DOI:
10.1039/C1FO10037H
(Paper)
Food Funct., 2011,
2, 320-327
Amelioration of scopolamine induced cognitive dysfunction and oxidative stress by Inonotus obliquus – a medicinal mushroom
Received
5th March 2011
, Accepted 16th May 2011
First published on 6th June 2011
Abstract
The present study was aimed to investigate the cognitive enhancing and anti-oxidant activities of Inonotus obliquus (Chaga) against scopolamine-induced experimental amnesia. Methanolic extract of Chaga (MEC) at 50 and 100 mg kg −1doses were administered orally for 7 days to amnesic mice. Learning and memory was assessed by passive avoidance task (PAT) and Morris water maze (MWM) test. Tacrine (THA, 10 mg kg −1, orally (p.o)) used as a reference drug. To elucidate the mechanism of the cognitive enhancing activity of MEC, the activities of acetylcholinesterase (AChE), anti-oxidant enzymes, the levels of acetylcholine (ACh) and nitrite of mice brain homogenates were evaluated. MEC treatment for 7 days significantly improved the learning and memory as measured by PAT and MWM paradigms. Further, MEC significantly reduced the oxidative-nitritive stress, as evidenced by a decrease in malondialdehyde and nitrite levels and restored the glutathione and superoxide dismutase levels in a dose dependent manner. In addition, MEC treatment significantly decreased the AChE activity in both the salt and detergent-soluble fraction of brain homogenates. Further, treatment with MEC restored the levels of ACh as did THA. Thus, the significant cognitive enhancement observed in mice after MEC administration is closely related to higher brain anti-oxidant properties and inhibition of AChE activity. These findings stress the critical impact of Chaga, a medicinal mushroom, on the higher brain functions like learning and memory.
1. Introduction
Learning and memory are generated by an experience dependent and long lasting modification of the central nervous system. They involve activation by neurotransmitters, such as acetylcholine (ACh), dopamine and serotonin of the receptor linked enzymes that are responsible for the synthesis of intercellular messengers.1,2 Neuronal loss in the basal forebrain particularly within the septohippocampal acetylcholinergic systems involved in learning and memory processes constitutes a pathological hallmark of Alzheimer's disease (AD). Currently, the treatment of AD is the administration of acetylcholinesterase (AChE) inhibitors that increase the availability of ACh at cholinergic synapses.3,4 However, the nonselectivity of these drugs, limited efficacy, poor bioavailability, adverse cholinergic side effects in the periphery, narrow therapeutic ranges, and heptotoxicity are among the severe limitations to their therapeutic success.5 Hence, novel AChE inhibitors from natural sources could be valuable alternatives in the context of AD treatment.6
Apart from the cholinergic hypothesis, oxidative stress and reactive oxygen species (ROS) have been proposed to be major cause of aging and other aging related neurodegenerative conditions such as AD.7,8 Oxidative damage in the brain and memory impairment mainly results from an imbalance between ROS generation and anti-oxidant enzyme activities. The over production of ROS or decrease in anti-oxidants in the brain can cause lipid peroxidation, nuclear mitochondrial DNA damage and protein oxidation and finally affect the normal functions of organisms.9,10 In search of a model that induces both cholinergic blockade as well as brain oxidative stress, scopolamine, a muscarinic antagonist that induces central cholinergic blockade in rodents4,11 and humans12,13 and also generates oxidative stress14 in the brain, was utilized in the present study to induce amnesia.
Mushrooms are a nutritionally functional food and a source of physiologically beneficial medicines. In Russian traditional medicine, an extract from the mushroom Inonotus obliquus (Chaga) is used as an antitumor and diuretic.15 Moreover, it has been reported that Chaga has therapeutic effects, such as anti-inflammatory, immunomodulatory and hepatoprotective effects.16 There are reports that compounds with an immunomodulatory effect improve the cognitive enhancing activities.17 The medicinal mushroom also produces various classes of secondary metabolites with potent anti-oxidant activity.18 Recently we have reported the in vitro protective effect of 3,4-dihydroxybenzalacetone isolated from Chaga against hydrogen peroxide induced oxidative stress in PC12 cells.19 However, no study has been performed to evaluate whether treatment with Chaga has an anti-amnesic potential against scopolamine-induced cognitive dysfunction. Hence the present study was designed to investigate the effect of Chaga in scopolamine-induced cognitive dysfunction and oxidative stress. Behavioral parameters were evaluated using the passive avoidance task (PAT) and Morris water maze (MWM) in mice. In addition, biochemical parameters like estimation of AChE activity, ACh and nitrite levels and different anti-oxidant enzyme levels were also evaluated in the mice brain homogenates.
2. Materials and methods
2.1. Animals
Male Balb-c mice weighing 25–30g were housed in a group of six, under standard laboratory conditions of temperature (25 ± 1 °C), lighting (on at 0700 h), and relative humidity (50 ± 5%). The food, in the form of dry pellets and water, were available ad libitum. The animal experiments were performed according to internationally followed ethical standards and approval from Niigata University of Pharmacy and Applied Life Sciences.
Methanolic extract of Chaga (MEC).
The dry powder of Chaga (160 g) was extracted with 80% methanol (1.5 L for 3 times) at room temperature.20 The extract was dried up under reduced pressure to give the 80% methanolic extract (10.7 g).
Scopolamine dissolved in saline and given at the dose of 1 mg kg−1 intraperitoneal (i.p). Tacrine (THA) dissolved in water and administered orally at the dose of 10 mg kg−1.21
2.3.
Drugs and chemicals
Inonotus obliquus (persoon) were provided by Isukura Industry Co. Ltd. Tokyo. THA, scopolamine, acetylthiocholine iodide (AChI), 5,5′-dithiobis(2-nitrobenzoic acid) (DTNB) were purchased from Sigma-Aldrich USA. All other chemicals used in the study were of analytical grade. Solutions of the drug and chemicals were freshly prepared before use.
2.4. Passive avoidance task
Training for and testing of passive avoidance performance were carried out in two identical light and dark square boxes.22 The mice were initially placed in the light chamber and 10 s later the door between compartments was opened. When mice entered the dark compartment, the door closed and an electrical foot shock (0.1 mA/10 g body weight) for a period of 2 s was delivered through the stainless steel rods (one trial training). Six mice were used per treatment. Mice received MEC or THA at 60 min before the training trial. After 30 min amnesia was induced in mice with scopolamine (1 mg kg−1i.p). Twenty four hours after the training trial, the mice were again placed in the light compartment. The escape latency to enter the dark compartment was measured. If the mice did not enter the dark compartment within 300 s, the experiment was stopped.
2.5. Morris water maze
A spatial memory test was performed by MWM test. MWM is a circular pool (50 cm diameter and 40 cm height) with a featureless inner surface. The circular pool is made opaque by the addition of milk powder. The pool was divided into four quadrants of equal areas. A white platform (5 cm diameter and 25 cm height) was centered in the one of four quadrants of the pool and submerged 1 cm below the water surface so that it was invisible at water level. The day prior to the experiment was dedicated to swim training for 60 s in the absence of the platform. In the days (day 2, 3, 4 and 5) following, the mice were given single trial sessions each day for 4 consecutive days. During each trial, the time taken to swim to the platform (escape latency) was recorded. Once the mouse located the platform, it was permitted to remain on it for 10 s and then removed from the pool.23 One day after the last trial sessions, mice were subjected to a probe trial session (day 7) in which the platform was removed from the pool; mice were allowed to swim for 120 s to search for it. A record was kept of the swimming time in the pool quadrant where the platform had been previously placed. Six mice were used per treatment. Mice were treated with MEC or THA at 1 h before the training trial (day 2, 3, 4 and 5). After 30 min, amnesia was induced by scopolamine. All mice were tested for spatial memory 30 min after the administration of scopolamine.
2.6. Tissue preparation
Following the behavioral study mice were euthanized. The whole intact brain was carefully removed and placed in an ice chilled petri dish for cleaning. The cerebellum was rapidly removed and the remaining brain was weighed, washed with isotonic saline and homogenized (10% w/v) in 30 mM Na2HPO4, pH 7.6. The homogenates were sonicated briefly on ice and then centrifuged at 20,000 × g at 4 °C for 2 h to recover a salt-soluble fraction (SS). The pellets were re-extracted with an equal volume of 30mM Na2HPO4, pH 7.6, and containing 1% Triton X-100 and the suspensions were centrifuged at 20,000 × g at 4 °C for 2 h to recover a detergent-soluble fraction (DS).24 The supernatant was collected and stored at −20 °C. The protein concentrations were determined by the Bradford assay with bovine serum albumin as standard (0.05–1.00 mg ml−1).
2.7.
AChE assay
AChE activity was determined using the colorimetric assay of Ellman, as previously described.25 Briefly, in the 96 well plates, 25 μl of 15 mM AChI, 75 μl of 3 mM DTNB and 75 μl of 50 mM Tris-HCL, pH 8.0, containing 0.1% BSA, were added and the absorbance was read at 405 nm after 5 min incubation at room temperature. Any increase in absorbance due to the spontaneous hydrolysis of the substrate was corrected by subtracting the rate of the reaction before adding the enzyme. Then, 25 μl of sample (SS and DS fraction) was added and the absorbance was read again after 5 min of incubation at room temperature. All determinations were carried out twice and in triplicate.
2.8. Assay of ACh
ACh was determined by the method of Hestrin.26 Briefly, 10% brain homogenate in cold saline was prepared on ice. The aliquots (0.8 ml) of brain homogenate were mixed with 1.4 ml distilled water, 0.2 ml of 1.5 mM physostigmine, and 0.8 ml of 1.84 M trichloroacetic acid blending adequately. After centrifugation, 1 ml of each supernatant was added to 1ml of basic hydroxylamine. The mixture was incubated for 15 min at 25 °C and then 0.5 ml of 4 M HCl and 0.5 ml of 0.37 M FeCl3 were added. Absorbance was read at 540 nm, and calibrated with standard (ACh – 0.2 μM ml−1).
2.9. Estimation of nitrite
The accumulation of nitrite in the supernatant, an indicator of the production of nitric oxide, was determined by colorimetric assay using Greiss reagent as described by Green et al.27 Equal volumes of supernatant and Greiss reagent (1% sulphanilamide, 2% O-phosphoric acid and naphthyl ethylene diamine dihydrochloride 0.1%) were mixed, the mixture incubated for 10 min at room temperature in the dark and the absorbance determined at 540 nm spectrophotometrically. The concentration of nitrite in the supernatant was determined from sodium nitrite standard curve and expressed as μmol per mg protein.
The quantitative measurement of MDA end product of lipid peroxidation in brain homogenate was performed according to the method of Ohkawa et al.28 Briefly, to each 50 μl of homogenate sample, 8.1% sodium dodecyl sulphate, 0.04% thiobarbituric acid in 20% acetic acid (pH 3.5) and distilled water were added. The mixture was then incubated at 95 °C for 1 h. After cooling with tap water, 15
:
1 (v/v) n-butanol-pyridine were added, and the mixture was shaken vigorously. After centrifugation at 1000 g for 10 min, the absorption of the upper organic layer was determined at 530 nm by spectrophotometer.
2.11. Reduced glutathione (GSH)
GSH estimation was performed using the method of Griffith.29 To the required amount of homogenate, 0.7 ml of 0.3 mM l−1 nicotinamide adenine dinucleotide phosphate (NADPH), 0.1 ml of 6 mM l−1DTNB, and 0.48 U glutathione reductase were combined, and the absorbance of 5-thio-2-nitrobenzoic acid was read at 412 nm. A standard curve was obtained with standard GSH. The level of GSH was expressed as micrograms per milligram of protein.
GSSG estimation was performed using the method of Griffith.29 The required amount of homogenate was incubated at room temperature with 0.005 mL of 2 mol l−12-venyl pyridine for 1 h. Following incubation, 0.4 ml of 0.5 mmol l−1NADPH, 0.1 ml of 6 mol l−1DTNB, and 0.48 U glutathione reductase were added and measured at 412 nm. A standard curve was obtained with standard GSSG. The level of GSSG was expressed as micrograms per milligram of protein.
2.13.
Glutathione peroxidase (GPx)
The activity of GPx was determined at 340 nm in reaction medium containing 50 mM K, Na-phosphate buffer (pH 7.4 at 30 °C), 1 mM EDTA, 0.12 mM NADPH, 0.85 mM GSH, 0.5 unit ml−1glutathione reductase and 0.2 mM tert-butyl hydrogenperoxide as a substrate.30 The results were expressed as unit/mg protein.
2.14.
Superoxide dismutase (SOD)
Into 2.5 ml of a 50 mM sodium phosphate buffer (pH 7.0), 0.1 ml of 3 mM xanthine, 3 mM EDTA, WST solution and the sample were added. The reaction was initiated by adding a xanthine oxidase solution.31 The absorbance change at 438 nm (WST-1) was noted. The results were expressed as unit/mg protein.
2.15. Statistical analysis
The results are given as mean ± S.E.M. The data obtained was analysed by one way analysis of variance (ANOVA) followed by Tukey's post hoc test. Differences were considered significant at the 5% level.
3. Results
3.1. Effects of MEC on memory impairment induced by scopolamine in PAT
During acquisition trials, latency times were not different among the experimental groups of mice by the acute treatment with MEC. For retention trials, the ANOVA followed by Tukey's test revealed significant differences for latencies time in the acute treatment. The step-through latency of scopolamine-treated mice was significantly (P < 0.001) shorter than that of the control group. In THA (positive control group) with scopolamine-treated group step-through latencies was significantly (P < 0.001) higher than that of the scopolamine-treated group (Fig. 1A). Moreover, the shorter step-through latencies induced by scopolamine were significantly attenuated by MEC 50 (P < 0.05) and 100 mg kg−1 (P < 0.001) treatment.
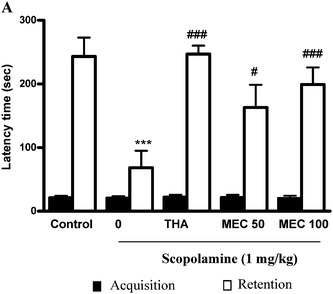 |
| Fig. 1 Effects of MEC on scopolamine-induced memory impairment in the PAT response in mice. For the study on the effect of MEC on the scopolamine-induced memory deficit model, mice were administered MEC (50 and100 mg kg−1) or THA (10 mg kg−1, p.o., positive control) 1 h before the acquisition trial. Memory impairment was induced by scopolamine treatment (1 mg kg−1, i.p.) and acquisition trials were carried out 30 min after scopolamine treatment. At 24 h after the acquisition trials, retention trials were carried out. Data represents mean ± S.E.M (n = 6). ***P < 0.001, statistically different from control group. ###P < 0.001, #P < 0.05 statistically different from scopolamine-treated group. | |
3.2. Effects of MEC on memory impairment induced by scopolamine in MWM test
The effect of MEC on spatial learning was evaluated using the MWM task. As shown in Fig. 2A, the scopolamine-treated group exhibited longer escape latencies throughout training compared with the control group. MEC treatment significantly shortened the increased escape latency by scopolamine. THA also significantly reduced escape latencies compared with those in the scopolamine-treated group. On the day following the final day of training trial sessions, swimming times within the target quadrant in the scopolamine-treated group were significantly shorter (P < 0.01) than those in the control group (Fig. 2B). Moreover, the shortened swimming time within the platform quadrant induced by scopolamine was significantly increased by MEC 100 mg kg−1 (P < 0.01) and THA (P < 0.01) treatment.
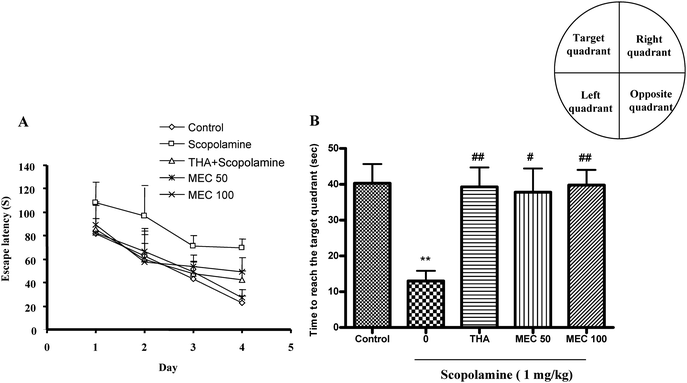 |
| Fig. 2 Effect of MEC on performance during training trial sessions (A) and probe trial sessions (B) using MWM in scopolamine-induced memory deficit mice. At 60 min before the training trial session, MEC (50 and100 mg kg−1) or THA (10 mg kg−1, p.o., positive control) was administered to mice. Memory impairment was induced by scopolamine (1 mg kg−1, i.p.) at 30 min after MEC or THA administration. The training trial and the probe trial sessions were conducted as described in materials and methods. Data represents mean ± S.E.M (n = 6). **P < 0.01, statistically different from control group. ##P < 0.01, #P < 0.05 statistically different from scopolamine-treated group. | |
3.3. Effects of MEC on AChE assay
Fig. 3A and B shows the effect of MEC on AChE levels in both SS and DS fractions of brain homogenates. Acute administration of MEC 100 mg kg−1 (P < 0.001) resulted in a significant decrease in AChE-specific activity for both SS and DS fractions compared with those of the scopolamine-treated group. There was a significant increase in AChE activity in both the SS (P < 0.001) and DS (P < 0.01) fraction of the scopolamine-treated group. The standard drug THA also significantly (P < 0.001) reduced the AChE activity in both the SS and DS fractions.
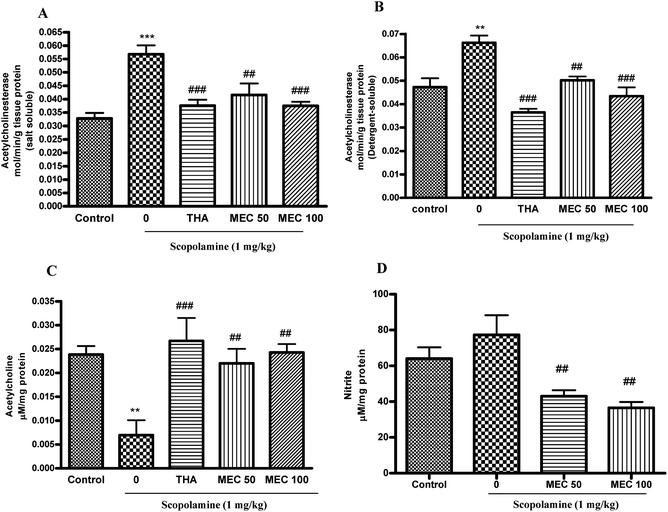 |
| Fig. 3 The effect of MEC (50 and 100 mg kg−1) administration for 7 days on AChE activity in SS fraction (A) and DS fraction (B), ACh level (C) and nitrite level (D) of mice brain homogenates. Data represents mean ± S.E.M (n = 6). ***P < 0.001, **P < 0.01 statistically different from control group. ###P < 0.001, ##P < 0.01 statistically different from scopolamine-treated group. | |
3.4. Effects of MEC on ACh level
As Fig. 3C shows, scopolamine-induced a significant decrease of ACh levels (P < 0.01) in the brain homogenates and the results were consistent with a previous report by Saito et al.32 At the same time, MEC significantly prevented the decrease (50, 100 mg kg−1 (P < 0.01)) and maintained the ACh levels as normal control. THA as a reference drug also significantly (P < 0.001) inhibited the decrease of ACh levels.
3.5. Effects of MEC on nitrite level
There was no significant increase in nitrite levels in the scopolamine-treated group as compared to that in the control group. However, there was a dose dependent decrease in nitrite levels observed in the MEC treated group (P < 0.01) compared with the scopolamine-treated one. Similiarly, Kuo et al. reported the in vitro nitrite inhibitory property of inotilone, a component from Inonotus, against LPS-induced nitritive stress.33
3.6. Anti-oxidant potential of MEC on scopolamine-induced oxidative stress
To further elucidate the biochemical mechanism of the anti-amnesic activity of MEC, its effects on different anti-oxidant enzymes and cellular GSH levels were determined. The treatment of mice with scopolamine resulted in a significant increase in MDA levels (Fig. 4A) and significant decreases in GSH (Table 1) and SOD (Fig. 4C) levels, while the activity of GPx was also reduced but the difference was not statistically significant (Fig. 4B) and the results were consistent with earlier reports.34 We also found a significant increase (P < 0.05) in the GSSG/GSH ratio in the scopolamine-treated group mice. The acute treatment of amnesic mice with MEC 50 mg kg−1 (P < 0.05) and MEC 100 mg kg−1 (P < 0.01) significantly preserved the activities of GSH and SOD. MEC treatment also preserved the GPx activity significantly (P < 0.05) as compared to the scopolamine treated group. There was a significant decreased in the MDA levels in MEC 50 mg kg−1 (P < 0.05) and MEC 100 mg kg−1 (P < 0.01) groups as compared with those of the scopolamine-treated group, as shown in Fig. 4A. Further treatment with MEC 50 mg kg−1 and 100 mg kg−1 (P < 0.05) significantly reduced the GSSG/GSH ratio in the brain homogenate.
Table 1 The effects of MEC (50 and100 mg kg−1) treatment on GSH and GSSG levels in scopolamine-induced memory deficit mice.a
Groups
|
Glutathione (reduced) (μg mg protein−1) |
Glutathione (oxidized) (μg mg protein−1) |
GSSG/Total GSH ratio |
**
P < 0.01, *P < 0.05 statistically different from control group. ###P < 0.001, ##P < 0.01, #P < 0.05 statistically different from scopolamine-treated group.
|
Control |
2.070 ± 0.297 |
0.931 ± 0.282 |
0.280 ± 0.053 |
Scopolamine
|
0.521 ± 0.136** |
1.586 ± 0.437 |
0.704 ± 0.076* |
MEC 50 + Scopolamine |
2.004 ± 0.320## |
1.142 ± 0.375 |
0.341 ± 0.102# |
MEC + 100 + Scopolamine |
2.340 ± 0.301### |
1.547 ± 0.581 |
0.311 ± 0.093# |
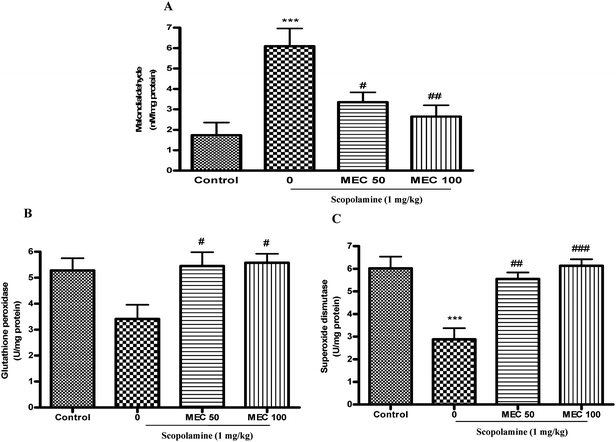 |
| Fig. 4 Effects of MEC (50 and 100 mg kg−1) treatment on the concentrations of MDA (A), the activities of GPx (B) and SOD (C) in scopolamine-induced memory deficit mice. ***P < 0.001, statistically different from control group. ###P < 0.001, ##P < 0.01, #P < 0.05 statistically different from scopolamine-treated group. | |
4. Discussion
In the present study, we examined the neuroprotective effects of MEC by biochemical and behavioral evaluations using a pharmacologically induced amnesia by scopolamine in mice. We found that treatment with MEC attenuated memory impairment induced by scopolamine in behavioral paradigms. Furthermore, in biochemical investigations, MEC significantly decreased the AChE activity and restored the levels of ACh. It also exhibited antioxidative and nitritive properties, as evidenced by the significant protection of different anti-oxidant enzymes and a decrease in nitrite levels.
Oxyradical-induced damage to macromolecules (lipids, proteins and nucleic acids, etc.) is considered as an important factor in the acceleration of aging and age-related neurodegenerative disorders such as AD.35 The anti-oxidant system uses reduced GSH, the most abundant non-protein thiol, which buffers free radicals in brain tissue.36 It functions as an electron-donating substrate of GPx to eliminate cellular H2O2 and organic peroxides. In the reaction, GSH is oxidized to form glutathione disulfide.37 Depletion of the cellular level of GSH not only slows H2O2 clearance and promotes OH radical formation, which is the species that is most toxic to the brain and that leads to more oxidant load and consequently more oxidative damage, but also disturbs cellular redox regulation. The increase in H2O2 induces the peroxidation of polyunsaturated fatty acids leading to the formation of MDA, which is the end product of lipid peroxidation.38 Treatment with MEC not only inhibited the reduction in SOD activity induced by scopolamine but also elevated as equal to normal control mice. In the brain of AD patients, SOD activity was significantly lower than that of the non-demented controls. Although there are still conflicting reports associated with SOD activity in AD, SOD mimetics have come to the forefront of antioxidative therapeutics of neurodegenerative diseases.39
El-Sherbiny et al. reported that memory impairment induced by scopolamine is associated with altered status of brain oxidative stress in rats.14 In our experimental conditions, the treatment with scopolamine resulted in a significant decrease in both cellular GSH and SOD activities; the activity of GPx also decreased although the changes were not statistically significant. GPx reduces toxic radicals using GSH as a substrate, subsequent to the oxidation of GSH to GSSG. GSSG is in turn reduced again to GSH by glutathione reductase at the expenses of NAPDH, forming a redox cycle.40 We suggest that the restoration of the activities of GPx and GSH by the MEC might promote scavenging of free radicals using recycled GSH from GSSG. From the above results, it could be postulated that MEC treatment prevented cerebral oxidative damage induced by scopolamine through not only the scavenging of ROS but also the modulation of anti-oxidant enzymes such as cellular GSH and SOD levels. The result supported the previous report in which Chaga showed strong anti-oxidant activity in in vitro models.41
In the PAT study, scopolamine shortened the step-through latency demonstrating marked memory deterioration in mice. In the present study, reduced step-through latency by scopolamine was recovered to approximately 57% and 72% by MEC treatment. The percentage recovery of the THA-treated group was 77%. In the MWM learning task that is used to assess hippocampal-dependent spatial learning ability,42 escape latencies extended by scopolamine treatment were significantly shortened by MEC treatment in four days of training trial sessions. At the probe trial session, MEC at the dose of 50 and 100 mg kg−1 increased swimming time within the zone of the previously placed platform to approximately 65% and 67% respectively as compared with that of the scopolamine group, as did THA. Therefore, it appears reasonable to conclude MEC reverses the memory deficits induced by scopolamine.
It has been suggested that the impairments in learning, memory and behavior observed in patients with dementia are caused, at least in part, by changes within the cholinergic system.43,44 Cholinergic transmission is terminated mainly by ACh degradation mediated by the enzyme AChE. It is currently believed that the activity of this enzyme could affect the underlying processes in AD.45 Two major isoforms of AChE are globular monomer (G1) protein and globular tetramer (G4) of the same monomer subunit. The G1 isoform is reported to be present in the cytoplasm of neuronal cells, whereas the G4 isoform is predominantly membrane-bound.46 The experimental procedure of Das et al. was followed in the present study to measure each isoform in different cellular fractions. Results showed that MEC treatment significantly attenuated the AChE (G1 and G4 isoforms) levels in both SS and DS brain homogenates and the effect was comparable to that of the reference compound THA. Therefore, the results suggest that MEC treatment is effective in attenuating AChE levels against scopolamine-induced amnesia. Further we analyzed the ACh levels in the brain homogenate of memory deficient mice. We observed that, ACh levels were significantly reduced in scopolamine-treated mice but treatment with MEC restored the reduced ACh level as did THA.
In addition to this Chaga classified as natural polymer pigments of melanins.47 The melanin character of pigments isolated from Chaga was confirmed by the complex analysis of their physicochemical properties.48 Fungal melanins exhibit high anti-oxidant, immunomodulatory and anti-inflammatory properties with low toxicity.49 Recent studies have demonstrated the anti-inflammatory, immunostimulatory and antioxidant potential of Chaga.50–52 Hence these properties of Chaga may also contribute to its memory improving action against scopolamine-induced memory impairment and oxidative damage.
In summary, the present study has shown that MEC are effective in ameliorating scopolamine-induced behavioral alterations, cholinergic dysfunctions and oxidative-nitrative stress. The observed beneficial effects of Chaga in spatial memory processing may be due to its ability to prevent neuronal damage caused by scopolamine, possibly through its anti-oxidant mechanism and also by its inhibitory action on AChE. Rigorous verification of such properties might better clarify the mechanism of action of Chaga and support the rationale of clinical use of this traditional formula in neurodegenerative disorders.
Acknowledgements
This study was supported by a grant to TK from the Promotion and Mutual Aid Corporation for Private Schools. The authors thank the Rotary Yoneyama Scholarship Association for the financial assistance to V. V. G.
Reference
-
I. Izquierdo, G. Lynch, J. L. McGaugh, and N. M. Weinberger, Neurobiology of learning and memory. The Guilford Press. New York 1984, p. 333–350 Search PubMed.
- L. Jodar and H. Kaneto, Synaptic plasticity: stairway to memory, Jpn. J. Pharmacol., 1995, 68, 359–387 CrossRef CAS.
- D. Braida, E. Paladini, P. Griffini, M. Lamerti, A. Maggi and M. Sala, An inverted U shaped curve for heptylphysostigmine on radial maze performance in rats: comparison with other cholinesterase inhibitors, Eur. J. Pharmacol., 1996, 302, 13–20 CrossRef CAS.
- C. Bejar, R. H. Wang and M. Weinstock, Effect of rivastigmine on scopolamine induced memory impairment in rats, Eur. J. Pharmacol., 1999, 383, 231–240 CrossRef CAS.
- G. M. Bores, F. P. Huger, W. Petko, A. E. Mutlib, F. Camacho and D. K. Rush, Pharmacological evaluation of novel Alzheimers disease therapeutics: acetylcholinesterase inhibitors related to galantamine, J. Pharm. Exp. Ther., 1996, 277, 728–738 CAS.
- Y. Ren, P. J. Houghton, R. C. Hider and M. L. Howes, Novel diterpenoid acetylcholinesterase inhibitors from, Salvia miltiorhiz, Planta Med., 2004, 70, 201–204 Search PubMed.
- C. W. Olanow, A radical hypothesis for neurodegeneration, Trends Neurosci., 1993, 16, 439–444 CrossRef CAS.
- M. Valko, D. Leibfritz, J. Moncol, M. E. Cronin, M. Mazur and J. Telser, Free radicals and antioxidants in normal physiological functions and human diseases, Int. J. Biochem. Cell Biol., 2007, 39, 44–84 CrossRef CAS.
- C. M. Maier and P. H. Chan, Role of superoxide dismutase in oxidative damage and neurodegenerative disorders, Neuroscientist, 2002, 8, 323–334 CrossRef CAS.
- M. A. Lovell and W. R. Markesbery, Oxidative damage in mild cognitive impairment and late stage Alzheimer's disease, Nucleic Acids Res., 2007, 35, 7497–7504 CrossRef CAS.
- M. Ohno and S. Watanabe,
D-Cycloserine, a glycine site agonist, reverses working memory failure by hippocampal muscarinic receptor in rats, Eur. J. Pharmacol., 1996, 318, 267–271 CrossRef CAS.
- R. W. Jones, K. A. Wesnes and J. Kirby, Effects of NMDA modulation in scopolamine dementia, Ann. N.Y. Acad. Sci., 1991, 640, 241–244 CAS.
- K. A. Wesness, P. M. Simpson, L. White, S. Pinker, G. Jertz and M. Murphy, Cholinesterase inhibition in the scopolamine model of dementia, Ann. N.Y. Acad. Sci., 1991, 640, 268–271.
- D. A. El-Sherbiny, A. E. Khalifa, A. S. Attia and E. E. S. Eldenshary,
Hypericun perforatum extract demonstrates antioxidant properties against elevated rat brain oxidative status induced by amnestic dose of scopolamine, Pharmacol., Biochem. Behav., 2003, 76, 525–533 CrossRef CAS.
- N. L. Huang, Inonotus obliquus, Edible Fungi of China, 2002, 21, 7–8 Search PubMed.
- P. W. Solomon and L. W. Alexander, Therapeutic effects of substances occurring in higher Basidiomycetes mushrooms: a modern perspective, Crit. Rev. Immunol., 1999, 19, 65–96.
- V. G. Vijayasree, A. T. Rajarajan, M. Vasudevan, A. D. Taranalli, W. Kenichi and K. Tetsuya,
Ocimum sanctum Linn. leaf extracts inhibit acetylcholinesterase and improve cognition in rats with experimentally induced dementia, J. Med. Food., 2011 Search PubMed (in press).
- I. K. Lee, Y. S. Kim, Y. W. Jang, J. Y. Jung and B. S. Yun, New antioxidant polyphenols from the medicinal mushroom, Bioorg. Med. Chem. Lett., 2007, 17, 6678–6681 CrossRef CAS.
- Y. Nakajima, H. Nishida, Y. Nakamura and T. Konishi, Prevention of hydrogen peroxide-induced oxidative stress in PC12 cells by 3,4-dihydroxybenzalacetone isolated from Chaga (Inonotus obliquus (persoon) Pilat), Free Radical Biol. Med., 2009, 47, 1154–1161 CrossRef CAS.
- Y. Nakajima, Y. Sato and T. Konishi, Antioxidant small phenolic ingredients in Inonotus obliquus (persoon) Pilat (Chaga), Chem. Pharm. Bull., 2007, 55, 1222–1226 CrossRef CAS.
- K. Jung, B. Lee, S. J. Han, J. H. Ryu and D. H. Kim, Mangiferin ameliorates scopolamine-induced learning deficits in mice, Biol. Pharm. Bull., 2009, 32, 242–246 CrossRef CAS.
- S. R. Kim, S. Y. Hwang, Y. P. Jang, M. J. Park, G. J. Markelonis and T. H. Oh, Protopine from Corydalis ternata has anticholinesterase and antiamnesic activities, Planta Med., 1999, 65, 218–221 CrossRef CAS.
- S. R. Kim, S. Y. Kang, K. Y. Lee, S. H. Kim, G. J. Markelonis and T. H. Oh, Anti amnestic activity of E-p-methoxycinnamic acid from, Cognit. Brain Res., 2003, 17, 454–461 Search PubMed.
- A. Das, M. Dikshit and C. Nath, Role of molecular isoforms of acetylcholinesterase in learning and memory functions, Pharmacol., Biochem. Behav., 2005, 81, 89–99 CrossRef CAS.
- G. L. Ellman, K. D. Courtney, V. Jr.Andres and R. M. Featherstone, A new and rapid colorimetric determination of acetyl cholinesterase activity, Biochem. Pharmacol., 1961, 7, 88–95 CrossRef CAS.
- S. Hestrin, The reaction of acetylcholine and other carboxylic acid derivatives with hydroxylamine, and its analytical application, J. Biol. Chem., 1949, 180, 249–261 CAS.
- L. C. Green, D. A. Wagner, J. Glogowski, P. L. Skipper, J. S. Wishnok and S. R. Tannenbaum, Analysis of nitrate, nitrite and {15N} nitrate in biological fluids, Anal. Biochem., 1982, 126, 131–138 CrossRef CAS.
- H. N. Ohkawa, Ohishi and K. Yagi, Assay for lipid peroxidation in animal tissues by thiobarbituric acid reaction, Anal. Biochem., 1979, 95, 351–358 CAS.
- O. W. Griffith, Determination of glutathione and glutathione sulfide using glutathione reductase and 2-Vinyl pyridine, Anal. Biochem., 1980, 106, 207–212 CAS.
- V. Z. Lankin, A. K. Tikhase, A. L. Kovalevskaya, V. V. Lemeshko and A. M. Vikhert, Age related changes in the glutathione S transferease and glutathione peroxidase activity of rat liver cytosol, Dokl. Akad. Nauk SSSR, 1981, 261, 1467–1470 CAS.
- U. Hiroyuki, K. Daisuke, M. Susumu and S. Masayoshi, Spectrometric assay for superoxide dismutase based on the reduction of highly water soluble tetrazolium salts by xanthine oxidase, Biosci., Biotechnol., Biochem., 1999, 63, 485–488 CrossRef CAS.
- K. Saito, S. Honda, A. Tobe and I. Yanagiya, Effects of bifemelane hydrochloride (MCI-2016) on acetylcholine level reduced by scopolamine, hypoxia and ischemia in the rats and mongolian gerbils, Jpn. J. Pharmacol., 1985, 38, 375–380 CrossRef CAS.
- Y. C. Kuo, C. S. Lai, J. M. Wang, V. Badmaev, K. Nagabhushanam, C. T. Ho and M. H. Pan, Differential inhibitory effects of inotilone on inflammatory mediators, inducible nitric oxide synthase and cyclooxygenase-2, in LPS-stimulated murine macrophage, Mol. Nutr. Food Res., 2009, 53, 1386–1395 CrossRef CAS.
- V. V. Giridharan, R. A. Thandavarayan, S. Sato, K. M. Ko and T. Konishi, Prevention of scopolamine induced memory deficits by schisandrin B, an antioxidant lignan from Schisandra chinensis in mice, Free Radical Res., 2011 DOI:10.3109/10715762.2011.571682.
- R. Liu, I. Y. Liu, X. Bi, R. F. Thompson, S. R. Doctrow and B. Malfroy, Reversal of age related learning deficits and brain oxidative stress in mice with superoxide dismutase/catalase mimetics, Proc. Natl. Acad. Sci. U. S. A., 2003, 100, 8526–8531 CrossRef CAS.
- R. Dringen, Metabolism and functions of glutathione in brain, Prog. Neurobiol., 2000, 62, 649–671 CrossRef CAS.
- Y. Sun, Free radicals, antioxidant enzymes, and carcinogenesis, Free Radical Biol. Med., 1990, 8, 583–599 CrossRef CAS.
- C. W. Olanow and W. G. Tatton, Etiology and pathogenesis of Parkinsons disease, Annu. Rev. Neurosci., 1999, 22, 123–144 CrossRef CAS.
- K. Pong, Oxidative stress in neurodegenerative disease: therapeutic implications for superoxide dismutase mimetics, Expert Opin. Biol. Ther., 2003, 3, 127–139 Search PubMed.
- S. C. Lu, Regulation of hepatic glutathione synthesis: current concepts and controversies, FASEB J., 1999, 13, 1169–1183 CAS.
- L. Liang, Z. Zhang and H. Wang, Antioxidant activities of extracts and sub fractions from, Int. J. Food Sci. Nutr., 2009, 60, 175–184 CrossRef CAS.
- C. A. Barnes, W. Danysz and C. G. Parsons, Effects of the uncompetitive NMDA receptor antagonist memantine on hippocampal long term potentiation, short term exploratory modulation and spatial memory in awake, freely moving rats, Eur. J. Neurosci., 1996, 8, 565–571 CrossRef CAS.
- A. Blokland, Acetylcholine: a neurotransmitter for learning and memory?, Brain Res. Rev., 1995, 21, 285–300 CrossRef CAS.
- V. Fodale, D. Quattrone, C. Trecroci, V. Caminiti and L. B. Santamaria, Alzheimer's disease and anaesthesia: implications for the central cholinergic system, Br. J. Anaesth., 2006, 97, 445–452 CrossRef CAS.
- C. G. Balllard, N. H. Greig, A. L. Guillozet-Bongaarts, A. Enz and S. Darvesh, Cholinesterases: roles in the brain during health and disease, Curr. Alzheimer Res., 2005, 2, 307–318 CrossRef.
- J. Massoulie, L. Pezzementi, S. Bon, E. Krejci and F. M. Vallette, Molecular and cellular biology of cholinesterases, Prog. Neurobiol., 1993, 41, 31–91 CrossRef CAS.
- V. G. Babitskaia, V. V. Shcherba and N. V. Ikonnikova, Melanin complex of the fungus Inonotus obliquus, Prikl. Biokhim. Mikrobiol., 2000, 36, 439–444 Search PubMed.
- A. Jarosz, M. Skorska, J. Rzymowska, J. Kochmańska-Rdest and E. Malarczyk, Effect of the extracts from fungus Inonotus obliquus on catalase level in HeLa and nocardia cells, Acta Biochim. Pol., 1990, 37, 149–151 CAS.
- M. Shashkina, P. N. Shashkin and A. V. Sergeev, Chemical and medicobiological properties of Chaga, Pharmaceu. Chem. J., 2006, 40, 37–44 Search PubMed.
- S. Y. Choi, S. J. Hur, C. S. An, Y. H. Jeon, Y. J. Jeoung, J. P. Bak and B. O. Lim, Anti-inflammatory effects of Inonotus obliquus in colitis induced by dextran sodium sulfate, J. Biomed. Biotechnol., 2010, 2010, 943516 Search PubMed.
- D. P. Won, J. S. Lee, D. S. Kwon, K. E. Lee, W. C. Shin and E. K. Hong, Immunostimulating activity by polysaccharides isolated from fruiting body of Inonotus obliquus, Mol. Cells, 2011, 31, 165–173 CrossRef CAS.
- L. Liang, Z. Zhang and H. Wang, Antioxidant activities of extracts and subfractions from Inonotus Obliquus, Int. J. Food Sci. Nutr., 2009, 60, 175–184 CrossRef CAS.
|
This journal is © The Royal Society of Chemistry 2011 |