Microwave-assisted synthesis of ZnO–graphene composite for photocatalytic reduction of Cr(VI)
Received
28th March 2011
, Accepted 1st June 2011
First published on 11th July 2011
Abstract
ZnO–graphene composites are successfully synthesized via microwave-assisted reaction of zinc sulfate in aqueous solution with a graphite oxide dispersion using a microwave synthesis system. Their morphology, structure and photocatalytic performance in reduction of Cr(VI) are characterized by scanning electron microscopy, X-ray diffraction spectroscopy and UV-vis absorption spectrophotometer, respectively. The results show that in the composite the graphene nanosheets are decorated densely by ZnO nanosheets, which display a good combination between graphene and ZnO nanosheets. The ZnO–graphene composite exhibits an enhanced photocatalytic performance in the reduction of Cr(VI) with a removal rate of 98% under UV light irradiation as compared with pure ZnO (58%) due to the increased light absorption intensity and range, as well as the reduction of electron–hole pair recombination with the introduction of graphene.
1. Introduction
Currently considerable attention has been paid to semiconductor oxide photocatalysis for water treatment due to its ability to destroy pollutants and broad compound applicability.1–4 Among various semiconductor oxides, ZnO has been proven to be a promising photocatalyst for widespread environmental applications due to its intriguing optical and electric properties, low cost, and ease of availability.5–8 However, the quick recombination of photo-generated charge carriers has significantly decreased the photocatalytic performance of ZnO in aqueous solution. Currently a particularly attractive option is to design and develop hybrid materials based on ZnO to solve this problem.9–14Graphene is an emerging carbon material with a unique two-dimensional conjugated chemical structure, which causes its excellent electronic properties, superior chemical stability and high specific surface area.15–17 Attempts to combine ZnO and graphene have been reported in efforts to obtain hybrid materials with superior optical or electrical properties.18–24 Williams et al.25 and Akhavan26 fabricated a ZnO–graphene composite by ultra violet (UV)-assisted photocatalytic reduction of graphite oxide (GO) in ZnO suspensions. Wu et al.27 employed a facile solvothermal method to prepare a sandwich-like graphene–ZnO composite in an ethylene glycol medium using GO and zinc acetylacetonate as precursors. Li et al.28 reported the incorporation of graphene in ZnO to form a ZnO–graphene composite by chemically reducing the mixture of GO dispersion and Zn(AcO)2 in aqueous solution using NaBH4 and studied its significant influence on photocatalytic degradation of dyes under UV and visible light irradiation. Xu et al.29 prepared a graphene–ZnO composite by reducing GO coated on the surface of ZnO nanoparticles using hydrazine and found that the graphene–ZnO composite showed an improved photocatalytic performance in the degradation of organic dye as compared with pure ZnO. In the photocatalysis process, graphene can act as an excellent electron-acceptor/transport material to effectively facilitate the migration of photo-induced electrons and hinder the charge recombination in the electron-transfer processes due to the electronic interaction between ZnO and graphene, which enhances the photocatalytic performance of ZnO.28,29 Despite the above progress to date, as promising hybrid materials for photocatalysis, the exploration on ZnO–graphene composite is not nearly enough so far. Especially as an inexpensive, quick, versatile technique, microwave-assisted reaction is seldom employed to synthesize ZnO–graphene hybrid composite materials for photocatalysis although such a method has been used successfully to fabricate graphene30–33 and ZnO.34–37
In this work, one-step synthesis of a ZnO–graphene composite was carried out through microwave-assisted reaction of zinc sulfate in aqueous solution with a GO dispersion using a microwave system. Microwave irradiation can heat the reactant to a high temperature in a short time by transferring energy selectively to microwave absorbing polar solvents. Thus it can facilitate mass production in a short time with little energy cost32,38,39 and form an intimate contact between ZnO and graphene,40,41 which is crucial for the formation of an electronic interaction and inter-electron transfer at the interface.29 The ZnO–graphene composite exhibited an enhanced photocatalytic performance in the reduction of Cr(VI) under UV light irradiation as compared with pure ZnO and commercial P25 TiO2.
2. Experimental
2.1 Synthesis of ZnO–graphene composite
Commercial graphite powder was used as the starting reagent for the synthesis of GO via a modified Hummers method.42 A certain amount of 1.8 mg ml−1 GO suspension was added into 20 ml 0.1 M ZnSO4 solution, which was placed in a 35 ml microwave tube, and then the solution was sonicated for 30 min to produce a uniform dispersion. A dilute NaOH solution was dropped in the solution to form a brownish–black suspension with a pH value of 9. The mixture was then put into an automated focused microwave system (Explorer-48, CEM Co.) and treated for 10 min at 150 °C. It was obviously found that the color of suspension had changed to grayish–black, indicating the chemical reduction of the GO sheets.43,44 The obtained graphene consists of four or five carbon atom layers.41 The as-synthesized ZnO–graphene samples with 0.6, 0.8, 1.0, 1.2 wt% graphene, named as ZG-1, ZG-2, ZG-3 and ZG-4, were isolated by filtration, washed three times with distilled water, and finally dried in a vacuum oven at 60 °C for 24 h. Pure graphene was synthesized by direct microwave-assisted reduction of a GO suspension. Pure ZnO, obtained by direct microwave-assisted reaction of zinc ions in aqueous solution, and P25 TiO2 (Degussa) were also used for comparison.
Under acidic conditions of pH <6.0, the Zn exists as Zn2+ and ZnOH+ soluble ions in aqueous solution.41,45Zn2+ and ZnOH+ ions could be tightly adsorbed onto both sides of the GO due to the functional groups such as carboxyl, hydroxyl and epoxy groups on the surface of the GO. With the increase of pH value (∼9), a conversion from Zn2+ and ZnOH+ ions to Zn(OH)2 precipitation happens and finally GO is reduced to graphene and the Zn(OH)2 is transformed to ZnO sheets on the both sides of graphene under microwave heating.
2.2 Characterization
The surface morphology, structure and composition of the samples were characterized by field-emission scanning electron microscopy (FESEM, Hitachi S-4800) and X-ray diffraction spectroscopy (XRD, Holland Panalytical PRO PW3040/60) with Cu-Kα radiation (V = 30 kV, I = 25 mA), and energy dispersive X-ray spectroscopy (EDS, JEM-2100), respectively. The UV-vis absorption spectra were recorded using a Hitachi U-3900 UV-vis spectrophotometer.
2.3 Photocatalytic experiments
The photocatalytic performance of the as-prepared samples was evaluated by photocatalytic reduction of Cr(VI) under UV light irradiation. The samples (1 g l−1) were dispersed in 60 ml Cr(VI) suspensions (10 mg l−1) which were prepared by dissolving K2Cr2O7 into deionized water. The mixed suspensions were first magnetically stirred in the dark for 0.5 h to reach the adsorption–desorption equilibrium. Under ambient conditions and stirring, the mixed suspensions were exposed to UV irradiation produced by a 500 W high pressure Hg lamp with the main wave crest at 365 nm. At certain time intervals, 2 ml of the mixed suspensions were extracted and centrifuged to remove the photocatalyst. The filtrates were analyzed by recording UV-vis spectra of Cr(VI) using a Hitachi U-3900 UV-vis spectrophotometer.
3. Results and discussion
3.1 Characterization of materials
Fig. 1(a) and (b) show the FESEM images of as-synthesized graphene and ZnO. The graphene nanosheets are curled and corrugated and ZnO also displays the sheet nanostructure. Fig. 1(c) displays the FESEM image of ZG-3. The morphologies of ZG-1, ZG-2 and ZG-4 (not shown here) are similar to that of ZG-3. It is clearly observed that graphene nanosheets are decorated densely by ZnO nanosheets, which display a good combination between graphene and ZnO. The thickness of ZnO in the ZnO–graphene composite is about 20–30 nm, as shown in the inset of Fig. 1(c). The existence of ZnO in the composite has been proved by the peaks of Zn and O in EDS data (Fig. 1(d)).
The XRD patterns of graphene, ZnO, ZG-1, ZG-2, ZG-3, and ZG-4 are shown in Fig. 2. Graphene nanosheets exhibit a strong (002) diffraction peak at 26° and a (100) peak at 44.5°.46 The XRD analysis further shows that the main diffraction peaks of ZnO–graphene composite are similar to that of pure ZnO and corresponds to wurtzite-structured ZnO (JPCDS 36-1451), which indicates that the presence of graphene does not result in the development of new crystal orientations or changes in preferential orientations of ZnO. No typical diffraction peaks of carbon species are observed, which is due to the low amount of graphene in the composite.18
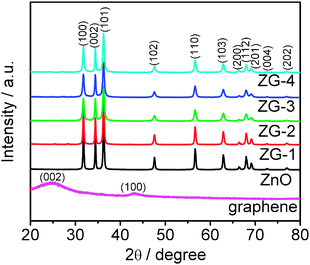 |
| Fig. 2
XRD patterns of graphene, ZnO, ZG-1, ZG-2, ZG-3, and ZG-4. | |
3.2 Photocatalytic activity
The photocatalytic reduction of Cr(VI) under UV irradiation was used to evaluate the photocatalytic performance of ZnO, P25, ZG-1, ZG-2, ZG-3, and ZG-4, as shown in Fig. 3. The normalized concentration changes (C/C0) of Cr(VI) during the photocatalytic process are proportional to the normalized maximum absorbance (A/A0), which can be derived from the changes in the Cr(VI) absorption profile at a given time interval. It is observed that Cr(VI) is hardly reduced under UV light in the absence of the photocatalyst and ZnO–graphene composites exhibit better photocatalytic performance than pure ZnO and P25. The removal rates of Cr(VI) for pure ZnO and P25 are 58% and 70%, respectively. When graphene is introduced into ZnO, the removal rate is increased to 65% and 85% for ZG-1 and ZG-2. After reaching a maximum value of 98% for ZG-3, the removal rate decreases with further increase of the graphene content. It indicates that the introduction of graphene into ZnO plays an important role in the photocatalytic performance of the ZnO–graphene composite.
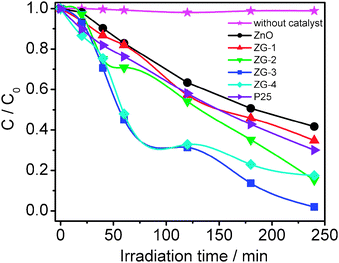 |
| Fig. 3 Photocatalytic reduction of Cr(VI) by ZnO, P25, ZG-1, ZG-2, ZG-3, and ZG-4 under UV irradiation. The concentrations of Cr(VI) and the photocatalyst are 10 mg l−1 and 1 g l−1, respectively. | |
In the photocatalytic reduction process of Cr(VI), ZnO is excited by the absorption of photons under UV irradiation and electron–hole pairs are created.
Cr(
VI) is reduced to Cr(
III) by the photogenerated electrons, while the hole reaction is the oxidation of
water to oxygen as follows
Cr2O72− + 14H+ + 6e− → 2Cr3+ + 7H2O |
3.3 Mechanism for photocatalytic activity
During the photocatalysis, the light absorption and the charge transportation and separation are crucial factors.47 The UV-vis absorption spectra of pure ZnO, ZG-1, ZG-2, ZG-3, and ZG-4 are shown in Fig. 4. The sharp characteristic absorption peak at 360 nm indicates the presence of good crystalline and impurity suppressed ZnO nanostructures.24 The blue shift in the absorption peak as compared to bulk ZnO (∼370 nm) is ascribed to the quantum size-effect of nanometre size.48,49 It is observed that the absorbance of ZnO–graphene composite increases with the increase of graphene content, which is similar to those reported in the hybridization of TiO2 with graphite-like carbon layers or graphene.50,51 The presence of graphene induces an increased light absorption intensity, which may be due to an increase of the surface electric charge of the oxides in the ZnO–graphene composite and modification of the fundamental process of electron–hole pair formation during irradiation.29 In addition, the band gap of the ZnO–graphene composite displays a red shift compared to pure ZnO nanosheets, which is ascribed to the chemical bonding between the semiconductor photocatalyst and graphene, which is similar to the result in the case of TiO2–graphene composite materials.52,53 Therefore, the presence of graphene in ZnO can increase the light absorption intensity and range, which results in the enhancement the photocatalytic performance.
Another mechanism responsible for the photocatalytic performance enhancement is the stepwise structure of energy levels constructed in the ZnO–graphene composite, as shown in Fig. 5. The conduction band of ZnO is −4.05 eV (vs. vacuum) and valence band −7.25 eV (vs. vacuum).28 The work function of graphene is −4.42 eV.54,55 On the basis of the relevant band positions of ZnO and graphene, photo-induced electrons easily transfer from the ZnO conduction band to graphene, which could efficiently separate the photo-induced electrons and hinder the charge recombination in electron-transfer processes, and thus also enhance the photocatalytic performance.29 However, when the graphene content is further increased above its optimum value, the photocatalytic performance deteriorates. This is ascribed to the following reasons: (i) graphene can absorb some UV light and thus there exists a light harvesting competition between ZnO and graphene with an increase of the amount of graphene;29,56 (ii) the excessive graphene can act as a kind of recombination center instead of providing an electron pathway.55,57 All of these lead to the decrease of the photocatalytic performance.
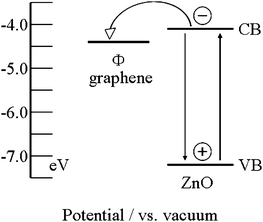 |
| Fig. 5 Schematic diagram of energy levels of ZnO and graphene. CB, VB, and Φ are the conduction band, valence band, and work function, respectively. | |
4. Conclusions
ZnO–graphene composites were successfully synthesized via microwave-assisted reaction of zinc sulfate in aqueous solution with a GO dispersion. The results of photocatalytic experiments indicate that (i) ZnO–graphene composites exhibit a better photocatalytic performance than pure ZnO and P25; (ii) the photocatalytic performance of ZnO–graphene is dependent on the proportion of graphene in the composite and a ZnO–graphene composite with 1.0 wt% graphene achieves a highest Cr(VI) removal rate of 98%; (iii) the enhanced photocatalytic performance is ascribed to the increased light absorption intensity and range, and the reduction of photoelectron–hole pair recombination with the introduction of graphene.
Acknowledgements
Financial support from Special Project for Nanotechnology of Shanghai (No. 1052nm02700) and the Key laboratory of new ceramics and fine processes at Tsinghua University and MOE (RG15/09) of Singapore is gratefully acknowledged.
Notes and references
- C. T. Chen, J. Q. Kao, C. Y. Liu and L. Y. Jiang, Catal. Sci. Technol., 2011, 1, 54–57 CAS.
- R. Guttel, M. Paul and F. Schuth, Catal. Sci. Technol., 2011, 1, 65–68 Search PubMed.
- C. C. Ou, C. S. Yang and S. H. Lin, Catal. Sci. Technol., 2011, 1, 295–307 CAS.
- J. Zhang, Y. P. Zhang, Y. K. Lei and C. X. Pan, Catal. Sci. Technol., 2011, 1, 273–278 CAS.
- R. Georgekutty, M. K. Seery and S. C. Pillai, J. Phys. Chem. C, 2008, 112, 13563–13570 CAS.
- D. Lin, H. Wu, R. Zhang and W. Pan, Chem. Mater., 2009, 21, 3479–3484 CrossRef CAS.
- S. F. Chen, W. Zhao, S. J. Zhang and W. Liu, Chem. Eng. J., 2009, 148, 263–269 CrossRef.
- Y. J. Tak and K. J. Yong, J. Phys. Chem. B, 2005, 109, 19263–19269 CrossRef CAS.
- S. Cho, J. W. Jang, J. S. Lee and K. H. Lee, CrystEngComm, 2010, 12, 3929–3935 RSC.
- J. T. Tian, L. J. Chen, Y. S. Yin, X. Wang, J. H. Dai, Z. B. Zhu, X. Y. Liu and P. W. Wu, Surf. Coat. Technol., 2009, 204, 205–214 CrossRef CAS.
- H. B. Fu, T. G. Xu, S. B. Zhu and Y. F. Zhu, Environ. Sci. Technol., 2008, 42, 8064–8069 CrossRef CAS.
- T. A. Saleh, M. A. Gondal, Q. A. Drmosh, Z. H. Yamani and A. Al-yamani, Chem. Eng. J., 2011, 166, 407–412 CrossRef CAS.
- P. S. S. Kumar, M. R. Raj, S. Anandan, M. F. Zhou and M. Ashokkumar, Water Sci. Technol., 2009, 60, 1589–1596 CrossRef CAS.
- L. W. Zhang, H. Y. Cheng, R. L. Zong and Y. F. Zhu, J. Phys. Chem. C, 2009, 113, 2368–2374 Search PubMed.
- T. N. Lambert, C. A. Chavez, B. Hernandez-Sanchez, P. Lu, N. S. Bell, A. Ambrosini, T. Friedman, T. J. Boyle, D. R. Wheeler and D. L. Huber, J. Phys. Chem. C, 2009, 113, 19812–19823 CAS.
- K. S. Novoselov, A. K. Geim, S. V. Morozov, D. Jiang, Y. Zhang, S. V. Dubonos, I. V. Grigorieva and A. A. Firsov, Science, 2004, 306, 666–669 CrossRef CAS.
- M. Pumera, Energy Environ. Sci., 2011, 4, 668–674 CAS.
- Y. L. Chen, Z. A. Hu, Y. Q. Chang, H. W. Wang, Z. Y. Zhang, Y. Y. Yang and H. Y. Wu, J. Phys. Chem. C, 2011, 115, 2563–2571 CAS.
- W. T. Zheng, Y. M. Ho, H. W. Tian, M. Wen, J. L. Qi and Y. A. Li, J. Phys. Chem. C, 2009, 113, 9164–9168 CAS.
- T. V. Cuong, V. H. Pham, J. S. Chung, E. W. Shin, D. H. Yoo, S. H. Hahn, J. S. Huh, G. H. Rue, E. J. Kim and S. H. Hur, Mater. Lett., 2010, 64, 2479–2482 CrossRef CAS.
- H. X. Chang, Z. H. Sun, K. Y. F. Ho, X. M. Tao, F. Yan, W. M. Kwok and Z. J. Zheng, Nanoscale, 2011, 3, 258–264 RSC.
- W. B. Zou, J. W. Zhu, Y. X. Sun and X. Wang, Mater. Chem. Phys., 2011, 125, 617–620 CrossRef CAS.
- J. O. Hwang, D. H. Lee, J. Y. Kim, T. H. Han, B. H. Kim, M. Park, K. No and S. O. Kim, J. Mater. Chem., 2011, 21, 3432–3437 RSC.
- J. Lin, M. Penchev, G. Wang, R. K. Paul, J. B. Zhong, X. Y. Jing, M. Ozkan and C. S. Ozkan, Small, 2010, 6, 2448–2452 CrossRef CAS.
- G. Williams and P. V. Kamat, Langmuir, 2009, 25, 13869–13873 CrossRef CAS.
- O. Akhavan, Carbon, 2011, 49, 11–18 CrossRef CAS.
- J. L. Wu, X. P. Shen, L. J. Jiang, K. Wang and K. M. Chen, Appl. Surf. Sci., 2010, 256, 2826–2830 CrossRef CAS.
- B. J. Li and H. Q. Cao, J. Mater. Chem., 2011, 21, 3346–3349 RSC.
- T. G. Xu, L. W. Zhang, H. Y. Cheng and Y. F. Zhu, Appl. Catal., B, 2011, 101, 382–387 CrossRef CAS.
- Z. Li, Y. G. Yao, Z. Y. Lin, K. S. Moon, W. Lin and C. P. Wong, J. Mater. Chem., 2010, 20, 4781–4783 RSC.
- W. Chen, L. Yan and P. R. Bangal, Carbon, 2010, 48, 1146–1152 CrossRef CAS.
- A. V. Murugan, T. Muraliganth and A. Manthiram, Chem. Mater., 2009, 21, 5004–5006 CrossRef CAS.
- B. G. Choi, H. Park, M. H. Yang, Y. M. Jung, S. Y. Lee, W. H. Hong and T. J. Park, Nanoscale, 2010, 2, 2692–2697 RSC.
- W. W. Wang and Y. J. Zhu, Inorg. Chem. Commun., 2004, 7, 1003–1005 CrossRef CAS.
- X. H. Zhang, S. Y. Xie, Z. Y. Jiang, X. Zhang, Z. Q. Tian, Z. X. Xie, R. B. Huang and L. S. Zheng, J. Phys. Chem. B, 2003, 107, 10114–10118 CrossRef CAS.
- X. L. Hu, Y. J. Zhu and S. W. Wang, Mater. Chem. Phys., 2004, 88, 421–426 CrossRef CAS.
- P. L. Zhu, J. W. Zhang, Z. S. Wu and Z. J. Zhang, Cryst. Growth Des., 2008, 8, 3148–3153 CAS.
- C. T. Lee, F. S. Chen and C. H. Lu, J. Alloys Compd., 2010, 490, 407–411 CrossRef CAS.
- F. Y. Jiang, C. M. Wang, Y. Fu and R. C. Liu, J. Alloys Compd., 2010, 503, L31–L33 CrossRef CAS.
- G. Zhu, L. K. Pan, T. Xu and Z. Sun, Nanoscale, 2011, 3, 2188–2193 RSC.
- T. Lu, L. K. Pan, H. B. Li, G. Zhu, T. Lv, X. J. Liu, Z. Sun, T. Chen and D. H. C. Chua, J. Alloys Compd., 2011, 509, 5488–5492 CrossRef CAS.
- H. B. Li, T. Lu, L. K. Pan, Y. P. Zhang and Z. Sun, J. Mater. Chem., 2009, 19, 6773–6779 RSC.
- H. A. Becerril, J. Mao, Z. Liu, R. M. Stoltenberg, Z. Bao and Y. Chen, ACS Nano, 2008, 2, 463–470 CrossRef CAS.
- N. A. Kotov, I. Dékány and J. H. Fendler, Adv. Mater., 1996, 8, 637–641 CrossRef CAS.
- X. Chen, Y. He, Q. Zhang, L. Li, D. Hu and T. Yin, J. Mater. Sci., 2009, 45, 953–960 CrossRef.
- L. H. Tang, Y. Wang, Y. M. Li, H. B. Feng, J. Lu and J. H. Li, Adv. Funct. Mater., 2009, 19, 2782–2789 CrossRef CAS.
- H. Zhang, X. J. Lv, Y. M. Li, Y. Wang and J. H. Li, ACS Nano, 2010, 4, 380–386 CrossRef CAS.
- J. W. Li, X. J. Liu, L. W. Yang, Z. F. Zhou, G. F. Xie, Y. Pan, X. H. Wang, J. Zhou, L. T. Li, L. K. Pan, Z. Sun and C. Q. Sun, Appl. Phys. Lett., 2009, 95, 031906 CrossRef.
- C. Q. Sun, Prog. Solid State Chem., 2007, 35, 1–159 CrossRef CAS.
- Y. J. Wang, R. Shi, J. Lin and Y. F. Zhu, Appl. Catal., B, 2010, 100, 179–183 CrossRef CAS.
- K. F. Zhou, Y. H. Zhu, X. L. Yang, X. Jiang and C. Z. Li, New J. Chem., 2011, 35, 353–359 RSC.
- H. Zhang, X. J. Lv, Y. M. Li, Y. Wang and J. H. Li, ACS Nano, 2010, 4, 380–386 CrossRef CAS.
- Y. H. Zhang, Z. R. Tang, X. Z. Fu and Y. J. Xu, ACS Nano, 2010, 4, 7303–7314 CrossRef CAS.
- R. Czerw, B. Foley, D. Tekleab, A. Rubio, P. M. Ajayan and D. L. Carroll, Phys. Rev. B: Condens. Matter, 2002, 66, 033408 CrossRef.
- N. L. Yang, J. Zhai, D. Wang, Y. S. Chen and L. Jiang, ACS Nano, 2010, 4, 887–894 CrossRef CAS.
- Y. B. Tang, C. S. Lee, J. Xu, Z. T. Liu, Z. H. Chen, Z. B. He, Y. L. Cao, G. D. Yuan, H. S. Song, L. M. Chen, L. B. Luo, H. M. Cheng, W. J. Zhang, I. Bello and S. T. Lee, ACS Nano, 2010, 4, 3482–3488 CrossRef CAS.
- G. Zhu, T. Xu, T. A. Lv, L. K. Pan, Q. F. Zhao and Z. Sun, J. Electroanal. Chem., 2011, 650, 248–251 CrossRef CAS.
|
This journal is © The Royal Society of Chemistry 2011 |