009, 63, 444 CrossRef CAS.
- X. M. Zhang, Y. Zhang, Z. L. Wang, W. J. Mai, Y. D. Gu, W. S. Chu and Z. Y. Wu, Appl. Phys. Lett., 2008, 92, 162102 CrossRef.
- M. Bouloudenine, N. Viart, S. Colis, J. Kortus and A. Dinia, Appl. Phys. Lett., 2005, 87, 052501 CrossRef.
- I. Djerdj, Z. Jaglicic, D. Arconde and M. Niederberger, Nanoscale, 2010, 2, 1096 RSC.
- I. Djerdj, G. Garnweitner, D. Arcon, M. Pregelj, Z. Jaglicic and M. Niederberger, J. Mater. Chem., 2008, 18, 5208 RSC.
- J. M. D. Coey, M. Venkatesan and C. B. Fitzgerald, Nat. Mater., 2005, 4, 173 CrossRef CAS.
- P. V. Radovanovic and D. R. Gamelin, J. Am. Chem. Soc., 2001, 123, 12207 CrossRef CAS.
- D. A. Schwartz, N. S. Norberg, Q. P. Nguyen, J. M. Parker and D. R. Gamelin, J. Am. Chem. Soc., 2003, 125, 13205 CrossRef CAS.
- W. J. Liang, B. D. Yuhas and P. D. Yang, Nano Lett., 2009, 9, 892 CrossRef CAS.
- X. M. Zhang, W. Mai, Y. Zhang, Y. Ding and Z. L. Wang, Solid State Commun., 2009, 149, 293 CrossRef CAS.
- Z. B. Gu, C. S. Yuan, M. H. Lu, J. Wang, D. Wu, S. T. Zhang, S. N. Zhu, Y. Y. Zhu and Y. F. Chen, J. Appl. Phys., 2005, 98, 053908 CrossRef.
- Y. H. Huang, X. D. Bai and Y. Zhang, J. Phys.: Condens. Matter, 2006, 18, L179 CrossRef CAS.
- Z. H. Zhang, X. F. Wang, J. B. Xu, S. Muller, C. Ronning and Q. Li, Nat. Nanotechnol., 2009, 4, 523 CrossRef CAS.
- H. M. Cheng, H. C. Hsu, Y. K. Tseng, L. J. Lin and W. F. Hsieh, J. Phys. Chem. B, 2005, 109, 8749 CrossRef CAS.
- X. T. Zhang, Y. C. Liu, Z. Z. Zhi, J. Y. Zhang, Y. M. Lu, D. Z. Shen, W. Xu, G. Z. Zhong, X. W. Fan and X. G. Kong, J. Phys. D: Appl. Phys., 2001, 34, 3430 CrossRef CAS.
- L. L. Yang, Q. X. Zhao, M. Willander, J. H. Yang and I. Ivanov, J. Appl. Phys., 2009, 105, 053503 CrossRef.
- X. F. Wang, R. K. Zheng, Z. W. Liu, H. P. Ho, J. B. Xu and S. P Ringer, Nanotechnology, 2008, 19, 455702 CrossRef.
- X. F. Wang, J. B. Xu, X. J. Yu and K. Xue, Appl. Phys. Lett., 2007, 91, 031908 CrossRef.
- R. Cuscó, E. Alarcón-Lladó, J. Ibáñez, L. Artús, J. Jiménez, B. Wang and M. J. Callahan, Phys. Rev. B: Condens. Matter Mater. Phys., 2007, 75, 165202 CrossRef.
- J. Zhao, X. Q. Yan, Y. Yang, Y. H. Huang and Y. Zhang, Mater. Lett., 2010, 5, 569 CrossRef.
- X. Q. Yan, Y. S. Gu, X. M. Zhang, Y. H. Huang, J. J. Qi, Y. Zhang, T. Fujita and M. W. Chen, J. Phys. Chem. C, 2009, 113, 1164 CrossRef CAS.
- J. H. He, C. S. Lao, L. J. Chen, D. Davidovic and Z. L. Wang, J. Am. Chem. Soc., 2005, 127, 16376 CrossRef.
- H. Kind, H. Q. Yan, B. Messer, M. Law and P. D. Yang, Adv. Mater., 2002, 14, 158 CrossRef CAS.
- X. F. Wang, F. Q. Song, Q. Chen, T. Y. Wang, J. L. Wang, P. Liu, M. Q. Shen, J. G. Wan, G. H. Wang and J. B. Xu, J. Am. Chem. Soc., 2010, 132, 6492 CrossRef CAS.
- J. Y. Park, Y. S. Yun, Y. S. Hong, H. Oh, J. J. Kim and S. S. Kima, Appl. Phys. Lett., 2005, 87, 123108 CrossRef.
- Y. Huang, X. Duan, Y. Cui and C. M. Lieber, Nano Lett., 2002, 2, 101 CrossRef CAS.
- Z. B. Gu, C. S. Yuan, M. H. Lu, J. Wang, D. Wu, S. T. Zhang, S. N. Zhu, Y. Y. Zhu and Y. F. Chen, J. Appl. Phys., 2005, 98, 053908 CrossRef.
- K. R. Kittilstved, W. K. Liu and D. R. Gamelin, Nat. Mater., 2006, 5, 291 CrossRef CAS.
- L. B. Duan, G. H. Rao, Y. C. Wang, J. Yu and T. Wang, J. Appl. Phys., 2008, 104, 013909 CrossRef.
|
This journal is © The Royal Society of Chemistry 2011 |
Click here to see how this site uses Cookies. View our privacy policy here.