DOI:
10.1039/C0NR00610F
(Paper)
Nanoscale, 2011,
3, 1139-1148
Aqueous synthesis of ZnTe/dendrimer nanocomposites and their antimicrobial activity: implications in therapeutics†
Received
18th August 2010
, Accepted 15th October 2010
First published on 7th January 2011
Abstract
The present strategy proposes a simple and single step aqueous route for synthesizing stable, fluorescent ZnTe/dendrimer nanocomposites with varying dendrimer terminal groups. In these hybrid materials, the fluorescence of the semiconductor combines with the biomimetic properties of the dendrimer making them suitable for various biomedical applications. The ZnTe nanocomposites thus obtained demonstrate bactericidal activity against enteropathogenic bacteria without having toxic effects on the human erythrocytes. The average size of the ZnTe nanoparticles within the dendrimer matrix was in the range of 2.9–6.0 nm, and they have a good degree of crystallinity with a hexagonal crystal phase. The antibacterial activities of the ZnTe/dendrimer nanocomposites (ZnTe DNCs) as well other semiconductor nanocomposites were evaluated against enteropathogenic bacteria including multi-drug resistant Vibrio cholerae serogroup O1 and enterotoxigenic Escherichia coli (ETEC). ZnTe DNCs had significant antibacterial activity against strains of V. cholerae and ETEC with minimum inhibitory concentrations ranging from 64 to 512 μg ml−1 and minimum bactericidal concentrations ranging from 128 to 1000 μg ml−1. Thus, the observed results suggest that these water-soluble active nanocomposites have potential for the treatment of enteric diseases like diarrhoea and cholera.
1. Introduction
Hybrid dendrimer nanocomposites (DNCs) are an emerging class of new materials that hold significant promise in diverse fields, such as bio-imaging, non-linear optics, sensors, catalysis and cancer treatment, and can serve as building blocks for highly ordered nanostructures including self-assembled ultrathin multilayers and smart nano-devices.1–6Dendrimer-mediated synthesis exhibits a greater degree of control with respect to their composition, size, shape and surface functionalities, which in turn, imparts stability, biocompatibility and water-solubility to the nanocomposites. So, these novel hybrid materials are ideally suited for various biomedical applications.7–12
There have been several reports on the synthesis of metal sulfides, like CdS and ZnS nanocrystals (quantum dots), in a dendrimer matrix.10–16 We earlier observed that sulfide based semiconductor nanocrystals synthesized in a dendrimer matrix showed relatively low luminescence quantum efficiency compared to telluride based ones. However, literature on the synthesis of metal telluride nanocrystals in a dendrimer matrix is limited.17 In the present study, we have endeavoured to synthesize water soluble, biologically suitable ZnTe/dendrimer nanocomposites because of the exciting optical properties of ZnTe nanocrystals (direct transition band gap of 2.26 eV at room temperature).18 Various synthetic methodologies have been tried for the production of colloidal ZnTe nanocrystals.19 Among these, the most successful approach for obtaining good quality particles was the pyrolysis of organometallic precursors at high temperature.20 However, nanoparticles obtained via organometallic routes are not fully compliant, or rather fail the criteria for biomedical applications.21–25 Hence, aqueous synthesis is an alternative and important strategy to directly prepare water-dispersed quantum dots (QDs) from the point of view of biological applications. However, ZnTe nanocrystals synthesized earlier in aqueous medium were quite large and also of poor luminescent qualities.26 Here, we have demonstrated that ZnTe/dendrimer nanocomposites with a narrow size distribution and reasonably good quantum yield can be synthesized through an aqueous route and are useful for biological applications. The dendrimer prevents agglomeration and stabilizes the nanoparticles, making it possible to tune solubility. Furthermore, dendrimer provides a means of immobilization of the nanoparticles on a solid support and to afford controllable self-assembly of entrained nanoclusters on a variety of surfaces through chemi-sorption.13,16 Additionally, the surface group of the dendrimer remains free and can be utilized for conjugation with other biomolecules for biosensors and biolabelling experiments.11,13–16 Formation of nanoparticles in a dendrimer matrix opens up the possibility of fabricating new materials and devices with novel or enhanced physical and chemical properties as interactions between proximal nanocrystals give rise to new collective phenomena.
In recent times, inorganic nanoparticles with antimicrobial activity have been emerging as a new class of biomedical materials to fulfill the increasing general demands for hygiene in daily life due to their large specific surface area and high bioactivity.27 A number of nanoparticles with antimicrobial activities have been reported recently, particularly, silver or gold nanoparticles have been used extensively in many bactericidal fields.28–30 Their predominant antimicrobial activity can be attributed to the strong cytotoxicity to various bacterial cells, i.e., they can interact with the functional groups on the bacterial cell surface and inactivate bacteria.31–34 QDs have superior size-dependent optical properties and play an important role in the studies of complex microbial populations and the identification of bacteria, through the construction of probe-conjugated QDs for single bacterium imaging.35,36 In contrast, little attention has been paid to exploring the potential of antimicrobial activities of QDs.37–39
The incidence of diarrhoeal disease varies largely according to geography, with an estimated incidence between 1.3 and 2.3 episodes of diarrhoea per child per year in developed countries compared with 3–9 episodes per child per year in the developing world. Diarrhoea still accounts for 1.6–2.5 million deaths annually in children below 5 years of age.40 Among the diarrhoeal diseases, cholera is a serious epidemic disease caused by the Gram-negative bacterium Vibrio cholerae.41 Treatment with oral rehydration solution (ORS) has reduced the levels of mortality in children and adults by dehydration, but not morbidity for diarrhoea.42 Although the drugs such as racecadotril and loperamide are used to treat secretory diarrhoea, these drugs have side effects such as bronchospasm, vomiting and fever.43Antibiotics are used to treat diarrhoeal patients. However, antibiotic resistance has been of great concern due to the extensive use of classical antibiotics.44,45Antibiotic resistance among the pathogens is a serious clinical problem in the treatment and containment of the disease. Different inorganic nanoparticles have elicited much interest due to their potential for achieving a specific process and selectivity in biological and pharmaceutical applications.32–34,46 However, all studies are focused on the antimicrobial effects of cadmium based particles such as CdSe and CdTe-core QDs. Because their chemical and physical properties play vital roles in their bioactivities, QDs in a polymer matrix may have a distinct interaction with microbes. The antibacterial properties of semiconductor/nanocomposites have not been studied yet. Thus, it is of great importance to investigate the antimicrobial activities of semiconductor dendrimer nanocomposites that are composed of other zinc based core materials. The present investigation proposes an aqueous synthesis of ZnTe/dendrimer nanocomposites, which show significant antimicrobial activity towards enteropathogens without having toxicity on human erythrocytes.
2. Experimental
2.1 Reagents
Starburst PAMAM dendrimers of generation having surface amino group, half generation 3.5 with carboxyl end groups, hydroxyl end groups (G4) and with succinamic acid end groups were purchased from Sigma Aldrich. ZnSO4·7H2O, CdCl2·4H2O, sodium thiosulfate (Na2S2O3·5H2O), were obtained from Merck, India, and Telluric acid (H2TeO4·2H2O) and Sodium borohydride (NaBH4) (from BDH, India). All chemicals used were of analytical reagent grade. Milli-Q water (Millipore) and methanol (HPLC grade) were used as solvents. The dendrimer solution (1.73 × 10−4 M) in water under N2 atmosphere at 10 °C was prepared freshly before the synthesis taking into account the manufacturer's value of the dendrimer weight fractions in methanol and the known dendrimer densities.
2.2 Preparation of NaHTe solution
In the preparation of NaHTe solution, 4.5 mg of telluric acid was dissolved in minimum amount of water (∼0.3 ml) and the solution was heated with borohydride under a continuous flow of nitrogen gas until the colour changed from black to colourless. Then the colourless solution was kept in an ice bath for 2–3 h after which a white precipitate of sodium tetraborate came out. The clear supernatant containing NaHTe free from contamination of borates was used for the synthesis.
2.3 Preparation of ZnTe/dendrimer nanocomposites
A typical preparation of ZnTe/dendrimer nanocomposites with an initial Zn2+/Te2− molar ratio of 1
:
1 was as follows: 5 ml aliquot of Zn2+ stock solution (2.0 mM) in water was added to 10 ml of dendrimer solution at 10 °C and vigorously stirred for 2 min to make the final concentration of Zn2+ and dendrimer as 2 × 10−3 M and 1 × 10−4 M, respectively. Then, NaHTe solution (as prepared above) was taken in a syringe and injected to the argon purged solution mixture of Zn2+ and dendrimer. The resulting solution was pale yellow and did not show any evidence of precipitates. In a similar manner, CdTe/dendrimer nanocomposites has been prepared using Cd2+ stock solution in place of Zn2+ stock solution at the same concentration.17 The ZnS/dendrimer and CdS/dendrimer nanocomposites used in the microbial study were prepared following our methods reported earlier.15,47 It was observed that there was no significant change in absorption spectral profile of the semiconductor nanoparticles in dendrimer matrix for a period of one month. Unaltered absorption onset suggests that the particle size has not changed. The as-prepared nanocomposite solutions were concentrated 4–5 times by freeze-drying, in which a pressure of 10 mTorr and temperature of −85 °C were maintained. Further, on gradual addition of a ‘non-solvent’ (isopropanol) to the colloidal solution of nanocomposites, different fractions precipitated. The precipitates thus obtained were washed with acetone followed by diethyl ether to remove residual chemicals. The nanocomposites were then dried and stored in vacuum desiccators.
2.4 Characterization and spectral analyses
Transmission electron microscopy (TEM) was carried out on JEOL-2010 with acceleration voltage of 200 kV. A drop of as-prepared solution of ZnTe/dendrimer nanocomposite was placed on a carbon-coated copper grid of 300 mesh and dried before putting it on to the TEM sample chamber. About eight images are taken for each sample. The crystal phases of the nanocrystals were characterized by X-ray powder diffraction (XRD) measurements using a Philips Analytical X-Ray B.V. diffractometer type PW 1710 equipped with graphite mono chromatized Cu-Kα radiation (λ = 1.54056 Å). A scanning rate of 0.02° per 2 s in 2θ range from 10°–80° was employed.
UV–Vis absorption spectra were recorded with a Shimadzu UV-1601PC spectrophotometer. Photoluminescence measurements of ZnTe NPs were monitored by Perkin Elmer LS-55 luminescence spectrometer. pH measurement was performed on a Jenways ion-meter. Size distribution and zeta potential of ZnTe/dendrimer nanocomposites were determined by dynamic light scattering spectrophotometer (Model DLS - nanoZS, Zetasizer, Nanoseries, Malvern Instruments). Samples were filtered several times through a 0.22 μm Millipore membrane filter prior to recording measurements. The zeta potential was calculated from the electrophoretic mobility using the Smoluchowski equation with the help of commercial software. The results are expressed as mean values of three samples. Zeta potential measurements were performed at 25 °C. The FTIR spectra were recorded with Perkin Elmer, Spectrum GX equipment with a resolution of 2 cm−1 and scan range of 1000–4000 cm−1. Fluorescence images were recorded using fluorescence and phase-contrast microscope (Model BX51/B52; Olympus, Japan).
2.5 Screening for antimicrobial activity
The antibacterial activity of the nanocomposite against some of the pathogenic Gram-positive and Gram-negative bacteria was determined by agar-diffusion assay as described previously48 and following the method of Reeves et al.49 Among the Gram-negative bacteria, clinical isolates of Vibrio cholerae strains NB2 (O1 serotype); and Escherichia coli strain PC80 (Enterotoxigenic Escherichia coli, ETEC) were used in this study. The other strains used in this study were Gram-positive bacteria including Staphylococcus aureus ATCC 25923, and Bacillus subtilis ATCC 6623. Bacterial strains were first grown in Mueller-Hinton broth (MHB) (HiMedia, Mumbai, India) under shaking condition for 4 h at 37 °C, and after the incubation, 1 ml of culture was spread on Mueller-Hinton agar (MHA) (HiMedia). The wells were made using a sterile 6 mm cork borer in the inoculated MHA plate. The wells were filled with 50 μl (2 mg ml−1) of the samples of nanocomposites (re-suspended in water) and blanks (water). Tetracycline (10 μg/50 μl) was used as antibacterial positive control and E. coli ATCC 25922 was included for quality assurance. Zone diameter was measured after 24 h incubation at 37 °C. The photograph was taken in Gel documentation system (Vilber Lourmat, France).
2.6 Determination of minimum inhibitory concentration (MIC) and minimum bactericidal concentration (MBC)
MIC and MBC of the dendrimer nanocomposites were determined using broth micro dilution as described previously48 following the method recommended by the National Committee for Clinical Laboratory Standards.50,51 An inoculum of the microorganism was prepared from 24 h MHB cultures and suspensions were adjusted with turbidity equivalent to that of a 0.5 McFarland standard. Bacterial suspensions were further diluted 1
:
10 in sterile MHB to obtain a final inoculum of 5 × 105CFU ml−1 (colony forming units). The 96-well round bottom sterile plates were prepared by dispensing 180 μl of the inoculated broth into each well. A 20 μl aliquot of the sample was added. The concentrations of samples tested were 1, 2, 4, 8, 16, 32, 64, 128, 500, and 1000 μg ml−1. Dilutions of tetracycline served as positive control, while broth with 20 μl of water was used as negative control. E. coli ATCC 25922 was included for quality assurance purposes. Plates were covered and incubated for 24 h in ambient air at 37 °C. After incubation, minimum inhibitory concentrations (MIC) were read visually; all wells were plated to nutrient agar (Hi-Media) and incubated. The minimal bactericidal concentration (MBC) was defined as a 99.9% reduction in CFU from the starting inoculum after 24 h incubation interval.
2.7 The time-kill kinetic study
Cultures of V. choleraeNB2 in MHB (around 1 × 107CFU ml−1) were incubated separately in the absence (control) and in the presence of ZnTe_G4.NH2 at a concentration of 128 μg ml−1 (MBC) for a period of 12 h at 37 °C. Samples of the bacterial cultures were removed at 2 h intervals to record survival counts, expressed as CFU ml−1. The surviving log10CFU ml−1 was plotted against time.
2.8 Haemolytic activity
A haemolysis test was employed to determine cellular toxicity of the nanocomposites as previously described.52 The nanocomposites at concentrations ranging from 1× to 8× MBC, were incubated with an equal volume of 1% human red blood cells in phosphate buffered saline (10 mM PBS, pH 7.4) at 37 °C for 1 h. Non-haemolytic and 100% haemolytic controls were the buffer alone and the buffer containing 1% Triton X-100, respectively. Cell lysis was monitored by measuring the release of haemoglobin at 540 nm.
2.9 Statistical analysis
Values are expressed, as mean ± S.D. Statistical significance was determined using Student's t-test. Values with p < 0.05 were considered significant.
3. Results and discussion
3.1 Characterization of particle size by TEM and XRD
A typical transmission electron microscopic image for ZnTe/dendrimer nanocomposites is shown in Fig. 1a, which yields an average particle size of about 3.0 nm for the synthesis in water at 10 °C using NH2 terminated dendrimer. The shape of the ZnTe nanocrystals are not spherical but slightly oblate as evident from the TEM image (Fig. 1a). The corresponding particle size of ZnTe NPs as determined from the respective absorption onset is 2.9 nm. This is in good agreement with the size calculated from TEM data. Lattice fringes can be discerned in the high resolution TEM image, which suggest good crystallinity of ZnTe NPs. The inter-planar distance was found to be 3.9 Å. The SAED pattern displays bright rings at a distance of 3.11, 2.24, 1.93, 1.33 and 1.167 Å corresponding to 003, 103, 111, 210, and 300 lattice planes of the hexagonal crystal phase of ZnTe (JCPDS no. 83–0967). It appears that the size of the ZnTe nanocrystal in the NH2 terminated dendrimer is smaller and more monodisperse than that of ZnTe nanocystal (3.3 nm and 6.0 nm) using COOH and OH terminated dendrimer respectively (as shown in Fig. 1b, c). ZnTe nanocrystals in the NH2 terminated dendrimer matrix are less aggregated compared to the COOH and OH terminated dendrimer (ESI†), presumably due to the existence of the primary as well as internal secondary amine groups of dendrimers that more effectively prevent the aggregation of ZnTe NPs during their formation process. It may be noted that in our earlier study, the size of the particles of ZnS in the dendrimer matrix could be tuned in the range of 2.2–3.1 nm whereas, in case of CdS, it was within 2.8–3.9 nm. However, the observed size tunability in the presently synthesized ZnTe nanocrystals is in the range of 2.9–6.0 nm.
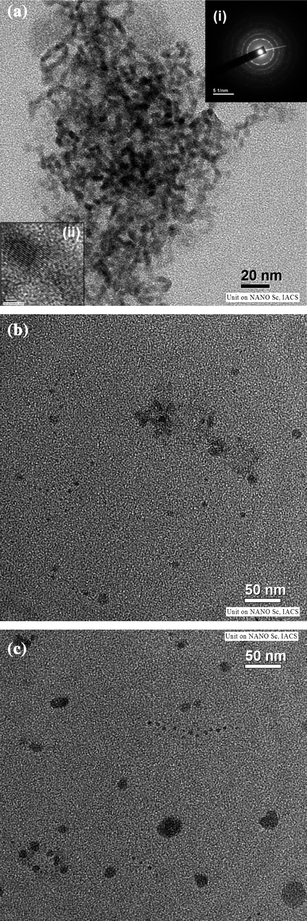 |
| Fig. 1
(a)
TEM image of ZnTe/dendrimer nanocomposites using NH2 terminated dendrimer. (b)TEM image of ZnTe/dendrimer nanocomposites using COOH terminated dendrimer. (c)TEM image of ZnTe/dendrimer nanocomposites using OH terminated dendrimer. | |
Typical X-ray powder diffraction (XRD) patterns of the as-prepared ZnTe nanocrystals are shown in Fig. 2. The three distinct diffraction peaks were observed at 2θ values of 27.6°, 38.4° and 48.4°, respectively, corresponding to the (101), (103) and (112) crystalline planes of hexagonal ZnTe (JCPDS No. 830–967). The broad nature of the XRD peaks could be attributed to the nano-crystalline nature of ZnTe particles. No other characteristic peaks of impurities were observed.
3.2
Nanoparticles/PAMAM dendrimer binding
It was found that a strong coordinating interaction did exist between the nanoparticles and the amide moieties—tertiary amine groups and surface end groups such as amino, carboxyl, hydroxyl, and sucinamic acid groups of PAMAM-dendrimer. Typical FTIR spectra of dendrimer and ZnTe/dendrimer nanocomposites with terminal COOH are depicted in Fig. 3. It appears that the bands at 1562 and 1641 cm−1 assigned for symmetric stretching mode of C–O or bending mode of O–H are shifted to 1567 and 1649 cm−1, respectively. Again, the band at 3286 cm−1 corresponding to stretching mode of hydroxyl group (OH) is shifted to 3357 cm−1 suggesting that NPs are attached to the dendrimer through the surface carboxyl (COOH) group.
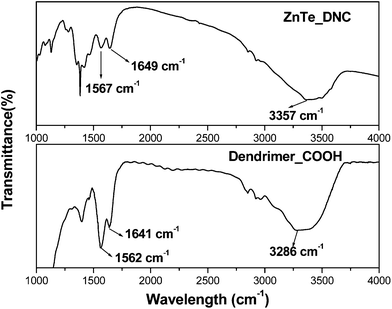 |
| Fig. 3
FTIR spectra of COOH terminated dendrimer and ZnTe/dendrimer nanocomposites. | |
3.3 Functionalization of ZnTe/dendrimer nanocomposites
We have previously reported that subtle variation of the terminal group of poly(amidoamine) (PAMAM) dendrimers can effectively control the quality of nanoparticles formed.15,47 In this study, we describe the templated synthesis and characterization of ZnTe/dendrimer nanocomposite using PAMAM dendrimer with different terminal groups such as amino (NH2), carboxyl (COOH), hydroxyl (OH) and succinamic acid (SAH). These nanocomposites were prepared under identical conditions (at 10 °C, fixed molar ratio) following the method described earlier. Fig. 4 displays the optical spectra of ZnTe/dendrimer nanocomposites. It was observed that a sharp excitonic peak was obtained in the case of ZnTe/dendrimer nanocomposites with terminal NH2 (ZnTe_G4.NH2) and COOH (ZnTe_G3.5COOH), whereas a relatively broad spectrum and a broad shoulder were observed with terminal OH (ZnTe_G4.OH) and SAH (ZnTe_G3.5SAH) respectively. ZnTe nanocrystals in the dendrimer matrix (in the case of amine and carboxyl end groups of dendrimer) show well-defined 1s–1s electronic transitions in the absorption spectra. The absorbance edge of the nanocrystals was 329 and 338 nm respectively and the band gap was calculated to be 3.7 and 3.6 eV. The absorbance edge blue-shifts 219 nm compared with bulk ZnTe (the band gap is 2.26 eV, and the absorbance edge is 548 nm). In fact, the value of the energy gap, estimated from Fig. 4, reflects a considerable blue shift relative to the absorption band edge of bulk ZnTe. Generally, the wavelength of the exciton absorption band decreases with decreasing particle size as a result of quantum confinement of the photo-generated electron hole pairs.53
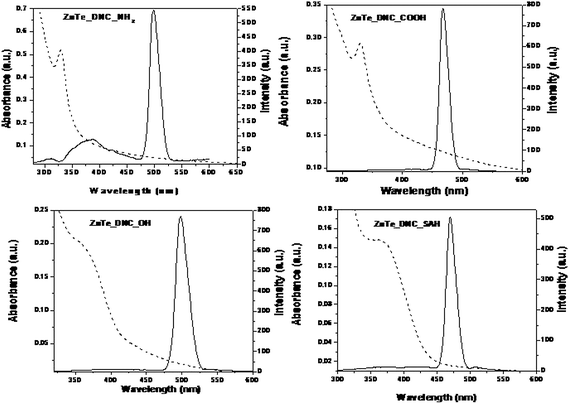 |
| Fig. 4 Functionalization of ZnTe/dendrimer nanocomposites in the presence of amino (NH2), carboxyl (COOH), hydroxyl (OH), succinamic acid (SAH) terminated dendrimers. | |
On the other hand, NH2- and COOH-terminated dendrimers produced smaller ZnTe NPs (2.9 and 3.5 nm, respectively) as compared to SAH-terminated dendrimer (5.0 nm). This is probably due to the differential interactions of ZnTe NPs with the surface groups of the dendrimers. Strong electrostatic interaction between Zn2+ and NH2 or COOH groups controls the growth and stabilization of the ZnTe NPs within the dendrimer matrix. Similar terminal group dependence was observed in ZnS/dendrimer nanocomposites and CdS/dendrimer nanocomposites synthesized via chemical or radiolytic routes.15,47 The PL spectrum consists of a peak around 310 nm originating from electron-hole recombination (band edge emission) and a strong peak around 497 nm due to recombination via surface localized state (trap state emission). Band edge emission strongly depends on particle size within the quantum confinement regime. Here, in the present study, we have not observed any significant peak shift in PL emission at 497 nm, when particle size was varied. Moreover, Stokes' shift is usually found to be small in case of band edge emission.53–55 The absorption and emission spectra of the ZnTe/dendrimer nanocomposites suggest that there is a substantial Stokes' shift from 329 to 497 nm. Thus, a size invariant PL maxima and a large Stokes' shift suggest that photoluminescence originates from defect-states. However, the predominance of trap emission suggests that the surface defects are not adequately passivated. It is also possible that the majority of defects originate from the core atoms of the nanocrystals, which remain inaccessible to the ligand-moieties of the dendrimer. The maximum PLQE of 15% was obtained with NH2-terminated dendrimer for the particle size of 2.5 nm, while the lowest PLQE (9%) was obtained for OH-terminated dendrimer (Table 1). It can be concluded that NH2-, COOH- and SAH-terminated dendrimers provide better protection than OH-terminated dendrimer and yield higher luminescence quantum efficiencies. Furthermore, the fluorescence image shows that, in contrast to OH-terminated dendrimer, NH2-terminated dendrimer provides better protection and produces highly luminescent ZnTe nanoparticles (Fig. 5a, b). Since dendrimers containing an almost unlimited range of cores and peripheral groups may be synthesized, it is now possible to easily control nanocluster properties such as composition, size, morphology, solubility and degree of encapsulation. One application of this latter property is to control the release rate of entrained medicinal agents/sensors based on structural or environmental changes, for targeted drug delivery or in situ monitoring.
Table 1 Average zeta potential and photoluminescence efficiency data of ZnTe/dendrimer nanocomposites
Materials |
Zeta potential (mV) |
Photoluminescence efficiency (%) |
ZnTe_G4.NH2 |
+8.1 ± 0.3 |
15.0 |
ZnTe_G3.5COOH
|
− 49.5 ± 0.2 |
12.0 |
ZnTe_G4.OH
|
−35 ± 0.4 |
9.0 |
ZnTe_G4.SAH
|
−35.6 ± 0.5 |
11.0 |
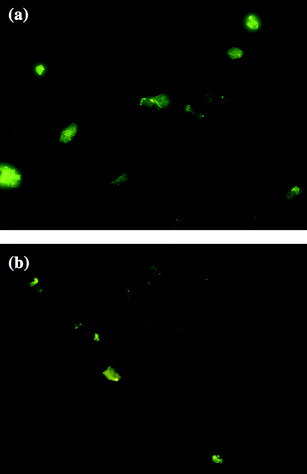 |
| Fig. 5
(a)
Fluorescence image of ZnTe/dendrimer nanocomposites using NH2-terminated dendrimer (at 100× magnification). (b)Fluorescence image of ZnTe/dendrimer nanocomposites using OH-terminated dendrimer (at 100× magnification). | |
3.4 Zeta potential
Zeta potential plays a crucial role, as the surface charge property of a material influences the biological performance under physiological conditions. By varying the surface charges one can vary the electrostatic interaction, hydrophobic interaction and specific chemical interaction between the NPs and various biological entities for specific applications such as drug delivery, intracellular targeting, cellular uptake, etc.15,56
The surface charge of the ZnTe/dendrimer nanocomposites was measured by zeta potential, which depends on the terminal groups of the dendrimer as shown in Table 1. Amine terminated ZnTe/dendrimer nanocomposites were positively charged, whereas carboxyl and succinamic acid terminated dendrimer nanocomposites were negatively charged indicating that functional groups of the dendrimer molecule is not significantly influenced after the formation of the hybrid nanostructures. This further indicates that after the formation of the hybrid nanostructures the terminal amines of the dendrimers, which can be used to link biological ligands, are still available. Importantly, recent advances in using functionalized dendrimers for targeting, imaging and drug molecules9,57 suggest that nanoparticles within a dendrimer matrix are ideal nanodevices for a range of biomedical applications.58,59 In addition, the surface charge polarity of ZnTe/dendrimer nanocomposites is similar to the corresponding dendrimer molecule. It can be concluded that the dendrimer molecule imparts the surface charge tunability to the ZnTe/dendrimer nanocomposites. It is expected that the higher surface charge of the particles would help nanoparticles not to aggregate and retain good colloidal stability through electrostatic repelling forces. However, nanocomposites using amine terminated dendrimer possessing small positive charge are found to be highly stable. However, it is difficult to make a comparison between dendrimer terminal groups and the higher surface potential of the particles in terms of the stabilization of the ZnTe nanocrystal. For the matter of surface potential effect, earlier work shows that fully acetylated Au nanoparticles in a dendrimer matrix with a close to neutral surface potential still retain good colloidal stability.6 Importantly, however, irrespective of the zeta potential, the NPs remained stable in aqueous solutions, indicating the strong colloidal stabilising effect of the dendrimer with a large number of surface end groups.
3.5 Antimicrobial activity of semiconductor/dendrimer nanocomposites
We tested the strains of V. cholerae, E. coli, S. aureus and B. subtilis to evaluate the antibacterial activity of the ZnTe/dendrimer nanocomposites and other II–VI semiconductor/dendrimer nanocomposites. The strains of V. cholerae and E. coli included in this study are multi-drug resistant. However, some of the dendrimer nanocomposites tested here showed inhibitory activity against V. cholerae O1 and ETEC by agar-diffusion assay with significance (p < 0.05) (Table 2 and Fig. 6). However, except one (CdTe_G4.NH2), none of the dendrimer nanocomposites was active against the Gram-positive bacteria used in this study. CdTe_G4.NH2 was active against B. Subtilis but exhibited bactericidal activity at very high concentrations of more than 1000 μg ml−1. In contrast to semiconductor/dendrimer nanocomposites, PAMAM dendrimer encapsulated silver nanoparticles have good antimicrobial activity against Gram-positive bacteria.60CdS_G4.NH2 and ZnS_G4.NH2 nanocomposites were not active against any of the strains used in this study.
Table 2 Antibacterial activity of nanocomposites determined by agar-diffusion method
Bacterial strains |
Zone of inhibition diameter (mm) |
Nanocomposites [50 μl (2 mg ml−1)/well] |
Antibiotic
|
Control |
ZnTe_G4.NH2 |
ZnTe_G3.5COOH
|
ZnTe_ G3.5SAH |
Bulk ZnTe |
CdTe_
G4.NH2 |
Tetracycline [50 μl (0.2mg ml−1)/well] |
Water
|
V. cholerae
NB2
|
14.6 ± 0.3 |
11.3 ± 0.4 |
11.8 ± 0.4 |
19.3 ± 0.3 |
13.3 ± 0.4 |
20.0 ± 0.0 |
0.0 |
E. coli
PC80
|
28.6 ± 0.7 |
20.6 ± 0.7 |
22.6 ± 0.7 |
29.8 ± 0.2 |
14.9 ± 0.0 |
0.0 |
0.0 |
B. subtillis ATCC 6623 |
0.0 |
0.0 |
0.0 |
0.0 |
12.1 ± 0.0 |
21.0 ± 0.2 |
0.0 |
S. aureus ATCC 25923 |
0.0 |
0.0 |
0.0 |
0.0 |
0.0 |
ND |
0.0 |
![Determination of the effect of nanocomposites on V. cholerae and E. coli by agar-diffusion assay method. E. coli strain PC80 (A) and V. cholerae strain NB2 (A) were spread on MHA. In each case, 50 μl of 2 mg ml−1 of nanocomposites (in water) [wells 2, ZnTe_G4.NH2; 3, ZnTe_G3.5COOH; 4, ZnTe_G3.5SAH; 6, Bulk ZnTe; 7, CdTe_G4.NH2; and 8, Zn-Dendrimer complex], 50 μl of 0.2 mg ml−1 of tetracycline (wells 1, and 9), and 50 μl of water (wells 5 and 10) were added to the wells.](/image/article/2011/NR/c0nr00610f/c0nr00610f-f6.gif) |
| Fig. 6 Determination of the effect of nanocomposites on V. cholerae and E. coli by agar-diffusion assay method. E. coli strain PC80 (A) and V. cholerae strain NB2 (A) were spread on MHA. In each case, 50 μl of 2 mg ml−1 of nanocomposites (in water) [wells 2, ZnTe_G4.NH2; 3, ZnTe_G3.5COOH; 4, ZnTe_G3.5SAH; 6, Bulk ZnTe; 7, CdTe_G4.NH2; and 8, Zn-Dendrimer complex], 50 μl of 0.2 mg ml−1 of tetracycline (wells 1, and 9), and 50 μl of water (wells 5 and 10) were added to the wells. | |
The observed differences in antibacterial activity might be attributed to the small particle size of ZnTe nanoparticles in the dendrimer matrix. Large surface area and high penetrating power make these particles effective to bind to the substrates on the outer membrane and cell membranes of organisms. Moreover, ZnTe nanocrystals possess well-developed surface chemistry, chemical stability and appropriate smaller size (3–6 nm in diameter, much smaller than a bacterium), which makes it easier for them to penetrate the microorganism cell walls. Nanocrystals are also able to maintain a constant shape and size in solution. Despite the fact that the mechanism of the interaction between nanoparticles and the constituents of the outer membrane/cell wall of micro organisms are still unanswered, it might be that the particles interact with the building elements of the outer membrane/cell wall causing structural changes, degradation and finally cell death. The semiconductor nanocomposites were most effective against Gram-negative organisms, which may be explained by the differences in the chemical nature of the material present in outer membrane and cell wall.
For the determination of MIC and MBC for the dendrimer nanocomposites against V. cholerae, and E. coli, the concentration ranges examined were from 2 to 1000 μg ml−1. ZnTe_G4.NH2 had strong bactericidal activity with MIC ranging from 64 to 128 μg ml−1 and MBC ranging from 128 to 256 μg ml−1 against V. cholerae and ETEC (Table 3). The nanocomposites CdTe_G4.NH2, bulk ZnTe and ZnTe_G3.5COOH showed activity with MIC ranging from 256 to 512 μg ml−1 and MBC 512 μg ml−1 against V. cholerae. CdTe_G4.NH2 also exhibited strong activity against ETEC. The results of the time-kill studies are shown in Fig. 7. The MBC of ZnTe_G4.NH2 (128 μg ml−1) showed a 2.5-log reduction in growth of V. cholerae in 8 h, compared to the untreated control. The time-kill kinetic study demonstrated the time-dependent bactericidal activity of ZnTe_G4.NH2. None of the active nanocomposites employed in the present study released haemoglobin and hence, these were not cytotoxic to human erythrocytes at concentrations of up to 8× MBC (see supplementary material). It was shown earlier that cytotoxicity due to semiconductor quantum dots could be correlated with surface oxidation probability and release of heavy metals. In this context, it was also envisaged that appropriate coating could render quantum dot systems non-toxic.39,61,62 It may reasonably be assumed from the observed results that dendrimers offer better surface capping leading to the non-cytotoxic nature of semiconductor/dendrimer nanocomposites.61,63 It appears from Table 2 that bulk ZnTe shows a higher zone of inhibition as compared to nanocomposites. However, if we consider the actual concentrations of ZnTe units in nanocomposites in a given sample, it appears that this semiconductor unit contributes only 24% of the weight of the sample, taking into account of large dendrimer molecule with molecular weight of 14
200 (approx.). So, the comparison of effectiveness of nanocomposites with bulk from Table 2 may not be appropriate. This is evident from the higher MIC and MBC values of bulk ZnTe in comparison with ZnTe/dendrimer nanocomposites. Furthermore, the agar diffusion assay is one method for determining the antimicrobial susceptibility and interpretation of results from this assay are analyzed based on the assumption that antibiotics diffuse freely in the solid nutrient medium. However, in many cases, this assumption may be incorrect, which leads to significant deviations of the predicted behavior from the experiment and to erroneous assessment of bacterial susceptibility to antibiotics.64 Such significant deviation is observed during the analysis of diffusion in solid agar of a number of antibiotics, especially those of more hydrophobic or amphipathic nature.64 However, the broth micro dilution method is recognized as an easy and reliable method for the determination of the MICs of antibiotics and this assay has been suggested as a reference method.65 In addition, synthesized semiconductor nanocomposites are highly water soluble and stable, whereas ZnTe is poorly soluble in water. This limits the use of bulk ZnTe in any biological system. Further, our studies also demonstrate that bulk ZnTe is toxic to human erythrocytes whereas the synthesized nanocomposites are non-toxic even at 8 times the minimum bactericidal concentrations (MBCs). The rigid structure of the dendrimer controls shape, size and stability of the nanocrystals during interaction with the bacterial surface in solution phase. During the present investigation, we observed that cysteine-capped semiconductor nanocrystals such CdTe, CdS were not effective in antimicrobial activity with regard to the strains in question. This highlights the usefulness of semiconductor/dendrimer nanocomposites for antimicrobial applications.
Table 3 Minimum inhibitory concentration (MIC) and minimum bactericidal concentration (MBC) of nanocomposites against multi-drug-resistant strains of V. cholerae and E. coli
Nanocomposites |
Antibacterial activity (μg ml−1) |
V. cholerae
NB2
|
E. coli
PC80
|
MIC |
MBC |
MIC |
MBC |
ZnTe_G4.NH2 |
64 |
128 |
128 |
256 |
ZnTe_ G3.5COOH
|
256 |
512 |
512 |
1000 |
Bulk ZnTe |
512 |
512 |
ND |
ND |
CdTe_
G4.NH2 |
512 |
512 |
128 |
256 |
Tetracycline
|
64 |
128 |
— |
— |
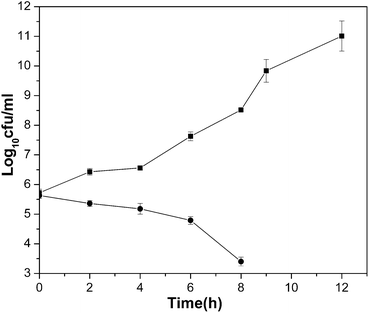 |
| Fig. 7
Cell growth of V. choleraeNB2 in the presence of ZnTe_G4.NH2 at MBC. –■–, and –●–, growth in the absence (control) and presence of the nanoparticle (128 μg ml−1), respectively. | |
The ZnTe/dendrimer nanocomposite with amine terminal group, ZnTe_G4.NH2 showed stronger antimicrobial activity than the carboxyl terminated ZnTe/dendrimer nanocomposite, ZnTe_G3.5COOH. The bacterial membranes contain mainly anionic phospholipids and no cholesterol,66 while mammalian cell membrane is mostly composed of the zwitterionic phospholipids phosphatidylcholine and sphingomyelin, along with cholesterol.67 Additionally, the outer membranes of Gram-negative bacteria and cell wall of Gram-positive bacteria contain lipopolysaccharides and teichoic acid, respectively, which adds to the negative charge of the bacterial surface.45,66Amine terminated ZnTe/dendrimer nanocomposites were positively charged; whereas carboxyl terminated dendrimer nanocomposites were negatively charged in the present study. It has been previously demonstrated that the cationic nature of native antimicrobial peptides clearly contributes to their preferential recognition by the negatively charged outer surfaces of bacterial membranes.45,68 This type of electrostatic interaction between bacterial surfaces and semiconductor nanoparticles may contribute to the better effectiveness of ZnTe_G4.NH2 than ZnTe_G3.5COOH as both have the identical dendrimer composites except their terminal groups. In addition, increased antimicrobial activity of the nanocomposites in the presence of amino terminated dendrimer may be attributed to very high local concentration (128 amino groups around a 46 Å diameter sphere) of nanoscopic size ZnTe nanocomposite particles that are accessible for micro organisms. However, interaction between the negatively charged dendrimer nanocomposites and bacterial cell membrane remains a question for further investigation.
4. Conclusion
In summary, we present here a simple approach for the synthesis of monodisperse, water-soluble, multifunctional ZnTe/dendrimer nanocomposites by a soft solution approach at low temperature. The approach of using a multifunctional dendrimer as a template to synthesize semiconductor nanoparticles could be a useful general strategy for creating multifunctional materials for a range of biomedical applications. The emergence of multiple drug resistant pathogens is a serious clinical problem in the treatment and containment of disease. However, this study showed that the semiconductor nanoparticles had significant bactericidal activity against multi-drug resistant enteropathogens including V. cholerae O1, the causative agent of the dreadful disease cholera. The MBC of ZnTe_G4.NH2 (128 μg ml−1) showed a 2.5-log reduction in growth of V. cholerae in 8 h, compared to the untreated control. Further, it may be mentioned that the present study shows that sulfide based nanocomposites are not effective as antimicrobial agents with regard to both Gram-negative and Gram-positive bacteria studied. Thus, the present investigation opens up the possibility of exploring suitable semiconductor/dendrimer nanocomposite for the development of new class of antibacterial agents. There are some questions that need to be addressed, such as, the exact mechanism of interaction of nanocomposites with the bacterial cells and the influence of surface area of nanoparticles in killing activity.
Acknowledgements
One of the authors (S.G.) is thankful to the Council of Scientific and Industrial Research, Govt. of India, for the award of Senior Research Fellowship. The authors are also thankful to the Saha Institute of Nuclear Physics, Kolkata for providing the electron microscopy facility.
References
- R. M. Crooks, B. I. Lemon, L. Sun, L. K. Yeung and M. Zhao, Top. Curr. Chem., 2000, 212, 81–135.
- K. J. Landmark, S. Dimaggio, J. Ward, C. Kelly, S. Vogt, S. Hong, A. Kotlyar, A. Myc, T. P. Thomas, J. E. P. Hahn, J. R. Baker, M. M. Holl and B. G. Orr, ACS Nano, 2008, 4, 773–783 CrossRef.
- S. Ghosh, S. C. Bhattacharya and A. Saha, Anal. Bioanal. Chem., 2010, 397, 1573–1582 CrossRef CAS.
- W. J. Scoot, O. M. Willson and R. M. Crooks, J. Phys. Chem. B, 2005, 109, 692–704 CrossRef CAS.
- Y. Wang, X. Xie and T. Goodson 3rd, Nano Lett., 2005, 5, 2379–2384 CrossRef CAS.
- L. Balogh, S. S. Nigavekar, B. M. Nair, W. Lesniak, C. Zhang, L. Y. Sung, W. Kariapper, M. S. T. Tan, A. El-Jawahri, M. Llanes, B. Bolton, F. Mamou, A. Hutson, L. Minc and M. K. Khan, Nanomed.: Nanotechnol., Biol. Med., 2007, 3, 281–296 CrossRef CAS.
- A. P. Alivasatos, Science, 1996, 272, 933–937 CrossRef.
- J. M. Costa-Fernández, R. Pereiro and A. Sanz-Medel, TrAC, Trends Anal. Chem., 2006, 25, 207–218 CrossRef.
- S. Svenson and D. A. Tomalia, Adv. Drug Delivery Rev., 2005, 57, 2160–2169.
- K. Sooklal, L. H. Hanus, H. J. Pleoehn and C. J. Murphy, Adv. Mater., 1998, 10, 1083–1087 CrossRef CAS.
- J. R. Lakowicz, I. Gryczynski, Z. Gryczynski and C. J. Murphy, J. Phys. Chem. B, 1999, 103, 7613–7620 CrossRef CAS.
- B. I. Lemon and R. M. Crooks, J. Am. Chem. Soc., 2000, 122, 12886–12887 CrossRef CAS.
- X. C. Wu, A. M. Bittner and K. J. Kern, J. Phys. Chem. B, 2005, 109, 230–239 CrossRef CAS.
- S. K. Gayen, M. Brito, B. B. Das, G. Comanescu, X. C. Liang, M. Alrubaiee, R. R. Alfano, C. Gonzalez, A. H. Byro, D. L. V. Bauer and V. Balogh-Nair, J. Opt. Soc. Am. B, 2007, 24, 3064–3071 Search PubMed.
- S. Ghosh, A. Priyam and A. Saha, J. Nanosci. Nanotechnol., 2009, 9, 6726–6735 CrossRef CAS.
- G. G. Luo, S. Lin, Y. J. Luo, D. Wu and S. N. Zheng, Chin. J. Inorg. Chem., 2005, 21, 1767–1771 CAS.
-
(a) S. Ghosh, A. Priyam, A. Chatterjee and A. Saha, J. Nanosci. Nanotechnol., 2008, 8, 952–957;
(b) Y. Zenga, C. Tanga, G. Tiana, P. Yia, H. Huangb, N. Hub, S. Li, H. Huang, C. Li, B. Lin, X. Yu, Y. Ling and X. Xia, Chem. Eng. J., 2009, 156, 224–227.
-
(a) Y. D. Li, Y. Ding and Z. Y. Wang, Adv. Mater., 1999, 11, 847–850 CrossRef CAS;
(b) K. Yoshino, A. Memon, M. Yoneta, K. Ohmori, H. Saito and M. Ohishi, Phys. Status Solidi B, 2002, 229, 977–980 CrossRef CAS.
-
(a) D. H. Lowndes, C. M. Rouleau, T. Thundat, G. Duscher, E. A. Kenik and S. J. Pennycook, Appl. Surf. Sci., 1998, 127–129, 355–361 CrossRef CAS;
(b) L. Li, Y. Yang, X. Huang, G. Li and L. Zhang, J. Phys. Chem. B, 2005, 109, 12394–12398 CrossRef CAS;
(c) D. Lee, J. Zucker, M. D. Divino, R. F. Austin, R. D. Feldman, K. L. Jones and A. M. Johnson, Appl. Phys. Lett., 1991, 59, 1867–1869 CrossRef CAS.
- Y. W. Jun, C. S. Choi and J. Cheon, Chem. Commun., 2001, 101–102 RSC.
- L. Li, Y. Yang, X. Huang, G. Li and L. Zhang, J. Phys. Chem. B, 2005, 109, 12394–12398 CrossRef CAS.
- D. Lee, J. Zucker, M. D. Divino, R. F. Austin, R. D. Feldman, K. L. Jones and A. M. Johnson, Appl. Phys. Lett., 1991, 59, 1867–1869 CrossRef CAS.
- U. Resch, H. Weller and A. Henglein, Langmuir, 1989, 5, 1015–1020 CrossRef.
- H. B. Huo, L. Dai, C. Liu, L. P. You, W. Q. Yang, R. M. Ma, G. Z. Ran and G. G. Qin, Nanotechnology, 2006, 17, 5912–5915 CrossRef CAS.
- D. H. Lowndes, C. M. Rouleau, T. Thundat, G. Duscher, E. A. Kenik and S. Pennycook, Appl. Surf. Sci., 1998, 127–129, 355–361 CrossRef CAS.
-
(a) U. Resch, H. Weller and A. Henglein, Langmuir, 1989, 5, 1015–1020 CrossRef;
(b) H. B. Huo, L. Dai, C. Liu, L. P. You, W. Q. Yang, R. M. Ma, G. Z. Ran and G. G. Qin, Nanotechnology, 2006, 17, 5912–5915 CrossRef CAS.
- Q. Li, S. Mahendra, D. Y. Lyon, L. Brunet, M. V. Liga, D. Li and P. J. J. Alvarez, Water Res., 2008, 42, 4591–4502 CrossRef CAS.
- Y. Zhang, H. Peng, W. Huanga, Y. Zhou and D. Yana, J. Colloid Interface Sci., 2008, 325, 371–376 CrossRef CAS.
- M. Rai, A. Yadav and A. Gade, Biotechnol. Adv., 2009, 27, 76–83 CrossRef CAS.
- D. Wei, W. Sun, W. Qian, Y. Ye and X. Ma, Carbohydr. Res., 2009, 344, 2375–2382 CrossRef CAS.
- S. Ray, R. Mohan, J. K. Singh, M. K. Samantaray, M. M. Shaikh, D. Panda and P. Ghosh, J. Am. Chem. Soc., 2007, 129, 15042–15053 CrossRef CAS.
- L. K. Adams, D. Y. Lyon and P. J. Alvarez, Water Res., 2006, 40, 3527–3532 CrossRef CAS.
- K. Kasemets, A. Ivask, H. C. Dubourguier and A. Kahru, Toxicol. in Vitro, 2009, 23, 1116–1122 CrossRef CAS.
- V. Aruoja, H. C. Dubourguier, K. Kasemets and A. Kahru, Sci. Total Environ., 2009, 400, 1461–1468 CrossRef.
- N. I. Chalmers, R. J. Palmer, L. Du-Thumm, R. Sullivan, W. Y. Shi and P. E. Kolenbrander, Appl. Environ. Microbiol., 2007, 73, 630–636 CAS.
- M. D. Hirschey, Y. J. Han, G. D. Stucky and A. J. Butler, JBIC, J. Biol. Inorg. Chem., 2006, 11, 663–669 CrossRef CAS.
- J. A. Kloepfer, R. E. Mielke and J. L. Nadeau, Appl. Environ. Microbiol., 2005, 71, 2548–2557 CrossRef CAS.
- Z. T. Jin, H. Q. Zhang, D. Sun, J. Y. Su and H. Sue, J. Food Sci., 2009, 74, M46–M52 CrossRef CAS.
- Z. Lu, C. M. Li, H. Bao, Y. Qiao, Y. Toh and X. Yang, Langmuir, 2008, 24, 5445–5452 CrossRef CAS.
- M. Kosek, C. Bern and R. L. Guerrant, Bull. World Health Organ., 2003, 81, 197–204.
- G. B. Nair, T. Ramamurthy, S. K. Bhattacharya, A. K. Mukhopadhyay, S. Garg, M. K. Bhattacharya, T. Takeda, Y. Takeda and B. C. Deb, J. Infect. Dis., 1994, 169, 1029–1034 CAS.
- L. Turvill, A. Wapnir, A. Wingertzahn, S. Teichberg and G. Farthing, Dig. Dis. Sci., 2000, 45, 946–951 CrossRef.
- E. Salazar, J. Santisteban, E. Chea and M. Gutierrez, N. Engl. J. Med., 2000, 343, 463–467 CrossRef.
- J. D. Williams, Int. J. Antimicrob. Agents, 2001, 18, 295–298 CrossRef CAS.
- M. Stark, L. Liu and C. M. Deber, Antimicrob. Agents Chemother., 2002, 46, 3585–3590 CrossRef CAS.
- S. Pal, Y. K. Tak and J. M. Song, Appl. Environ. Microbiol., 2007, 73, 1712–1720 CrossRef.
- S. Ghosh, A. Datta and A. Saha, Colloids Surf., A, 2009, 355, 130–138.
- P. Thakurta, P. Bhowmik, S. Mukherjee, T. K. Hajra, A. Patra and P. K. Bag, J. Ethnopharmacol., 2007, 111, 607–612 CrossRef.
-
D. S. Reeves, Antibiotic assays. In: P. M. Hawkey, D. A. Lewis (Eds) Medical bacteriology: a practical approach. Oxford: IRL Press, 1989. pp. 195–221 Search PubMed.
-
NCCLS, Approved standards M7–A4. Methods for dilution antimicrobial susceptibility tests for bacteria that grow aerobically. National Committee for Clinical Laboratory Standards, Wayne, Pa, 1997 Search PubMed.
-
NCCLS, Methods for determining bactericidal activity of antimicrobial agents. Approved guideline M26-A. National Committee for Clinical Laboratory Standards, Wayne, Pa, 1999 Search PubMed.
- H. Situ and L. A. Bobek, Antimicrob. Agents Chemother., 2000, 44, 1485–1493 CrossRef CAS.
-
S. V. Gaponenko, “Optical Properties of Semiconductor Nanocrystals”, Cambridge University Press, ( 1998) Search PubMed.
- Y. Tian, T. Newton, N. A. Kotov, D. M. Guldi and J. H. Fendler, J. Phys. Chem., 1996, 100, 8927–8939 CrossRef CAS.
- Z. Yu, J. Li, D. B. O'Connor, L. W. Wang and P. F. Barbara, J. Phys. Chem. B, 2003, 107, 5670–5674 CrossRef CAS.
- S. Patila, A. Sandbergb, E. Heckertc, W. Selfc and S. Seal, Biomaterials, 2007, 28, 4600–4607 CrossRef CAS.
-
P. Singh, Dendrimer-based biological reagents: Preparation and applications in diagnostics, in: J. M. J. Frechet, D. A. Tomalia (ed.), Dendrimer and Other Dendritic Polymers. New York: John Wiley, 2001. pp. 463–84 Search PubMed.
- X. Shi, S. Wang, S. Meshinchi, M. V. Antwerp, X. Bi, I. Lee and J. R. BakerJr, Small, 2007, 3, 1245–1252 CrossRef CAS.
- M. K. Khan, L. D. Minc, S. S. Nigavekar, M. S. T. Kariapper, B. M. Nair, M. Schipper, A. C. Cook, W. G. Lesniak and L. P. Balogh, Nanomed.: Nanotechnol., Biol. Med., 2008, 4, 57–69 CrossRef CAS.
- L. Balogh, D. R. Swanson, D. A. Tomalia, G. L. Hagnauer and A. T. McManus, Nano Lett., 2001, 1, 18–21 CrossRef CAS.
- A. M. Derfus, W. C. W. Chan and S. N. Bhatia, Nano Lett., 2004, 4, 111–118.
- R. A. Hardman, Environ. Health Perspect., 2006, 114, 165–172 CrossRef.
- W. Lesniak, A. U. Bielinska, K. Sun, K. W. Janczak, X. Shi, J. R. BakerJr and L. P. Balogh, Nano Lett., 2005, 5, 2123–2130 CrossRef CAS.
- B. Bonev, J. Hooper and J. Parisot, J. Antimicrob. Chemother., 2008, 61, 1295–1301 CrossRef CAS.
-
(a) P. Luber, E. Bartelt, E. Genschow, J. Wagner and H. Hahn, J. Clin. Microbiol., 2003, 41, 1062–1068 CrossRef CAS;
(b) L. Saiman, J. L. Burns, S. Whittier, J. Krzewinski, S. A. Marshall and R. N. Jones, J. Clin. Microbiol., 1999, 37, 2987–2991 CAS.
-
T. D. Brock, Biology of microorganisms. 2nd ed. Prentice-Hall, Inc., Englewood Cliffs, N.J. 1974 Search PubMed.
- A. J. Verkleij, R. F. Zwaal, B. Roelofsen, P. Comfurius, D. Kastelijn and L. L. Van Deenen, Biochim. Biophys. Acta, Biomembr., 1973, 323, 178–193 CrossRef CAS.
- Z. Oren and Y. Shai, Biopolymers, 1998, 47, 451–463 CrossRef CAS.
|
This journal is © The Royal Society of Chemistry 2011 |
Click here to see how this site uses Cookies. View our privacy policy here.