Direct oxidation of benzene to phenol by N2O over meso-Fe-ZSM-5 catalysts obtained via alkaline post-treatment
Received
14th April 2011
, Accepted 17th June 2011
First published on 8th August 2011
Abstract
Fe-ZSM-5
zeolites with uniform crystal size and shape were synthesized by a hydrothermal method, and these parent zeolites were desilicated by an alkaline-treatment method to obtain mesoporous Fe-ZSM-5 (meso-Fe-ZSM-5) zeolites. Both Fe-ZSM-5 and meso-Fe-ZSM-5 zeolites were characterized by XRD, N2 adsorption–desorption, ICP-AES, SEM, TEM, UV-vis, and TGA techniques and as catalysts they were used in the direct oxidation of benzene to phenol (BTOP) by N2O as oxidant. The meso-Fe-ZSM-5 catalysts show a much higher catalytic activity and stability, compared to the parent Fe-ZSM-5 catalysts. A comparison study on the uptakes of benzene in the parent and alkali-treated zeolites indicates that the internal mass-transfer limitations of the meso-Fe-ZSM-5 zeolites are significantly improved due to the introduction of intracrystalline mesoporosity, resulting in a better catalytic activity and stability in the BTOP reaction. Desilication in the alkaline medium of Fe-ZSM-5 crystals enables a more efficient utilization of the zeolite in the BTOP reaction that is strongly affected by diffusional limitations.
I. Introduction
Phenol is an important organic chemical intermediate and raw material, mainly used in the manufacture of phenolic resin, bisphenol A, and caprolactam. At present, the so-called cumene process is used for the production of phenol on an industrial scale.1 However, this process has some fundamental and inevitable disadvantages: acetone as byproduct produced in a 1
:
1 stoichiometry, which in terms of market demand is much smaller than phenol. Therefore, the productivity of phenolvia this technology is influenced by the market price of the co-produced acetone.
Compared with the industrialized process for phenol production, the direct oxidation of benzene to phenol (BTOP) has economical and environmentally friendly advantages, which have attracted researchers' attention. At present, the so-called Alphox process,2 in which N2O and Fe-ZSM-5 are used as oxidant and catalyst, respectively, is considered as the most promising technology for the direct oxidation of BTOP.2 In the Alphox process the selectivity for phenol is nearly 100%.3–5 However, the demonstration of the Alphox process on a pilot scale indicates that the used Fe-ZSM-5 catalysts are easily deactivated.6,7 This is due to the fact that the zeolite Fe-ZSM-5 is a microporous material, in which there are strong mass transfer limitations for the reactant benzene and the product phenol, and under the applied reaction conditions, the formation of coke leads to micropore blockage in the zeolite, resulting in rapid catalyst deactivation.6–10
In order to improve the mass-transfer limitations of microporous zeolites, post-treatments such as water vapor- and acid-treatment for dealumination have been used to create mesopores in zeolite crystals.11–14 However, an experimental investigation has proven that dealuminated species can block the interconnections between created mesopores and zeolite micropores.15 This cannot, in principle, improve the mass-transport properties of zeolites.
Recently, a method, the so-called alkaline treatment,15–23 has been used to selectively extract the framework silicon to create mesopores in zeolite crystals where the formed mesopores are connected with micropores. Groen et al.15 used a TEOM (Tapered Element Oscillating Microbalance) technique24,25 to make a comparison study on the diffusion of neopentane as a probe in the parent zeolite ZSM-5 and its desilicated zeolite. Their results showed that the characteristic diffusion length in the mesoporous zeolite crystals was dramatically shortened, resulting in an approximately 2 orders of magnitude faster diffusion of neopentane in the majority of the micropores.15 Desilication in the alkaline medium of microporous zeolite crystals, presenting a homogeneous distribution of framework aluminium, originates in an accessible interconnected network of intracrystalline mesopores and preserved crystallinity. In mesozeolites obtained by the alkaline treatment, the preserved micropores can serve as “micro-reactors” offering active species or adsorption sites with molecular-sieving properties while the created mesopores are beneficial to molecular diffusion.17 Therefore, the alkaline treatment is an efficient manner to improve the mass-transfer properties of microporous zeolites.
Up to now, however, research on the alkaline treatment for improving the mass-transfer properties of Fe-ZSM-5 and the application of the resulting meso-Fe-ZSM-5 in the BTOP reaction has been very limited.22 Additionally, almost no work has been conducted on identifying whether or not the Fe species after the alkaline treatment of Fe-ZSM-5 would be changed, although it has been widely accepted that the extra-framework dinuclear Fe species in the zeolite micropores are the most active sites for the BTOP reaction.26–28 Moreover, the state of the Fe species in Fe-ZSM-5 is strongly dependent on the method of catalyst preparation as well as on the post-treatment conditions, including the activation procedure.29
In the current work, Fe-ZSM-5 crystals with uniform size and shape were synthesized by a hydrothermal method, and these parent zeolites were desilicated by an alkaline-treatment method to obtain meso-Fe-ZSM-5 zeolites. Both Fe-ZSM-5 and meso-Fe-ZSM zeolites were characterized by XRD, N2 adsorption–desorption, ICP-AES, SEM, TEM, UV-vis, and TGA techniques. A comparison study was conducted on the catalytic oxidation of BTOP by N2O over Fe-ZSM-5 and meso-Fe-ZSM-5 zeolites. The differences in the catalytic properties between these two catalysts were elucidated. Finally, the applicability of the obtained meso-Fe-ZSM-5 catalysts in the BTOP reaction is anticipated.
II. Experimental
A Preparation of Fe-ZSM-5
All reagents with AR purity (analytical reagent grade) were purchased and used as received without further purification. Fe-ZSM-5 crystals were synthesized by a hydrothermal method as described in the literature30 with some modification. The sodium waterglass (Aldrich, 26.5 wt% SiO2 and 10.6 wt% Na2O) was added into the basic solution of the organic template TPABr (Tianjin Guangfu Fine Chemical Research Institute) and was hydrolyzed at room temperature. The solution containing the dissolved Al2(SO4)3 (Shanghai Xing Chemical Co., Ltd.) and Fe(NO3)3 (Sinopharm Chemical Reagent Co., Ltd.) was dropwise added into the above prepared solution. Nominal molar ratios were TPABr/Si = 0.2, NaOH/Si = 0.1, H2O/Si = 25, Si/Al = 25, and Fe/Si = 0.005. Subsequently, the formed gel was transferred to a Teflon-lined autoclave and kept in an oven at 380 K for 2 d and then at 450 K for 1 d. Afterwards the crystals were filtered, washed with demineralized water, dried at 353 K, ion-exchanged with a 0.5 M NH4NO3 solution 3 times, and finally calcined in static air at 823 K for 3 h. H-ZSM-5 was synthesized by the same method but without adding Fe(NO3)3 in the starting synthesis system.
B Alkaline treatment
Approximately 2 g of the synthesized Fe-ZSM-5 zeolite was treated with 150 ml of 0.3 M NaOH aqueous solution for 2 h at 353 K. The resulting crystals were washed with demineralized water and dried at 383 K. Prior to the characterization, the alkali-treated zeolite was ion-exchanged and calcined, following the same procedure as that for Fe-ZSM-5. The alkali-treated Fe-ZSM-5 sample is referred to as Fe-ZSM-5-AT.
C Characterization
The X-ray powder diffraction (XRD) patterns were obtained on a Philips PW3040/60 diffractometer, using Cu-Kα radiation (λ = 0.1541 nm) in a scanning range of 5–50° at a scanning rate of 2° min−1. The scanning electron microscope (SEM) observations were performed on a Hitachi S-4800 apparatus equipped with a field emission gun. The acceleration voltage was set to 5 kV. The sample was stuck on the observation platform and sprayed with gold vapor under high vacuum for about 20 s. The transmission electron microscopy (TEM) observations were carried out on a JEOL JEM-1200 working at 300 kV. The sample was diluted in ethanol to give a 1
:
5 volume ratio and sonicated for 10 min. The ethanol slurry was then dropped onto a Cu grid covered with a thin film of carbon. The chemical composition of the samples was determined by a Jarrell-Ash 1100 inductively coupled plasma-atomic emission spectrometer (ICP-AES). The textural properties of the samples were determined by N2 adsorption at 77 K using a Micromeritics ASAP 2020 instrument. The sample was outgassed in vacuum at 623 K for 10 h before the adsorption measurement. The specific surface areas (SBET) were calculated using the BET equation and the micropore volumes (Vmicro) were determined using the t-plot method. The mesopore size distribution plots were obtained by the BJH model applied to the adsorption branches of the isotherms.31 The UV-vis diffuse reflectance spectra were recorded on a Nicolet Evolution 500 UV-vis spectrometer against BaSO4 under ambient conditions to investigate the nature of the Fe species in the catalysts. The uptakes of benzene in the zeolites Fe-ZSM-5 and Fe-ZSM-5-AT were carried out using a NETZSCH STA 449C thermogravimetric analyzer (TGA). The amount of the zeolite crystals loaded in the TGA sample cell was ca. 30 mg. Prior to the uptake experiments, the zeolite crystals were outgassed in the following way: after a temperature rise with a rate of 10 K min−1 in a He flow of 30 ml min−1, the sample was heated at 623 K for 5 h. The feed gas of benzene vapor in He was generated as follows: the carrier gas He was saturated by benzene vapor when passed through a bottle filled with liquid benzene at 298 K and was then diluted by He. The uptake of benzene with a partial pressure of 0.58 kPa in flowing He (a total flow rate of 30 ml min−1 and a total pressure of 101 kPa) in the zeolite crystals was subsequently measured at 363 K.
D
BTOP reaction
The direct oxidation of benzene to phenol was performed in a fixed-bed quartz tube reactor at 593 K and 101 kPa. A feed stream, in which a molar ratio of C6H6
:
N2O
:
He was 1
:
1
:
28, was prepared as follows: the carrier gas He was saturated by benzene vapor when passed through a bottle filled with liquid benzene at 298 K and was then mixed with a N2O flow in He. All the gas flows were controlled by mass flow controllers. A total gas flow of 60 ml min−1 was fed into the reactor where 0.2 g of the catalyst powder was loaded [a total weight hourly space velocity (WHSV) of 18
000 ml gcatal−1 h−1]. Before the reaction, the catalyst was pre-treated in flowing He at 773 K for 1 h to remove any impurities adsorbed on the catalyst. Afterwards the temperature in the reactor was cooled down to 593 K for the reaction. The concentrations of the gaseous stream from the outlet of the reactor were determined by an on-line GC (Varian 3800) equipped with an FID detector and a 30 m HP-5 capillary column.
III. Results and discussion
A Physicochemical properties of Fe-ZSM-5 and Fe-ZSM-5-AT
The XRD patterns (Fig. 1) confirm that the synthesized zeolites and their alkali-treated samples have an MFI-type structure, and no diffraction peak ascribed to α-Fe2O3 (reflections at 2θ = 33.2° and 35.7°)32 or any other iron oxides are observed in the samples. In comparison, the diffraction pattern of Fe-ZSM-5-AT is very similar to that of Fe-ZSM-5 but the intensity of the diffraction peaks of Fe-ZSM-5-AT is slightly decreased, probably due to some loss in its crystallinity.33 In addition, the SEM (Fig. 2) and TEM (Fig. 3) images indicate that although the crystal size is not changed (ca. 2 μm) after the alkaline treatment, a morphology change takes place, i.e., the crystal surface of Fe-ZSM-5-AT becomes rough. The ICP-AES analysis shows that the Si/Al and Si/Fe ratios in Fe-ZSM-5-AT are lower than those in the parent zeolite (Table 1). This is directly related to the preferential framework silicon extraction for the formation of mesopores in the zeolite crystals during the alkaline treatment. Furthermore, the absence of measurable amounts of Al and Fe in the filtrate indicates that Al and Fe species were not leached from the parent zeolite.
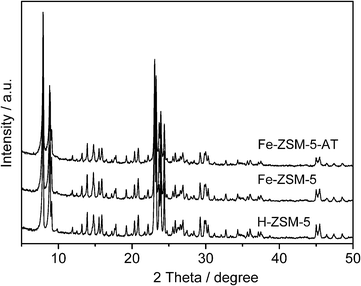 |
| Fig. 1
XRD patterns of H-ZSM-5, Fe-ZSM-5, and Fe-ZSM-5-AT. | |
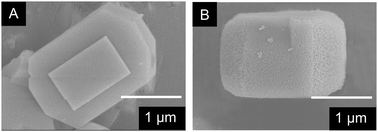 |
| Fig. 2
SEM images of Fe-ZSM-5 (A) and Fe-ZSM-5-AT (B). | |
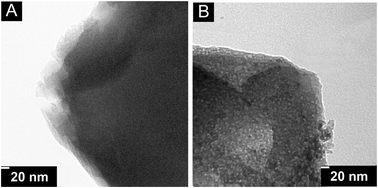 |
| Fig. 3
TEM images of Fe-ZSM-5 (A) and Fe-ZSM-5-AT (B). | |
Table 1 Chemical composition and textural properties of the parent Fe-ZSM-5 and alkaline-treated Fe-ZSM-5 zeolites
Sample |
Si/Ala (mol mol−1) |
Si/Fea (mol mol−1) |
S
BET
b (m2 g−1) |
V
total (cm3 g−1) |
V
micro
c (cm3 g−1) |
V
meso (cm3 g−1) |
ICP-AES analysis.
BET method.
t-plot method.
|
Fe-ZSM-5
|
24.6 |
301 |
378 |
0.21 |
0.15 |
0.06 |
Fe-ZSM-5-AT
|
14.2 |
260 |
398 |
0.33 |
0.13 |
0.20 |
The textural properties of the parent and alkali-treated zeolites are also summarized in Table 1. The parent zeolite Fe-ZSM-5 has a low mesopore volume of 0.06 cm3 g−1, and the corresponding adsorption isotherm of N2 on Fe-ZSM-5 also reveals a hysteresis loop, although it is not so obvious, compared with that on Fe-ZSM-5-AT (Fig. 4). This is due to the fact that Fe-ZSM-5 is prepared by a hydrothermal synthesis, the burning of the template in the sample during calcination induces the removal of Fe3+ from the framework to the extra-framework,34,35 leading to some defects in the zeolite framework, which in turn results in the formation of some mesoporosity.18 Differently, upon the alkaline treatment, the development of a distinct mesoporosity in Fe-ZSM-5-AT is demonstrated by a significant increase in mesopore volume to 0.20 cm3 g−1 (Table 1). The resulting BJH pore size distribution curve derived from the adsorption branch of the isotherm confirms the presence of mesopores centered around 15 nm for Fe-ZSM-5-AT (Fig. 5). Additionally, the micropore volume of Fe-ZSM-5-AT is 0.13 cm3 g−1, only slightly lower than 0.15 cm3 g−1 of the parent zeolite, indicating that after the alkaline treatment, the micropore system of the zeolite itself remains nearly unchanged.
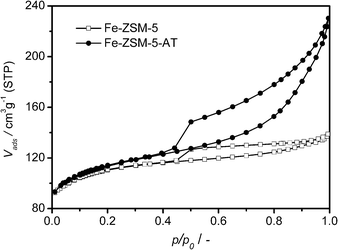 |
| Fig. 4 Isotherms of N2 on Fe-ZSM-5 and Fe-ZSM-5-AT at 77 K. | |
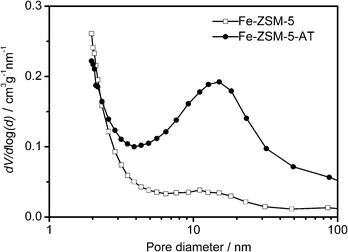 |
| Fig. 5 BJH pore size plots of Fe-ZSM-5 and Fe-ZSM-5-AT. | |
The UV-vis spectra of both samples shown in Fig. 6 predominantly feature two bands. According to the results from the literature,30,35–37 the band at 215 nm is ascribed to the framework Fe3+ species while the band at around 260 nm is associated with the extra-framework Fe3+ ones. The intensities of the band at 215 nm for both Fe-ZSM-5 and Fe-ZSM-5-AT are very similar while the intensity of the band at 260 nm for Fe-ZSM-5-AT is slightly lower, compared to that for Fe-ZSM-5. In addition, the bands between 300 and 400 nm for small oligonuclear Fex3+Oy clusters in the zeolite channels and the bands above 400 nm ascribed to Fe2O3 nanoparticles at the external surface of the zeolite crystal30,35–37 are more pronounced for Fe-ZSM-5-AT. Therefore, in combination with ICP-AES analysis indicating that Fe species are not leached from the parent zeolite during the alkaline treatment, it could be concluded that the number of extra-framework Fe3+ species (the active sites in the BTOP reaction) might be slightly decreased owing to the extra formation of some small oligonuclear Fex3+Oy clusters in the zeolite channels and Fe2O3 nanoparticles at the external surface of the zeolite crystal after the alkaline treatment, ion exchange, and calcination.
B
BTOP reaction
Fig. 7 shows a comparison result for the oxidation of benzene with N2O over Fe-ZSM-5 and Fe-ZSM-5-AT at 593 K. The two catalysts display different profiles. Over Fe-ZSM-5 the initially measured conversion of benzene is 15.4%, and the conversion has declined to 12.0% at a reaction time of 3 h. On the other hand, over Fe-ZSM-5-AT not only the initially measured conversion of benzene is 22.1%, much higher than that over Fe-ZSM-5, but also the catalyst shows a much better stability, although at a reaction time of 3 h the conversion is slightly decreased. Additionally, for comparison, the BTOP reaction over H-ZSM-5 was also investigated, and the result is included in Fig. 7. The initially measured conversion of benzene over H-ZSM-5 is only 1.5%, tenfold lower than the levels observed over the Fe-containing ZSM-5 catalysts. Thus, the Fe species in the zeolite are indispensable for the BTOP reaction, in accordance with the observation from the literature.7,38 Moreover, the H-ZSM-5 catalyst gets deactivated much faster.
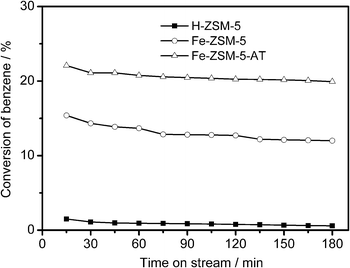 |
| Fig. 7 Conversion of benzene as a function of reaction time over H-ZSM-5, Fe-ZSM-5, and Fe-ZSM-5-AT catalysts. Reaction conditions: 593 K, 101 kPa, C6H6 : N2O : He (molar ratio) = 1 : 1 : 28, a total weight hourly space velocity (WHSV) of 18 000 ml gcatal−1 h−1. | |
It is worthy of note that the selectivity for phenol over both Fe-ZSM-5 and Fe-ZSM-5-AT catalysts is nearly 100% and is not changed with time on stream, although catalyst deactivation takes place. This implies that there could be no change in the mechanism of the catalyst deactivation, merely due to coke deposition on the zeolite channels and, thus, to decrease the number of the active sites.7
C Internal mass transfer
The uptakes of the reactant benzene in both Fe-ZSM-5 and Fe-ZSM-5-AT were measured to illustrate the effects of the formed mesopores on the improvement of the internal mass transfer properties. The normalized mass change as a function of time (t) is shown in Fig. 8. The uptake of benzene in Fe-ZSM-5-AT is much faster than that in Fe-ZSM-5. From a fundamental point of view, the intrinsic diffusivity of benzene in the micropores should be the same in the two zeolites. Therefore, the enhanced uptake rate of benzene in Fe-ZSM-5-AT crystals must be attributed to a shorter diffusion length in the micropores resulting from an accessible network of mesopores formed by the selective framework silicon extraction. Therefore, the presence of mesopores in Fe-ZSM-5-AT can significantly enhance its catalytic activity. In addition, a shorter diffusion length for the reactant benzene and the product phenol reflects a shorter residence time for these compounds in the micropores preventing the formation of coking, leading to a better catalyst stability over Fe-ZSM-5-AT.
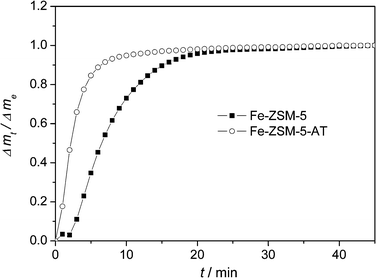 |
| Fig. 8 Normalized uptake profiles of benzene in Fe-ZSM-5 and Fe-ZSM-5-AT crystals at 363 K and a benzene partial pressure of 0.58 kPa in He. The lines are to guide the eye. | |
Gopalakrishnan et al.22 initially investigated the BTOP reaction over the mesoporous H-ZSM-5 zeolite obtained via desilication through the alkaline treatment of a commercial ZSM-5 zeolite containing a trace amount of Fe species, and they observed that the activity, stability, and selectivity for phenol over the alkali-treated H-ZSM-5 catalyst were significantly improved, compared with those over the parent H-ZSM-5 catalyst. They speculated that the improvement in the catalytic performances over the desilicated H-ZSM-5 was due to the presence of mesopores in the zeolite crystals.
However, compared with the results presented in the current paper, the following aspects regarding the work by Gopalakrishnan et al.22 should be addressed. Firstly, because of the only trace amount of Fe species present in the commercial H-ZSM-5 zeolite and its desilicated sample, both catalysts have very low activities in the BTOP reaction. Therefore, Gopalakrishnan et al. investigated the BTOP reaction in a temperature range from 673 to 753 K, much higher than the 593 K used in the current study, leading to a faster catalyst deactivation. The results for the BTOP reaction over the H-ZSM-5 in the current study confirm this aspect. Secondly, because of the trace amount of Fe species present in their catalysts, it could lead to some uncertainty in the determination of the number and state of the active Fe species before and after the alkaline treatment due to some limitation of the used techniques for analysis. Thirdly, Gopalakrishnan et al.22 reported that the selectivity for phenol over the commercial H-ZSM-5 zeolite decreased with time on stream while it remained almost constant over the desilicated catalyst. This difference in the selectivity for phenol between the parent and desilicated H-ZSM-5 zeolites could be due to the difference in the catalyst deactivation mechanism between the two catalysts. Differently, in the current study the selectivity for phenol over both Fe-ZSM-5 and Fe-ZSM-5-AT catalysts is nearly 100% and not changed with time on stream, although the degrees of the catalyst deactivation between the two catalysts are significantly different.
In the current study, the mesoporous Fe-ZSM-5 catalyst exhibits a superior catalytic performance in the BTOP reaction, i.e., a higher activity and a better stability with an absolute selectivity for phenol, compared to the mesoporous H-ZSM-5 reported by Gopalakrishnan et al.22 The Fe-ZSM-5 catalystsvia classical acid and steam post-treatments were also investigated, and their catalytic activities for the BTOP reaction were significantly enhanced, compared with that over Fe-ZSM-5 (these results are not included in Fig. 7). However, the acid or steam treated catalyst shows a much lower activity and gets deactivated faster, compared to Fe-ZSM-5-AT. During the steaming process (or acid treatment), Fe–O–Si and Al–O–Si bonds are broken and Fe and Al migrate toward extra-framework positions.38 These extra-framework Fe3+ species are responsible for enhancing the catalytic activity but the extra-framework Al3+ species could result in a faster catalyst deactivation due to coke formation. Through conventional steam and acid treatments, the introduced mesopores only moderately impact the intracrystalline diffusion due to the isolated nature of the created porosity (i.e., the mesopores do not constitute an interconnected accessible network).15 The results from the uptakes of benzene in both Fe-ZSM-5 and Fe-ZSM-5-AT demonstrate that the presence of the created mesopores in Fe-ZSM-5-AT can significantly improve the mass-transfer limitations due to a shorter diffusion length through the micropores. The shorter diffusion length through the micropores in the mesoporous zeolite also implies that the catalyst deactivation due to coking in the BTOP reaction can be improved to a great extent. The advantageous approach to manufacture the hierarchically structured micro- and mesoporous Fe-ZSM-5 zeolite for improved mass-transfer properties, as compared to the classical dealumination post-treatments, enables a more efficient utilization of the zeolite in the BTOP reaction that is strongly affected by diffusional limitations.
IV. Conclusions
The hierarchically structured micro- and mesoporous Fe-ZSM-5 zeolite can be obtained via desilication in the alkaline medium of Fe-ZSM-5 crystals. Fe species are not leached from the parent zeolite during the alkaline treatment but the number of the extra-framework Fe3+ species might be slightly decreased owing to the extra formation of some iron oxides in the zeolite channels as well as at the external surface of the zeolite crystal after the alkaline treatment, ion exchange, and calcination. The uptakes of benzene in both parent and desilicated zeolites demonstrate that the characteristic diffusion length in the mesoporous Fe-ZSM-5 crystals is dramatically shortened, resulting in an enhanced activity and stability for the catalytic oxidation of benzene with N2O as oxidant, which is strongly affected by diffusional limitations.
Acknowledgements
The financial support by the National Natural Science Foundation of China (20871104, 20971109, and 21036006), the Program for Changjiang Scholars and Innovative Research Team in Chinese Universities (IRT0980), and the Natural Science Foundation of Zhejiang Province, China (R4080084) is gratefully acknowledged.
Notes and references
- R. J. Schmidt, Appl. Catal., A, 2005, 280, 89–103 CrossRef CAS.
- P. P. Notté, Top. Catal., 2000, 13, 387–394 CrossRef.
- V. N. Parmon, G. I. Panov, A. Uriarte and A. S. Noskov, Catal. Today, 2005, 100, 115–131 CrossRef CAS.
- E. J. M. Hensen, Q. Zhu and R. A. van Santen, J. Catal., 2003, 220, 260–264 CrossRef CAS.
- E. J. M. Hensen, Q. Zhu, R. A. J. Janssen, P. C. C. M. Magusin, P. J. Kooyman and R. A. van Santen, J. Catal., 2005, 233, 123–135 CrossRef CAS.
- D. Meloni, R. Monaci, V. Solinas, G. Berlier, S. Bordiga, I. Rossetti, C. Oliva and L. Forni, J. Catal., 2003, 214, 169–178 CrossRef CAS.
- G. I. Panov, A. S. Kharitonov and V. I. Sobolev, Appl. Catal., A, 1993, 98, 1–20 CrossRef CAS.
- R. Buerch and C. Howitt, Appl. Catal., A, 1993, 106, 167–183 CrossRef.
- J. L. Motz, H. Heinichen and W. F. Holdrich, J. Mol. Catal. A: Chem., 1998, 136, 175–184 CrossRef CAS.
- D. P. Ivanov, M. A. Rodkin, K. A. Dubkov, A. S. Kharitonov and G. I. Panov, Kinet. Catal., 2000, 41, 771–775 CrossRef CAS.
- S. M. Campbell, D. M. Bibby, J. M. Coddington, R. F. Howe and R. H. Meinhold, J. Catal., 1996, 161, 338–349 CrossRef CAS.
- T. Masuda, Y. Fujikata, S. R. Mukai and K. Hashimoto, Appl. Catal., A, 1998, 172, 73–83 CrossRef CAS.
- A. K. Ghosh and R. A. Kydd, Zeolites, 1990, 10, 766–771 CrossRef CAS.
- P. J. Kooyman, P. Van der Waal and H. Van Bekkum, Zeolites, 1997, 18, 50–53 CrossRef CAS.
- J. C. Groen, W. D. Zhu, S. Brouwer, S. J. Huynink, F. Kapteijn, J. A. Moulijn and J. Pérez-Ramírez, J. Am. Chem. Soc., 2007, 129, 355–360 CrossRef CAS.
- J. C. Groen, L. A. A. Peffer, J. A. Moulijn and J. Pérez-Ramírez, Chem.–Eur. J., 2005, 11, 4983–4994 CrossRef CAS.
- J. C. Groen, J. A. Moulijn and J. Pérez-Ramírez, J. Mater. Chem., 2006, 16, 2121–2131 RSC.
- J. C. Groen, L. Maldonado, E. Berrier, A. Bruckner, J. A. Moulijn and J. Pérez-Ramírez, J. Phys. Chem. B, 2006, 110, 20369–20378 CrossRef CAS.
- I. Melián-Cabrera, S. Espinosa, C. Mentruit, F. Kapteijn and J. A. Moulijn, Catal. Commun., 2006, 7, 100–103 CrossRef.
- S. Abelló, A. Bonilla and J. Pérez-Ramírez, Appl. Catal., A, 2009, 364, 191–198 CrossRef.
- J. C. Groen, J. C. Jansen, J. A. Moulijn and J. Pérez-Ramírez, J. Phys. Chem. B, 2004, 108, 13062–13065 CrossRef CAS.
- S. Gopalakrishnan, A. Zampieri and W. Schwieger, J. Catal., 2008, 260, 193–197 CrossRef CAS.
- A. Reitzmann, E. Klemm and G. Emig, Chem. Eng. J., 2002, 90, 149–164 CrossRef CAS.
- W. Zhu, F. Kapteijn, B. van der Linden and J. A. Moulijn, Phys. Chem. Chem. Phys., 2001, 3, 1755–1761 RSC.
- W. Zhu, F. Kapteijn and J. A. Moulijn, Microporous Mesoporous Mater., 2001, 47, 157–171 CrossRef CAS.
- K. A. Dubkov, N. S. Ovanesyan, A. A. Shteinmain, E. V. Starokon and G. I. Panov, J. Catal., 2002, 207, 341–352 CrossRef CAS.
- E. Hensen, Q. Zhu, P. H. Liu, K. J. Chao and R. A. van Santen, J. Catal., 2004, 226, 466–470 CrossRef CAS.
- J. B. Taboada, E. J. M. Hensen, I. W. C. E. Arends, G. Mul and A. R. Overweg, Catal. Today, 2005, 110, 221–227 CrossRef CAS.
- A. Zecchina, M. Rivallan, G. Berlier, C. Lamberti and G. Ricchiardi, Phys. Chem. Chem. Phys., 2007, 9, 3483–3499 RSC.
- J. Pérez-Ramírez, J. C. Groen, A. Brückner, M. S. Kumar, U. Bentrup, M. N. Debbagh and L. A. Villaescusa, J. Catal., 2005, 232, 318–334 CrossRef.
- E. P. Barrett, L. G. Joyner and P. P. Halenda, J. Am. Chem. Soc., 1951, 73, 373–380 CrossRef CAS.
- M. H. Sayyar and R. J. Wakeman, Chem. Eng. Res. Des., 2008, 86, 517–526 CrossRef CAS.
- J. C. Groen, L. A. A. Peffer, J. A. Moulijn and J. Pérez-Ramírez, Colloids Surf., A, 2004, 241, 53–58 CrossRef CAS.
- A. Brückner, R. Lück, W. Wieker, B. Fahlke and H. Mehner, Zeolites, 1992, 12, 380–385 CrossRef.
- S. Bordiga, R. Buzzoni, F. Geobaldo, C. Lamberti, E. Giamello, A. Zecchina, G. Leofanti, G. Petrini, G. Tozzola and G. Vlaic, J. Catal., 1996, 158, 486–501 CrossRef CAS.
- J. Pérez-Ramírez, M. S. Kumar and A. Brückner, J. Catal., 2004, 223, 13–27 CrossRef.
- H. C. Xin, A. Koekkoek, Q. H. Yang, R. van Santen, C. Li and E. J. M. Hensen, Chem. Commun., 2009, 7590–7592 RSC.
- A. Ribera, I. W. C. E. Arends, S. de Vries, J. Pérez-Ramírez and R. A. Sheldon, J. Catal., 2000, 195, 287–297 CrossRef CAS.
|
This journal is © The Royal Society of Chemistry 2011 |