Poly-lysine supported cross-linked enzyme aggregates with efficient enzymatic activity and high operational stability†
Received
10th March 2011
, Accepted 19th July 2011
First published on 10th August 2011
Abstract
In this study, the operational stability of enzymes in a cross-linked aggregate (CLEA) formed with poly-lysine was examined. Chymotrypsin, subtilisin, citrate synthase and laccase, which are structurally and mechanistically diverse, were used as model enzymes. The preparation of poly-lysine supported CLEA was completed within 3 h. The immobilized enzymes were more stable than free enzymes at high temperature, in the presence of a chemical denaturant or in an organic solvent and were recycled without appreciable loss of activity. In addition, the immobilized proteases showed higher or similar hydrolytic activity in acidic pH than in neutral pH. This immobilization method was also applicable to the multi-subunit protein. These results suggest that the poly-Lys supported CLEA can be used as catalysts with own enzymatic activity and high operational stability.
Introduction
Enzymes have high catalytic activity and substrate specificity. Consequently, they are often applied as catalysts in industrial synthesis.1–3 However, because they lack operational stability and reusability, an important limitation hinders the use of enzymes in wider applications. Enzyme immobilization presents one means to overcome these difficulties.4,5 Immobilized enzymes present several advantages. An important benefit is their often-enhanced stability against thermal or chemical denaturation and autolysis (when a protease is immobilized), which provides high operational stability and reusability.5 Furthermore, the immobilized enzyme can be isolated from the product easily, requiring no purification steps. Because of these interesting features, many applications using the immobilized enzymes have been reported recently, including organic synthesis,4,5proteolysis in proteomic analysis,6–8 biosensors and protein microarrays.9
Enzyme immobilization is usually conducted using a non-covalent binding method or a covalent binding method.9 The non-covalent binding method is often performed using an affinity interaction such as a histidine-tagged enzyme to a chelating-agarose gel.4,9 Although the binding are specific interactions, it can be used only for the recombinant tagged enzymes. In contrast, the covalent binding methods are achieved by chemical reactions between the side-chains in protein and a solid support such as modified glass or poly-methylmethacrylate. These methods require no modification of the target enzymes. Therefore, they are widely applicable. However, it is difficult to control the immobilization yield because multiple reaction points exist in these covalent binding methods. Furthermore, the enzyme's conformation is often altered, engendering reduction of its catalytic activity and operational stability.10,11 In addition, the reported procedures for producing immobilized enzymes include multi-step procedures necessitating considerable time and effort.7,12,13 Therefore, a facile preparation procedure of the immobilized enzymes is sought for routine use.
Cross-linked enzyme aggregation (CLEA) has been reported as a carrier-free immobilization method.5,14 Generally, CLEA is prepared by precipitating the enzyme with addition of a salt or an organic solvent with a subsequent cross-linking reaction by a cross-linker such as glutaraldehyde (GA). However, the cross-linking reaction might not be as effective as expected for enzymes with low Lys residue contents. Reportedly, the addition of albumin, which forms co-aggregates with the enzyme with a low Lys residue content, can form CLEA with activity.15,16 Nevertheless, this method was often insufficient to improve the stability.16 Consequently, this is not a universal method. We previously reported a method by which the enzyme was immobilized on the inner wall of the microchannel through a cross-linking polymerization between Lys residues on the mixture of acidic enzyme and poly-lysine (poly-Lys).17Acylase, which has an isoelectric point (pI) of 4.0, was immobilized using this method, and the obtained acylase-CLEA showed pH stability.17 Moreover, protease-CLEA prepared using this method showed rapid proteolysis and high stability against temperature, urea, and organic solvents.8,18 From these results, we infer that poly-Lys supported enzyme immobilization is applicable not only for acylase and proteases but also for other enzymes, and that the prepared CLEAs can be useful for catalysts with high operational stability. To examine this hypothesis, the poly-Lys supported CLEA formation was applied in this study for four enzymes, which are structurally and mechanistically diverse. Their enzymatic activity and operational stability were studied.
Experimental
Materials
Chymotrypsin (CT, from bovine pancreas), GA and paraformaldehyde (PA) were obtained from Wako Pure Chemical (Osaka, Japan). Citrate synthase (CS, from porcine heart) in an ammonium sulfate suspension, acetyl-CoA, 5,5′-dithiobis nitrobenzoic acid, N-glutaryl-Phe-p-nitroanilide (GPNA), laccase (Lac, from Trametes versicolor), oxaloacetic acid, poly-L-Lys hydrobromides (70
400 Da, 32
200 Da and 4200 Da), subtilisin Carlsberg (SBc, from Bacillus licheniformis) and N-succinyl-Ala-Ala-Pro-Phe-p-nitroanilide (Suc-AAPF-pNA) were purchased from Sigma-Aldrich (St. Louis, MO, USA). In addition, 2,2′-azino-di-(3-ethylbenzothiazoline-6-sulfonate) (ABTS) was purchased from KPL (Gaithersburg, MD, USA). All other chemicals were of analytical grade.
Formation of poly-Lys supported CLEAs and a turbidity measurement
Proteases (CT and SBc) and poly-Lys were dissolved in 50 mM phosphate buffer (PB, pH 8.0) at concentrations of 10 mg mL−1 and 20 mg mL−1, respectively. The cross-linker reagent containing GA (0.25%, v/v) and PA (4%, v/v) in 50 mM PB (pH 8.0) was used at a ratio of 1
:
16 (v/v).17 All solutions were filtered using a 0.45 μm polypropylene cellulose syringe filter (Minisart RC4; Sartorius Stedim Biotech, Goettingen, Germany) before use. The enzyme, poly-Lys and the cross-linker were mixed in a 96-well plate (Nunc, Kamstrup, Denmark). After different incubation times at 4 °C, the turbidity of the mixture was measured by the absorbance at 630 nm using a spectrophotometer (Multiskan JX; Thermo, Waltham, MA, USA).
Preparation of poly-Lys supported CLEAs for an enzymatic activity measurement
The CS solution was prepared using dialysis against 50 mM PB (pH 8.0) to remove ammonium sulfate before use. Other enzymes were dissolved in 50 mM PB (pH 8.0) without dialysis. Concentrations of proteins were determined using the Coomassie Plus Bradford assay (Pierce, Rockford, IL, USA). The stock concentrations of proteases (CT and SBc), CS and Lac were 10 mg mL−1, 634 μg mL−1 and 969 μg mL−1, respectively. The enzyme, poly-Lys and the cross-linker were mixed at a volume ratio (40 μL
:
40 μL
:
80 μL) in a test-tube and incubated at 4 °C for 2 h. Then CLEA was collected using centrifugation (12
000 rpm for 10 min). It was rinsed with 200 μL of 1 M Tris–HCl (pH 8.0) for 20 min at 4 °C, which simultaneously quenched any active aldehyde group. To reduce the resulting Schiff base, CLEA was treated with 200 μL of 50 mM NaCNBH3 in 50 mM borate buffer (pH 9.0) for 20 min at 4 °C. Then it was washed with 20 mM PB (pH 7.5). The immobilized enzyme in the CLEA matrix was analyzed using the BCA protein assay (Pierce) or absorbance at 280 nm using an uncross-linking enzyme fraction.
CT and SBc hydrolytic activity measurements
Typical measurements of hydrolytic activity using CT-CLEA and SBc-CLEA were conducted in 20 mM PB (pH 7.5) at 30 °C for 20 min (CT) or 10 min (SBc). The GPNA for CT (1 mM) and Suc-AAPF-pNA for SBc (200 μM) were used as substrates. The chemical stability of CLEA was tested in a reaction buffer with urea (1 to 7 M) at 30 °C. The thermal stability of CLEA was tested in 50 mM PB (pH 7.5) at 30 or 50 °C. For pH stability, CLEA was incubated in buffer of several pH values (5.5–9.0) at 30 °C, using acetate buffer for pH 5.5, PB for pH of 6.0–8.0 or borate buffer for pH 9.0. The kinetic parameters, Km and kcat, were measured at various substrate concentrations. The data were fitted to the Michaelis–Menten equation.18 The reaction was evaluated as the amount of released p-nitroaniline measured from absorbance at 405 nm. For in-solution experiments, the concentrations of free CT and free SBc were 50 and 2 μg mL−1, respectively.
Lac enzymatic activity measurement
The activity was typically measured at 30 °C for 10 min in 100 mM acetate buffer (pH 4.5), for which ABTS (50 μM) was used as a substrate. For pH stability, CLEA was incubated in buffer with several pH values (4.5–9.0). Other reaction conditions are described above. The absorbance at 405 nm was measured at room temperature using a spectrophotometer. For in-solution experiments, the free Lac concentration was 5 μg mL−1; hydrochloric acid (1 M) was added to the solution to stop the reaction.
CS enzymatic activity measurement
CS-CLEA was added to a reaction mixture containing 20 mM Tris–HCl (pH 7.5), 300 μM acetyl-CoA, 100 μM oxaloacetic acid and 100 μM 5,5′-dithiobis nitrobenzoic acid.19,20 The reaction was conducted with or without 3 M urea at 30 or 50 °C for 20 min. For in-solution experiments, the free CS concentration was 1 μg mL−1.
Results and discussion
Poly-Lys supported enzyme immobilization
A typical procedure for cross-linking an enzyme involves activation of the primary amine groups of the enzyme with a cross-linker to create aldehyde groups that can react readily with other primary amine groups of enzymes.4,7,21 Cross-linking yields depend on the number of the Lys residues of the enzyme. Therefore, the acidic or neutral enzyme (pI < 7.0) cannot be cross-linked efficiently merely by the use of a cross-linker. Although high concentration of a cross-linker can increase the cross-linking yield, it often causes a change of its conformation, engendering a reduction of its catalytic activity.10,11 To overcome this difficulty, poly-Lys was used as the amine donor in this study. It is expected that the large number of primary amine groups of poly-Lys can improve the cross-linking yield with lower concentration of cross-linker than those in reported procedures (typically 5–10% of GA, v/v).22,23 Based on a similar idea, pentaethylenehexamine and albumin were used to cross-link the chloroperoxidase enzyme (pI = 4.1).15
The preparation steps of CLEA are presented in Fig. 1. As a cross-linker, the mixture of GA and PA was used at a ratio of 1
:
16 (v/v).21 The resulting Schiff base was reduced by NaCNBH3. To test the usability of our method for several types of enzymes, we prepared CLEAs using CT, SBc, Lac and CS. Their pI values were 8.4, 6.6, 5.8 and 7.0, respectively. Although the pI value of CT is close to the pH value of the reaction buffer (8.0), the reaction without poly-Lys showed low cross-linking yield (data not shown). The other three enzymes were also not efficiently cross-linked without poly-Lys under our experimental conditions, suggesting that only Lys residues on the surface of the enzymes are insufficient for cross-linking.
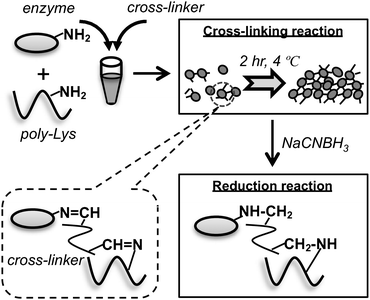 |
| Fig. 1 Preparation of the poly-Lys supported CLEA. The cross-linking reaction between the aldehyde groups of a cross-linker and amino groups present in the enzyme and/or poly-Lys forms reversible imine groups (Schiff base). To form stable amine groups, the CLEA matrix was reduced by NaCNBH3. | |
Effect of poly-Lys concentration on CLEA formation
To optimize the cross-linking reaction, we first studied the ratio of poly-Lys and enzyme. The CT and SBc that were frequently applied to study the enzymatic reactions for organic synthesis24,25 were used. The molecular weight of poly-Lys was 70 kDa. Different concentrations of poly-Lys (1.25, 2.5 and 5.0 mg mL−1) were mixed with CT or SBc at constant concentration (2.5 mg mL−1). The mixture was cross-linked by GA (0.25%, v/v) and PA (5%, v/v) in 50 mM PB (pH 8.0). To avoid autolysis of protease in bulk solution during the cross-linking reaction, the preparation of CLEA was conducted at 4 °C.18
The CLEA formation was evaluated according to the reaction mixture turbidity.17 The increase in poly-Lys concentration increased the turbidity (Fig. S1†), suggesting that enzymes were efficiently cross-linked in the presence of poly-Lys. One- or two-fold poly-Lys concentration was sufficient to form CLEA. After reaction for 2 h, more than 75% of CT and more than 65% of SBc had been cross-linked. No turbidity was observed when mixing the enzyme and poly-Lys without a cross-linker.
Both CLEAs showed their own hydrolytic activity (Fig. S1, ESI†), indicating that both proteases maintained their active conformations in the CLEA matrices. CT-CLEAs prepared using equivalent poly-Lys concentration with CT concentration showed higher activity than that by low poly-Lys concentration (Fig. S1A, ESI†). Leakage of free CT from the CLEA matrix was not observed during washing steps when high poly-Lys concentration was used. In contrast, SBc-CLEAs showed similar immobilization yield (≥65%) and activity (>90%) in all cases (Fig. S1B, ESI†). These results suggest that acidic SBc (pI = 6.6) can interact easily with basic poly-Lys, even at low poly-Lys concentration, while basic CT (pI = 8.4) requires high concentration of poly-Lys. This suggestion is supported by the results obtained for Lac, CS (see below) and alkaline phosphatase (pI = 5.9), which need no great amount of poly-Lys for immobilization.8
The molecular weight (MW) of poly-Lys can also affect the interaction between the enzyme and poly-Lys. Therefore, we studied the formation of CT-CLEA using different MWs of poly-Lys (32 kDa and 4 kDa). The CT and poly-Lys were mixed at a ratio of 1
:
1 and the cross-linking reaction was performed for 2 h at 4 °C. The turbidity measurement revealed that the advantage of lower MWs (32 kDa and 4 kDa) was lower CLEA formation than that in MW of 70 kDa (data not shown). Further experiments were performed using poly-Lys with 70 kDa.
Effect of cross-linker concentration on CLEA formation
We next optimized the cross-linker concentration for preparations of CT-CLEA and SBc-CLEA. Based on the above results, protease and poly-Lys were mixed at a ratio of 1
:
1, and the cross-linking reaction was performed for 2 h at 4 °C.
Using various amounts of the cross-linker mixture, we found that optimal concentrations of PA and GA for CT-CLEA were 2% (v/v) and 0.125% (v/v), respectively (Fig. S2A†). The activity recovery was 45% at 30 °C. Further increasing the cross-linker concentration produced similar immobilization yields (ca. 75%) and did not change the activity (ca. 40%). In contrast, CT-CLEA prepared with a low concentration of cross-linker (PA = 0.5%, v/v and GA = 0.031%, v/v) showed much lower immobilization yield and activity than those of other CT-CLEAs. For SBc-CLEA, the concentration of the cross-linker did not affect the immobilization yield (ca. 60%) or activity (≥90%) at 30 °C (Fig. S2B, ESI†). Moreover, no leakage of free SBc was observed using a low concentration of cross-linker. These results suggest that the acidic enzymes were sufficiently immobilized at low concentrations of cross-linker in the presence of poly-Lys.
Thermal stabilities of protease-CLEAs
We studied thermal stabilities of CT-CLEA and SBc-CLEA. The activities of both CLEAs at 50 °C are depicted in Fig. S2 (ESI†). At 50 °C, free CT showed lower activity than that at 30 °C (data not shown). In contrast, CT-CLEAs at 50 °C showed 1.6-fold higher activity than those at 30°C (Fig. S2A, ESI†), indicating that the immobilized CT has thermal stability and that diffusion of the substrate moving into the CLEA matrix was increased at 50 °C.
Reportedly, subtilisin from Bacillus subtilis showed high thermal stability.26 As reported, free SBc were not denatured at 50 °C and showed hydrolytic activity (data not shown). All five SBc-CLEAs kept at 30 °C retained their activity (>90% in Fig. S2B, ESI†), indicating that the immobilized SBc in the CLEA matrix also maintained thermal stability.
pH profiles of protease-CLEAs
Immobilized enzymes often produce a shift in the optimum pH.15,17,26 Therefore, the effects of pH on the activity of CT-CLEA and SBc-CLEA were examined at pH 5.5–9.0 at 30 °C (Fig. 2). Free CT and free SBc are well known to show maximum hydrolytic activity at around pH 7.0. In contrast, the pH profiles of both protease-CLEAs were broader than those of free proteases. An interesting difference is that both protease-CLEAs maintain their hydrolytic activity, even at pH 5.5. Because the enzymatic reaction at broad pH can be useful for catalysis in chemical and biotechnology fields, kinetic parameters by CT-CLEA and SBc-CLEA at pH 7.5 and pH 5.5 were measured (Tables S1 and S2†). The CT-CLEAs prepared using 2–3% (v/v) of PA and 0.125–0.187% (v/v) of GA showed higher kcat/Km than other CT-CLEAs exhibited at both pH conditions (Table S1, ESI†). At pH 7.5, kcat/Km values of CT-CLEAs were slightly less than those of free CT, probably because of the diffusional resistance of the substrate moving into the CLEA matrices. In contrast, at pH 5.5, kcat/Km values of CT-CLEAs were 5.6-fold higher than those of free CT. Moreover, Km values of CT-CLEAs were 7-fold lower than those of free CT at pH 5.5. A lower Km value is known to represent higher binding affinity between the enzyme and substrate, therefore suggesting that basic CLEA matrices with high poly-Lys contents probably interact easily with an acidic substrate (GPNA) at pH 5.5 than at pH 7.5. Because all SBc-CLEAs prepared using different cross-linker concentrations showed similar hydrolytic activity (Fig. S2B and 2B, ESI†), two SBc-CLEAs that were prepared using lowest and highest cross-linker concentrations were tested (Table S2, ESI†). At pH 7.5, kcat/Km values of both SBc-CLEAs were approximately 400-fold less than that of the free enzyme, probably because of the mass transfer limitation of the substrate in the SBc-CLEA matrix. In contrast, the kcat/Km value of free SBc at pH 5.5 was 5.8-fold lower than that at pH 7.5. Nevertheless, SBc-CLEAs maintained their kcat/Km values. These results suggest that both proteases in the CLEA matrices maintained their active conformations, leading to their own activities at pH 5.5.
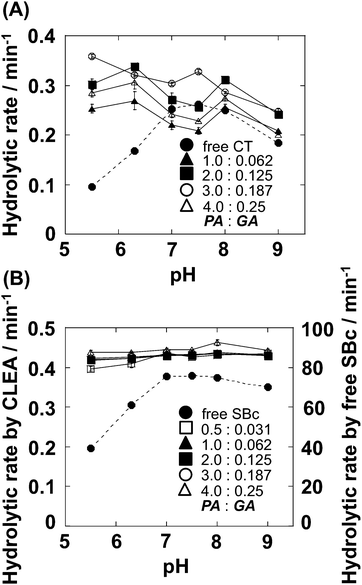 |
| Fig. 2 pH profile of CT-CLEA (A) and SBc-CLEA (B) prepared using different concentrations of PA (%, v/v) and GA (%, v/v). Hydrolytic reactions were conducted in 20 mM PB (pH 7.5) at 30 °C. Substrate: GPNA (1 mM) for CT-CLEA; Suc-AAPF-pNA (200 μM) for SBc-CLEA. Concentrations of free CT and free SBc were 50 and 2 μg mL−1, respectively. The graph shows the mean ± standard error for at least three experiments. | |
Hydrolysis activity of protease-CLEAs in urea or DMSO solution
We next studied the chemical stability of the immobilized enzyme against urea at 30 °C. Estimated [urea]1/2 of CT-CLEA (PA of 2%, v/v and GA of 0.125%, v/v), which is the necessary concentration of urea for half of the hydrolytic activity at 30 °C, was 6.6 M, whereas that of free CT was 3.4 M. It is indicated that the immobilization of CT increased the chemical stability. In contrast, free SBc was not denatured, even at 7 M urea. Therefore, we did not test the stability of SBc-CLEA.
The reaction in the organic solvent is important for the synthetic application of catalyst. Therefore, the hydrolytic reactions by protease-CLEAs (PA of 2%, v/v and GA of 0.125%, v/v) were performed in several organic solvents. Protease needs molecular water for its hydrolytic reaction. Therefore, DMSO, which is a miscible solvent with water, was used. The residual activities of CT-CLEA and SBc-CLEA in 40% (v/v) DMSO were 27% and 59%, respectively, although free proteases showed lower activity (<20%). The decrease in activity in DMSO might result from the stripping of water from the enzyme surface.26 It is possible that the CLEA matrix can retain water even in organic solvent. Therefore, the immobilized proteases were possibly more stabilized than free proteases. Similar stabilities of protease-CLEAs were observed in ethanol, 2-propanol and THF (data not shown).
Stability of Lac-CLEA
Lac, an industrially useful polyphenol oxidase, has four copper atoms in its catalytic site. Recently, Lac members have been applied for organic synthesis27 or degradation of recalcitrant compounds.28,29
Based on the results described above, Lac and poly-Lys were mixed at a ratio of 1
:
1. Then the cross-linking reaction was performed for 2 h at 4 °C. The pH profiles of Lac-CLEAs that had been prepared using different cross-linker concentrations are presented in Fig. 3. As free Lac, Lac-CLEAs showed the maximum activity, with the optimum pH of around 4.0. However, the activity recovery of Lac-CLEAs was lower than that of free Lac. Moreover, the stabilities of Lac-CLEAs against urea and DMSO were not improved (data not shown). In earlier papers, when Lac was immobilized using GA, Schiff base in the cross-linked Lac was not reduced by reducing reagents.16,29 Although those reports provided no description or explanation of reduction steps, the copper atoms in the catalytic site were possibly affected. These data imply that our poly-Lys supported enzyme immobilization is inapplicable to the enzyme with metal atom(s) in its catalytic site.
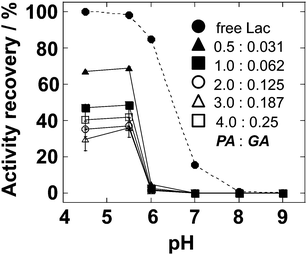 |
| Fig. 3 pH profile of Lac-CLEA that was prepared by different concentrations of PA (%, v/v) and GA (%, v/v). The results are presented as the activity recovery to free Lac at pH 4.5. All assays were conducted at 30 °C for 10 min. The concentration of free Lac was 5 μg mL−1. The graph shows the mean ± standard error for at least three experiments. | |
Stability of CS-CLEA
CS synthesizes citrate from oxaloacetic acid and acetyl-CoA. It is recognized to be of low operational stability.19,20 In addition, to express its activity, CS must form a dimer comprising two identical subunits. Therefore, it is expected that CS is a good model enzyme to test not only the operational stability, it is also useful as an application of our immobilization procedure for a multi-subunit enzyme.
For 2 h at 4 °C, CS, two-fold poly-Lys and the cross-linker (GA of 0.25%, v/v and PA of 4%, v/v) were mixed. Under these conditions, free CS was cross-linked almost completely in CS-CLEA. As depicted in Fig. 4, CS-CLEA showed its own activity, indicating that CS formed a dimer in the CLEA matrix. The CS-CLEA stability against thermal denaturation and urea denaturation was tested. Because the activity measurement using 5,5′-dithiobis nitrobenzoic acid19 is conducted for a neutral pH solution, the assay was carried out at pH 7.5. At 3 M of urea, CS-CLEA and free CS showed 66% and 9% activity, respectively. The estimated [urea]1/2 of free CS at 30 °C was 1.1 M. Therefore, CS-CLEA was more stable than free CS. In addition, CS-CLEA showed higher activity at 50 °C than free-CS did. It is suggested that, because of multipoint cross-linking between CS and poly-Lys, the immobilized CS increased stability although free enzyme was thermally or chemically denatured. These data show that the poly-Lys supported CLEA can be useful for immobilization of the multi-subunit protein.
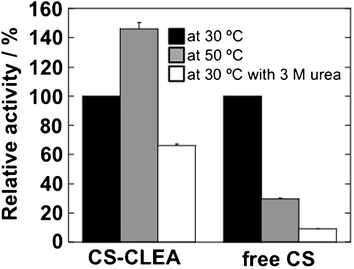 |
| Fig. 4 Enzymatic activity of CS-CLEA. The results are presented as the relative activity to that at 30 °C (black). The reactions were measured at 50 °C (grey) and at 30 °C with 3 M urea (white). All assays were performed in 20 mM Tris–HCl (pH 7.5). The concentration of free CS was 1 μg mL−1. The graph shows the mean ± standard error for at least three experiments. | |
Reusability
The reuse of the immobilized enzyme as a catalyst is important for a routine enzymatic reaction. Therefore, the reusability of CLEAs was tested. After each reaction cycle, CLEAs were washed with buffer solution and stored at 4 °C for more than 3 months. During this period, CLEAs were used for over 30 reaction cycles for the stability experiments described above. The respective residual activities of CT-, SBc- and CS-CLEAs, even after experiments of urea denaturation or thermal denaturation, were almost identical to those obtained before enzymatic reactions (>90%), indicating that these CLEAs maintained their activity after many uses. Additionally, no free enzymes were released from CT-, SBc- and CS-CLEAs, as measured using UV absorbance at 280 nm. Regarding free enzymes, they lost their activity almost completely within five days because free CS and Lac had formed precipitates and free proteases (CT and SBc) were digested by autolysis. Although the residual activity by Lac-CLEA was lower than that of free Lac (Fig 3), the storage stability during recycled use for 3 months was higher than that by free Lac. Once again, these results verified that the immobilized enzymes in each CLEA were stable, but this was not observed in the case of free enzymes. Although similar reusability of immobilized trypsin on polymer nanofibers30 or CLEAs that were prepared using the original procedure5,14 were previously reported, the reusability of poly-Lys supported CLEA was better than or comparable to those of the reported enzyme immobilization methods.
Conclusion
We studied the preparation of poly-Lys supported CLEAs and their operational stability. Poly-Lys was added to form co-aggregates with enzyme with a low Lys residue content. The present immobilization procedure was achieved within 3 h without the necessity for any special equipment. An acidic enzyme can interact easily with basic poly-Lys, even at low poly-Lys concentration, while a neutral or basic enzyme requires high concentration of poly-Lys (Fig. S1 and S4, ESI†). Moreover, this method is applicable to the multi-subunit protein (Fig. 4). In contrast, results of Lac-CLEA (Fig. 3) illustrate the difficulty of the application for the enzyme containing metal atom(s), indicating that the immobilization of the metalloenzyme must be modified in terms of its procedure.
The immobilized enzymes in present CLEAs were more stable against high temperature, pH, urea and DMSO than free enzymes (Fig. S2, ESI† and Fig. 2 and 4). It is indicated that the poly-Lys supported immobilization maintains their configurations for their own enzymatic activity from denaturation by heat or chemical reagents. In addition, CLEAs were recycled without appreciable loss of activity, which is important to ensure the cost-effective use of valuable enzymes. These advantages suggest that the poly-Lys supported CLEA are useful as catalysts in the fields of chemistry and biotechnology.
Acknowledgements
Part of this work was supported by Grant-in-Aid for Basic Scientific Research (B: 20310074 and 23310092) from the Japan Society for the Promotion of Science (JSPS).
Notes and references
- L. Hilterhaus and A. Liese, Adv. Biochem. Eng., 2007, 105, 133–173 CAS.
- R. A. Gross, M. Ganesh and W. Lu, Trends Biotechnol., 2010, 28, 435–443 CrossRef CAS.
- S. Sanchez and A. L. Demain, Org. Process Res. Dev., 2011, 15, 224–230 CrossRef CAS.
- M. Miyazaki and H. Maeda, Trends Biotechnol., 2006, 24, 463–470 CrossRef CAS.
- R. A. Sheldon, Adv. Synth. Catal., 2007, 349, 1289–1307 CrossRef CAS.
- H. Yamaguchi, M. Miyazaki and H. Maeda, Proteomics, 2010, 10, 2942–2949 CrossRef CAS.
- J. Ma, L. Zhang, Z. Liang, W. Zhang and Y. Zhang, Anal. Chim. Acta, 2009, 632, 1–8 CrossRef CAS.
- H. Yamaguchi, M. Miyazaki, H. Kawazumi and H. Maeda, Anal. Biochem., 2010, 407, 12–18 CrossRef CAS.
- L. S. Wang, F. Khan and J. Micklefield, Chem. Rev., 2009, 109, 4025–4053 CrossRef.
- H. H. P. Yiu and P. A. Wright, J. Mater. Chem., 2005, 15, 3690–3700 RSC.
- T. W. Cha, A. Guo and X. Y. Zhu, Proteomics, 2005, 5, 416–419 CrossRef CAS.
- M. Miyazaki, T. Honda, H. Yamaguchi, M. P. P. Briones and H. Maeda, Biotechnol. Genet. Eng. Rev., 2008, 25, 405–428 CrossRef CAS.
- J. Ma, L. Zhang, Z. Liang, W. Zhang and Y. Zhang, J. Sep. Sci., 2007, 30, 3050–3059 CrossRef CAS.
- R. A. Sheldon, Org. Process Res. Dev., 2011, 15, 213–223 CrossRef CAS.
- D. I. Perez, F. von Rantwijk and R. A. Sheldon, Adv. Synth. Catal., 2009, 351, 2133–2139 CrossRef CAS.
- I. Matijošyté, I. W. C. E. Arends, S. de Vries and R. A. Sheldon, J. Mol. Catal. B: Enzym., 2010, 62, 142–148 CrossRef.
- T. Honda, M. Miyazaki, H. Nakamura and H. Maeda, Adv. Synth. Catal., 2006, 348, 2163–2171 CrossRef CAS.
- H. Yamaguchi, M. Miyazaki, T. Honda, M. P. Briones-Nagata, K. Arima and H. Maeda, Electrophoresis, 2009, 30, 3257–3264 CrossRef CAS.
- D. L. Daugherty, D. Rozema, P. E. Hanson and S. H. Gellman, J. Biol. Chem., 1998, 273, 33961–33971 CrossRef CAS.
- H. Yamaguchi, M. Miyazaki, M. P. Briones-Nagata and H. Maeda, J. Biochem., 2010, 147, 895–903 CrossRef CAS.
- T. Honda, M. Miyazaki, H. Nakamura and H. Maeda, Chem. Commun., 2005, 5062–5064 RSC.
- J. Ma, Z. Ziang, X. Qiao, Q. Deng, D. Tao, L. Zhang and Y. Zhang, Anal. Chem., 2008, 80, 2949–2956 CrossRef CAS.
- H. Fan and G. Chen, Proteomics, 2007, 7, 3445–3449 CrossRef CAS.
- M. Kreiner and M. C. Parker, Biotechnol. Bioeng., 2004, 87, 24–33 CrossRef CAS.
- J. A. Rodríguez-Martínez, R. J. Solá, B. Castillo, H. R. Cintrón-Colón, I. Rivera-Rivera, G. Barletta and K. Griebenow, Biotechnol. Bioeng., 2008, 101, 1142–1149 CrossRef.
- K. Sangeetha and T. E. Abraham, Int. J. Biol. Macromol., 2008, 43, 314–319 CrossRef CAS.
- F. Bruyneel, O. Payen, A. Rescigno, B. Tinant and J. Marchand-Brynaert, Chem.–Eur. J., 2009, 15, 8283–8295 CrossRef CAS.
- A. I. Cañas and S. Camarero, Biotechnol. Adv., 2010, 28, 694–705 CrossRef.
- C. Nicolucci, S. Rossi, C. Menale, T. Godjevargova, Y. Ivanov, M. Bianco, L. Mita, U. Bencivenga, D. G. Mita and N. Diano, Biodegradation, 2011, 22, 673–683 CrossRef CAS.
- B. C. Kim, D. Lopez-Ferrer, S.-M. Lee, H.-K. Ahn, S. Nair, S. H. Kim, B. S. Kim, K. Petritis, D. G. Camp, J. W. Grate, R. D. Smith, Y.-M. Koo, M. B. Gu and J. Kim, Proteomics, 2009, 9, 1893–1900 CrossRef CAS.
Footnotes |
† Electronic supplementary information (ESI) available: Results of poly-Lys on CLEA formation and hydrolytic activity for CT-CLEA and SBc-CLEA. See DOI: 10.1039/c1cy00084e |
‡ Present address: Liberal Arts Education Center, Aso Campus, Tokai University, Aso, Minamiaso, Kumamoto 869-1404, Japan. |
|
This journal is © The Royal Society of Chemistry 2011 |