Structure and catalytic properties of hexagonal molybdenum disulfide nanoplates†
Received
19th February 2011
, Accepted 13th April 2011
First published on 12th May 2011
Abstract
Molybdenum disulfide (MoS2) is a compound very useful for its properties; it is used as a lubricant, catalyst in hydrodesulfurization, in hydrogen fuel storage, etc. In this work MoS2 hexagonal nanoplates were synthesized at different temperatures and characterized using X-ray diffraction (XRD), scanning electron microscopy (SEM), Raman spectroscopy and high resolution transmission electron microscopy (HRTEM). These nanoplates have a size of 25–35 nm as revealed by SEM. With aberration corrected STEM it was possible to measure the interatomic distance of Mo–Mo, which was found to be 2.8 Å. The catalytic properties of the nanoplates were measured in the hydrodesulfurization of dibenzothiophene, showing high activity and high direct desulfurization pathway (DDS) selectivity for an unpromoted MoS2 catalyst. Theoretical calculations were performed in 2H-MoS2 as well as 2H-MoS2 with a rotation of 16° and 19° which was applied to the two S planes in the crystalline structure. The results obtained on the rotated 2H-MoS2 yielded indication of a small gap semiconductor of Eg = 0.38 eV when compared to the unrotated 2H-MoS2, which is a semiconductor of Eg = 2.00 eV. This tendency of the rotated crystalline 2H-MoS2 toward metallicity could be responsible for the enhancement of the catalytic properties observed in the material in question, compared to other MoS2-based catalysts.
1. Introduction
Molybdenum disulfide is a widely used compound with many applications in catalysis1 and as solid lubricants,2 mainly because of the weak interlayer interaction of the material.3 More recent applications are hydrogen storage, as host–guest compounds, and as scanning tunneling microscope (STM) tips.4–8MoS2 is a transition metal dichalcogenide with properties that make it a material with promising applications such as solid-state secondary lithium battery cathodes,9 industrial catalysts for hydrodesulfurization of crude oil10 and as semiconductors.11 Similar to most of the transition metal chalcogenides, MoS2 displays the characteristic layered structure,12 metal chalcogenide slabs are formed by two layers of close-packed chalcogenide atoms sandwiching one metal layer between them. These slabs are stacked, with only van der Waals forces between the slabs only. As Mo adopts a trigonal-prismatic coordination, compounds such as MoS2 have semiconducting properties;13,14 the steric shielding of the metal atoms by the chalcogenide layers combined with the semiconducting properties makes MoS2 so inert that it resists oxidation in moist air even at 100 °C.15 This makes it an interesting material in the fields of catalysis, electrocatalysis, electrochemical intercalation and solid lubrication. It is interesting to note that small MoS2 clusters and nanoparticles exhibit triangular morphology, contrary to bulk MoS2.16,17 In this case, the catalytically important edges are to be found, if seen with scanning tunneling microscopy, reconstructed relative to the lattice layered compound of MoS2. Since the S–Mo–S sandwiches are held together by van der Waals interaction, this may play an important role in catalysis.18 In catalysis, MoS2 is also combined with other metals, such as Co, Ni and Fe,19 for use in hydrodesulfurization (HDS). It is generally agreed that the active sites reside at the edges of MoS2.17 In the past, researchers have synthesized MoS2 with different configurations, such as fullerenes,20,21nanorods,22nanotubes,23,24nanowires,25 nanoflowers,24,26 nanoflakes,27,28 nanospheres29,30 and nanohexagonal plates.3 Nanohexagonal plates are interesting because of its hexagonal shape and nanometric scale. This makes it a promising material for catalysis due to its high surface area. MoS2-based catalysts are widely used for the HDS of oil, so it is of interest to probe the nanohexagonal plates of MoS2 as potential catalysts for industrial HDS processes. The present report offers a detailed and systematic investigation of the synthesis of MoS2 nanoplates and its catalytic activity. The as synthesized plates were characterized by a variety of advanced electron microscopy techniques. High angle annular dark field scanning transmission electron microscopy (HAADF-STEM) imaging carried out with an aberration corrected (CS-STEM) revealed the atomic structure of the MoS2 plates in detail. The catalytic properties of these nanoparticles were tested, resulting in average to above average HDS activity compared to other MoS2-based catalysts, while differing significantly in its selectivity.
2. Experimental section
2.1 Synthesis
0.207 g of molybdenum(VI) oxide (MoO3), synthesized as described by Camacho-Bragado and José-Yacamán,31 and 0.414 g of sulfur (Sigma-Aldrich, 99.98%) were ground together in an agate mortar for 30 min. To this mixture more sulfur was added in order to have an excess of sulfur, finally having a mass ratio Mo
:
S of 1
:
5, the mixture was placed in a crucible and introduced into the hot zone of a tube furnace for 2 hours under an atmosphere of nitrogen. Then, the tube furnace was cooled down to room temperature. This mixture was annealed for 1 hour under an atmosphere of nitrogen and quenched.3 Three sets of reaction temperatures and annealing temperatures were employed. The reaction temperatures were 600 °C, 700 °C and 800 °C and the annealing temperatures were 800 °C, 900 °C and 1000 °C.
2.2 Characterization
X-Ray Diffraction (XRD) was performed with a Rigaku Ultima IV powder microdiffractometer (Cu Kα radiation). For the scanning electron microscopy analysis, the sample was dispersed in ethanol and a drop of this suspension was deposited onto a holey carbon grid. The MoS2 plates were characterized using a Hitachi S-5500 scanning electron microscope (SEM) with a bright-field/dark-field (BF/DF) duo STEM detector. To obtain the Raman spectrum, a high resolution confocal microscope (HORIBA Jobin Yvon) was used with a source of monochromatic radiation of 785 nm. For Raman measurements a 10× objective and a laser power of 185 mW were used, the signal detection was achieved through the use of a sensitive charge coupled device (CCD) array detector.
TEM was carried out with a JEOL JEM-2010F (FEG-TEM) operated at 200 kV with a 0.1 nm lattice resolution, which was employed to record high resolution (HRTEM) images, electron diffraction (ED) patterns and to perform the EDX analysis of the materials. For the aberration (Cs) corrected characterization, the samples were analyzed using a JEOL ARM (200F) 200 kV FEG-STEM/TEM, equipped with a CEOS Cs corrector on the illumination system. The probe size used for acquiring the HAADF as well as the BF-STEM images was 9C (23.2 pA) and the CL aperture size was 40 μm. High angle annular dark-field (HAADF) STEM images were acquired with a camera length of 8 cm/6 cm and a collection angle of 68–280 mrad/90–270 mrad was used. The BF-STEM images were obtained using a 3 mm/1 mm aperture and a collection angle of 17 mrad/5.6 mrad was used (camera length in this case was 8 cm). The HAADF as well as the BF images were acquired using a digiscan camera. In order to reduce the noise of the images and to obtain clearer images the raw data were filtered using the 2D Wiener filter and the Richardson–Lucy/Maximum Entropy algorithm implemented by Ishizuka.32 The EDAX analysis was performed using the EDAX instrumentation attached to the JEOL-ARM microscope. Spectra, line scans as well as chemical maps for the various elements were obtained using the EDAX genesis software. For the EDAX analysis the probe size used was 6C (145 pA) and the CL aperture size was 40 μm.
The catalytic properties were carried out by measuring the hydrodesulfurization (HDS) of dibenzothiophene (DBT), which was tested in a high pressure 300 mL Parr reactor by placing 4.4 g of DBT, 100 mL of decalin and 0.30 g of the MoS2 plates. The reactor was purged of residual air, pressurized with H2 to 3.1 MPa (450 psi) and then heated to the reaction temperature of 623 K in about 10 min. A stirring rate of 600 rpm was used. The advance of the reaction was monitored by gas chromatography with a HP 6890 gas chromatography, using samples taken every 20 min during the first hour, then every 30 min for the next four hours. Reduction of the sample volume due to sampling was ≤5% of the total volume. The identity of the reaction products was confirmed by mass spectrometry with a HP 6890 GC-MS, using a HP-5MS capillary column (30 m × 0.25 mm × 0.25 μm). Catalytic activity was expressed in terms of % conversion of DBTvs. reaction time and from these data, the reaction rates were calculated for each catalyst. The mean standard deviation for the catalytic measurements was about 2.5%.
2.3 Theoretical calculations
To explain the Moiré patterns produced by the rotated 2H-MoS2 plates, theoretical analysis using an extended Hückel tight-binding method was performed while the average total energy of the systems was monitored throughout the experiment. The calculations reported in this work have been carried out by means of the tight-binding method33 within the extended Hückel34 framework using YAeHMOP computer package with f-orbitals.35 The extended Hückel method is a semiempirical approach for solving the Schrödinger equation for a system of electrons, based on the variational theorem. In this approach, explicit electron correlation is not considered except for the intrinsic contributions included in the parameter set. More details about the mathematical formulation of this method have been described elsewhere36 and will be omitted here. Theoretical calculations were based on the crystalline 2H-MoS2 using the following primitive vectors: a = 3.1612 Å, c = 12.2985 Å and space groupP63/mmc (194).37 Atomic parameters for Mo and S atoms used in our calculation were obtained from Álvarez et al.38 Experimental lattice parameters instead of optimized values were used searching for the best match between the theoretical results and the available experimental information.
3. Results and discussion
In Fig. 1 the XRD spectrum of the MoS2 synthesized at 700 °C and annealed at 1000 °C is shown. This sample was the most crystalline sample and had no impurities of MoO3 and oxysulfides. The peaks observed correspond to the hexagonal phase of MoS2 (2H-MoS2). The reflections of MoS2 have been indexed to the hexagonal structure of the material with lattice constants: a = 3.1612 Å, c = 12.2985 Å (JCPPS card No. 00-037-1492). The (002), (101), (102), (103), (006), (105), (110), (008), (201), (203), (116) reflections are all sharp, indicating that the sample is crystalline, as shown in Fig. 1, where the reflections of the 2H polytype are shown. Fig. 1 also shows the reflections of the 3R polymorph of MoS2, although it shows the (002) peak, the peaks above and below 40° do not match, also as the peaks around 50°, then we conclude that the phase of this material is 2H and not 3R. The XRD spectra of all the other samples are shown in Fig. S1 (ESI†).
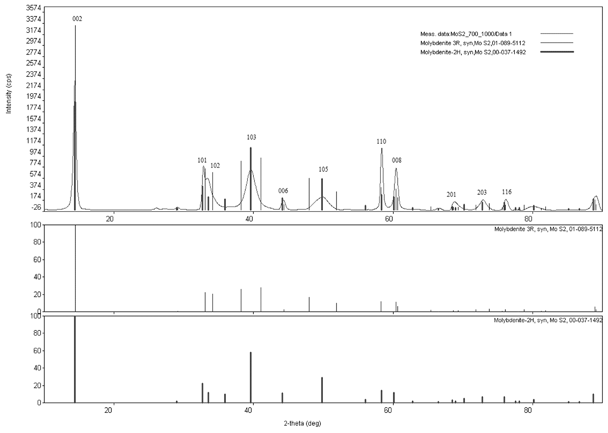 |
| Fig. 1
XRD patterns of the MoS2 plate synthesized at 700 °C and annealed at 1000 °C. The reaction time was 2.5 hours. The thin lines show the reflections corresponding to the 3R polymorph, while the broad lines represent the reflections corresponding to the 2H polymorphs. It should be noted that the best match corresponds to the 2H phase, the 3R phase has two peaks near 40° and two more near 50° that do not appear in the experimental XRD pattern. | |
Fig. 2a and b show the SEM micrographs of the MoS2 plates synthesized at 700 °C and annealed at 900 °C and 1000 °C, respectively. Both the images show the presence of hexagonal plates with nanometric size in the sample. The size of the plates is approximately 30–35 nm in diameter and the plates are hexagonal in shape. In Fig. 2a and b, the hexagonal plates are stacked in agglomerates consisting of the plates, which can be distinguished individually, these agglomerates can be broken by ultrasonication and then the individual platelets would make this material to have a high surface area. These temperatures were found to be the conditions that yielded the highest amount of hexagonal nanoplates, the micrographs of the samples synthesized at 600 °C and 800 °C are shown in Fig. S2 (ESI†).
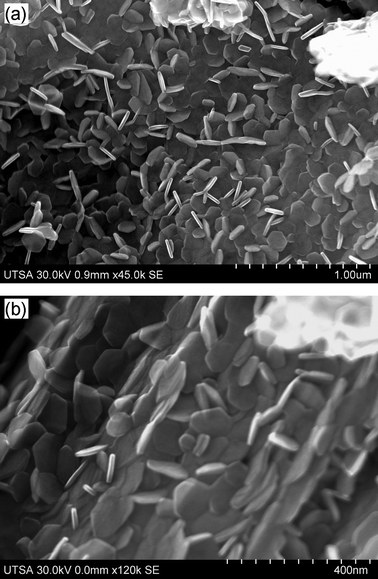 |
| Fig. 2
SEM micrographs of the MoS2 plates synthesized and annealed at: (a) 700 °C–900 °C; (b) 700 °C–1000 °C. These samples had the highest amount of plates. | |
The MoS2 plates were characterized in detail by Raman spectroscopy. Fig. 3 presents the Raman spectra of the MoS2 plate synthesized at 700 °C for 2.5 h of reaction time and annealed for 1 h at 1000 °C. This spectrum revealed peaks at 222 cm−1, 380 cm−1, 412 cm−1, 453 cm−1 and 630 cm−1; those peaks correspond to MoS2 in the hexagonal phase,41 except for the 222 cm−1. The spectrum is similar to the one displayed by MoS2 ribbons.42 In the figure it is seen that there is a peak at 380 cm−1, corresponding to the E12g, which is characteristic of hexagonal MoS2. At 412 cm−1 the A1g peak is present. The peak at 220 cm−1 corresponds to the J2 peak39 and is not normally seen in the Raman spectrum of 2H-MoS2 crystals.40,41 The appearance of the peak J2 can be explained in terms of the existence of a superlattice.39,43 This indicates that the particle size and crystal structure modification in these nanoparticles have brought about great changes to the electronic states.20 The Raman spectrum does not show the peaks corresponding to MoO3 or sulfur oxides, so it is concluded that the conversion is complete and the product is chemically stable during laser beam irradiation and does not oxidize during the analysis.42 The samples synthesized with lower reaction times showed peaks associated with sulfur oxides, the spectra and discussion of them are shown in Fig. S3 (ESI†).
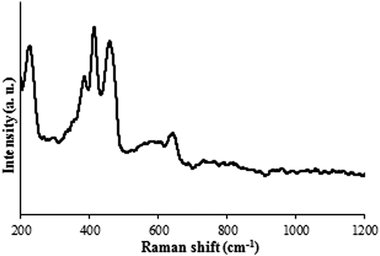 |
| Fig. 3
Raman spectra of the MoS2 plate synthesized at 700 °C and annealed at 1000 °C. The reaction time was 2.5 h. | |
Fig. 4a presents a TEM micrograph of a MoS2 plate at high resolution, it shows in detail the stacks of Mo; with this image it was possible to measure the Mo–Mo distances in the a1, a2 and a3 directions. Distances of 2.76 Å in the a1 direction, 2.8 Å in the a2 direction and 2.87 Å in the a3 direction are obtained, these values correspond to Mo–Mo interatomic distances.44 A line profile is shown in the inset of Fig. 4a. Thus the hexagonal atomic structure of MoS2 is observed with a resolution sufficient enough to resolve the Mo–Mo interatomic distance. This result is consistent with previous observations of a nanocatalyst.45 The images obtained here show a contrast based only on the atomic number Z and the thickeners of the sample. Therefore, the atomic positions and the distances between Mo–Mo that we have obtained are direct and reliable. Fig. 4b shows the diffraction pattern of Fig. 4a, it was obtained with the FFT, the a1, a2 and a3 directions are indicated in the figure; it is possible to clearly see the crystal array of MoS2, corresponding to a hexagonal crystal system. From Fig. 4b was obtained the cleavage of the crystal, it was 〈001〉.
In Fig. 5, three-plates of MoS2 are shown, forming a Moiré pattern composed by the three individual plates of MoS2. The upper inset of Fig. 5 shows a high resolution TEM micrograph of a Moiré pattern, a Moiré lattice is observed; the lower inset shows the Fast Fourier Transform (FFT) of the plates, three sets of hexagonal spots can be seen, corresponding to the three plates; the angles between the plates are measured in the figure, the angle between the first two plates is 19°, and the angle between the next two plates is 16°. These are the rotational angles of the plates, here three plates were found and their superimposition caused the Moiré pattern. Some of the nanoparticles observed contained Moiré patterns of three plates and some of them with two plates. There are also cases where there was no Moiré pattern (single plates). Fig. 6 shows a high resolution TEM micrograph of a MoS2 plate and its interior. The basal surface shows a Moiré pattern, the plate shown has a lateral cut showing the interior of the nanoparticle. The inset in the left shows the diffraction pattern of the interior, it was possible to obtain the direction of the crystal, that results to be [000n], where n is even. The inset in the right shows an amplification of the main image, several bands of different sizes are observed, the broad lines are molybdenum, as it has a high atomic number and a high atomic weight, the thin lines are sulfur, as the lines are regular and consist of a broad, thin, broad, thin, etc., then what the image is showing is the stacks of S–Mo–S forming sandwiches,16 three of these layers form an unit cell in MoS2,43 one of which is remarked in the inset of Fig. 6, we measured the distance between three atoms of Mo conforming a cell unit and the result, c, was 0.12 nm or 1.2 Å, this result agrees with the parameters obtained with the XRD, and with the reported value of c/2 = 6.53 Å in the literature;43,44 it should be remarked that between every atom of molybdenum there are two of sulfur, then the thin lines represent two stacks of atoms of sulfur one in front of each other. The orientation of the layers is obtained and shown in the inset in the right of Fig. 6.
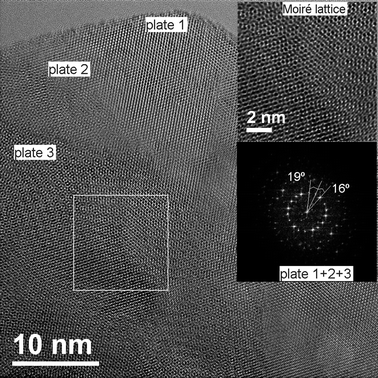 |
| Fig. 5 Image showing the superimposition of three plates of MoS2 and the formation of a Moiré pattern. The upper inset shows a high resolution TEM (HRTEM) micrograph of the Moiré pattern and the lattice. The lower inset shows the electron diffraction pattern with three sets of points representing the three plates, the angles between the plates are 19° and 16°. | |
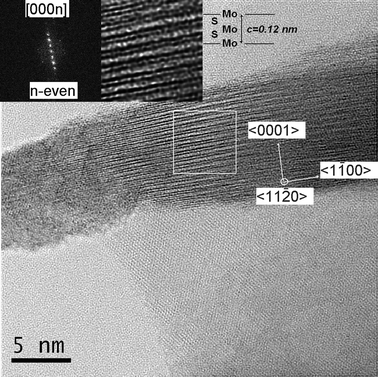 |
| Fig. 6 Bright field aberration corrected HRSTEM image of a plate with the self-assembled MoS2 layers. The inset in the left shows the Fast Fourier Transform in the 〈11−20〉 zone axis and the inset in the right shows a zoom of the self assembled unit cell (c = 1.2 Å). The stripes consist of stacks of Mo and of S. | |
EDAX
chemical maps (Fig. 7) indicate the presence of Mo (Mo(K,L)) and S(K) map indicating that the material is effectively composed of Mo and S with an atomic ratio of 1
:
2 between Mo and S. This indicates that the conversion is complete and there is no oxidation of the plates during the process (700–1000 °C and 2.5 hours of reaction).
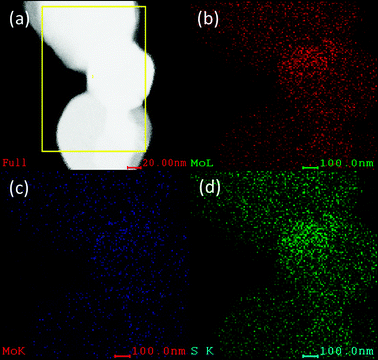 |
| Fig. 7 (a) EDX mapping of the MoS2 plates (b) showing the characteristic Mo(L), (c) Mo(K) and (d) S(K). The ratio of Mo and S is found to be 1 : 2. | |
3.5
Catalytic activity
The HDS of DBT yields biphenyl (BP) through the direct desulfurization pathway (DDS), and cyclohexylbenzene (CHB) and tetrahydrodibenzothiophene (THDBT) through the hydrogenation pathway (HYD). Since these two pathways are parallel, (HYD)/(DDS) selectivity was calculated by the equation:46
The average composition of reaction solution after 5 h is [CHB] = 14.2% and [BP] = 35.0%. These values produce a ratio of 0.4, which means the catalysts have a greater selectivity for BP, the desulfurization route. DBT conversion versus time was plotted and the reaction rate was calculated as the slope m = 1.53 × 10−3, assuming pseudo-zero order kinetics. The rate constant, K, was then calculated as 20.3 × 10−7 mol g−1 s, using:
K = m (1/60) (1/C) (mol. wt. DBT) |
where, m = reaction rate, 1/60 = conversion factor from minutes to seconds, C = mass of the catalyst, 4.4 g DBT and mol. wt. DBT = 184.26 g mol−1.
Fig. 8 shows the plot of the reaction time versus the conversion, the reaction rate was found as the slope, m = 1.53 × 10−3. This reaction rate is comparable to that of MoS2 obtained by thermal decomposition of ammonium thiomolybdate,47 more than three times higher than MoS2 catalysts from alkylthiomolybdates,48 and roughly 10 times greater than those reported for MoS2 nanorods, for the same model reaction and conditions.49 Hexagonal nanoplates are therefore deemed very active. Their selectivity, however, differs from the direct hydrogenation pathway preferred by the other catalysts.
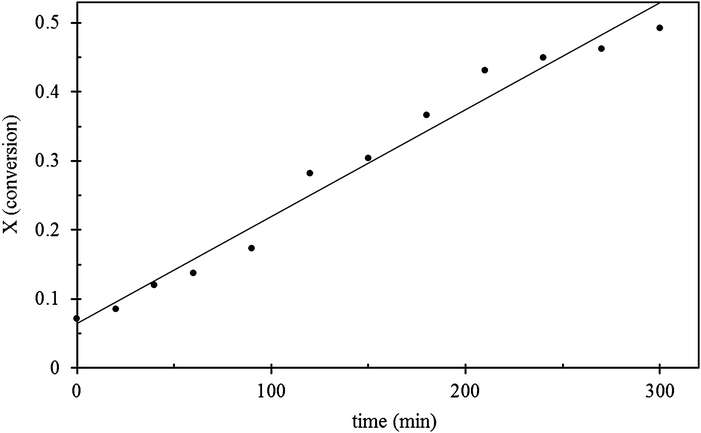 |
| Fig. 8 Graph of the HDS of DBT using MoS2 plates as catalyst, showing the plot of time vs. conversion. | |
3.6 Theoretical analysis
To explain the diffraction patterns in unrotated 2H-MoS2 and rotated 2H-MoS2 we proceed as follows: considering the average total energy of the system without rotation and after the rotation was applied, and taking into consideration that one of the layers is maintained fixed (a Mo layer) while the other layers (two sandwiches consisting of layers of S–Mo–S) were rotated by 16° and 19°, respectively, around the first Mo layer. Band structure calculations for the 2H-MoS2 with no rotation and 2H-MoS2 when a rotation of 16° and 19° were applied to two planes and performed using 51k points for each case, and sampling the First Brillouin Zone (FBZ) as depicted in Fig. 9a and b. It is well known that 2H-MoS2 crystalline bands are split into three sub-bands separated by a gap ranging from 1 to 1.9 eV. These different values were reported by several authors like Mattheis50 and Grant et al.51 Energy bands for 2H-MoS2 unrotated is depicted in Fig. 9a, while Fig. 9b yields energy bands for 2H-MoS2 when rotations of the MoS2 planes were applied. The Fermi level is indicated by a horizontal dotted line separating the valence band (VB) from the conduction band (CB) respectively. Energy in eV vs. k-values were plotted for each case, ranging from Γ (0 0 0) to K (1/3 1/3 0) to M (1/2 0 0) to Γ (0 0 0) to A (0 0 1/2) to L (1/2 0 1/2) to A (0 0 1/2) of π/a.
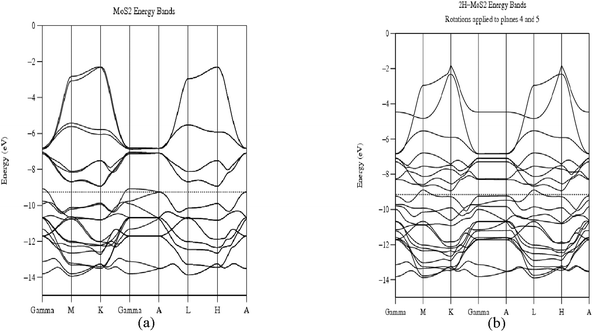 |
| Fig. 9 (a) and (b) Band structure calculations for unrotated 2H-MoS2 and 2H-MoS2 when two MoS2 sandwiches had been rotated by 16° and 19° around the first MoS2 plate. | |
The results obtained are provided in Table 1. The table provides information about the analyzed structure. Total average energy (eV) and the forbidden energy gap Eg (eV) for the two cases enunciated in the former paragraph. First, notice that for the unrotated 2H-MoS2, the average total energy is −495.2760 eV and looking at Fig. 9a the system is a semiconductor with a forbidden (indirect between K–Γ) energy gap Eg = 2.0 eV. Second, Fig. 9b for 2H-MoS2 rotated two MoS2 planes by 16° and 19°, the average energy is −495.9270 eV, similar to the unrotated case, which indicates that the rotation applied to two MoS2 planes was not severe enough to maintain the system in a similar space group as the original one, favoring this rotated state which provides confirmation that the Moiré patterns observed are as stable as the original unrotated case. On the other hand notice, also that the forbidden energy gap decreases to Eg ≈ 0.38 eV (direct gap in M) providing indication of a small gap semiconductor. The tendency of the rotated 2H-MoS2 toward the metallic behavior has been correlated by Topsøe and his group17,18 including our group52 relating MoS2 clusters to the enhancement of the catalytic activity in these systems, this rotation and the tendency to a metallic behavior improves the catalytic activity of the material compared to bulk MoS2 and to molybdenum disulfide with no rotation of layers.
Table 1 Total average energy (eV), Eg (eV) and behavior for 2H-MoS2 unrotated and rotated by 16° and 19°
Structure |
Figure |
Total average energy/eV |
E
g/eV |
Behavior |
2H-MoS2 unrotated |
9a
|
−495.2760 |
2.0 |
Semiconductor |
|
|
|
Indirect in Γ–M |
|
2H-MoS2 a rotation of 16° and 19° applied to two S planes |
9b
|
−495.9270 |
0.38 |
Semiconductor |
|
|
|
Direct in M |
|
4. Conclusions
Regular hexagonal MoS2 nanoparticles have been synthesized and their catalytic activity was tested. XRD patterns and the Raman spectrum showed peaks of 2H-MoS2. SEM micrographs showed nanoparticles of 30 nm in size, and that increasing annealing temperature leads to more crystalline samples. This was supported by XRD analysis, the best temperature of synthesis was 700 °C and the best annealing temperature was 1000 °C. The Raman analysis showed that the MoS2 plates are in the hexagonal phase with no impurities if the reaction time is 2.5 or 3 hours; lower times will lead to the presence of MoO3 and oxygen sulfides. It is interesting to note that the samples with most nanohexagonal plates were those synthesized at 700 °C, the plates synthesized at 600 °C were amorphous and those synthesized at 800 °C showed the presence of rods. Of the annealing temperatures studied, the best one was 1000 °C, because it led to more crystalline samples and to a higher amount of hexagonal plates with nanometric size, the annealing temperature of 800 °C had little influence on conformation. HRTEM showed that the Mo–Mo interatomic distance was about 2.8 Å, consistent with the distances measured in the literature.44HRTEM also revealed the 2H-MoS2 structure. There are Moiré patterns generated with two plates and with three plates. The measured rotation angle in the trilayer MoS2 was found to be 19° and 16°, this is due to the lack of AB stacking registry between the layers,53 and the different layers are typically in turbostrating stacking.54 The unit cell was measured with HRTEM and it was 0.12 nm, this technique also allowed to characterize the edges of the plates, showing that HRTEM allows a complete, detailed and accurate characterization of these nanoparticles. The selectivity and activity of the catalyst are very high, corresponding not to an unpromoted MoS2 catalyst but almost that of a doped MoS2 with cobalt. Theoretical analysis were performed on unrotated as well as rotated 2H-MoS2 and monitoring the total average energy in both cases yielded indication that the Moiré patterns observed in the rotated case are in stable states. On the other hand, energy bands on both systems indicate that for the rotated case the system indicates a trend toward the metallicity of the system, this is not observed when the system is unrotated, which could explain the enhancement in the catalytic properties. Such improved catalysts will lead to a cleaner gasoline and to a cheaper HDS process.
Acknowledgements
To CONACyT for their grant with key number 119826. We also acknowledge the NSF foundation for support through PREM program grant DMR-0934218. To the UTSA and the CNN for allowing us the use of their facilities.
Notes and references
- C. T. Tye and K. J. Smith, Top. Catal., 2006, 37, 2 Search PubMed.
- J. R. Lince, Tribol. Lett., 2004, 3, 17 Search PubMed.
- Z. Wu, D. Wang, X. Liang and A. K. Sun, J. Cryst. Growth, 2010, 312, 1973 Search PubMed.
- J. Chen, N. Kuriyama, H. Yuan, H. T. Takeshita and T. Sakai, J. Am. Chem. Soc., 2001, 123, 11813 CrossRef CAS.
- Z. Zak, Y. Feldman, V. Lyakhovitskaya, G. Leitus, R. Popovitz-Biro, E. Wachtel, H. Cohen, S. Reich and R. Tenne, J. Am. Chem. Soc., 2002, 124, 4747 CrossRef CAS.
- M. Remskar, Z. Skraba, P. Stadelmann and F. Levy, Adv. Mater., 2000, 12, 814 CrossRef CAS.
- M. Homyonfer, B. Alperson, Y. Rosenberg, L. Sapir, S. R. Cohen, G. Hodes and R. Tenne, J. Am. Chem. Soc., 1997, 119, 2693 CrossRef CAS.
- A. Rotschild, S. R. Cohen and R. Tenne, Appl. Phys. Lett., 1999, 75, 4025 CrossRef CAS.
- N. Imanishi, K. Kanamura and Z. Takehara, J. Electrochem. Soc., 1992, 139, 2082 CAS.
- J. Chen, S. L. Li, Q. Xu and K. Tanaka, Chem. Commun., 2002, 1722 RSC.
- G. L. Frey, R. Tenne, M. J. Matthews, M. S. Dresselhaus and G. Dresselhaus, Phys. Rev. B: Condens. Matter, 1999, 60, 2883 Search PubMed.
- N. Zink, J. Pansiot, J. Kieffer, H. A. Therese, M. Panthöfer, F. Rocker, U. Kolb and W. Tremel, Chem. Mater., 2007, 19, 6391 CrossRef CAS.
- W. Tremel and E. W. Finckh, Chem. Unserer Zeit, 2004, 38, 326 Search PubMed.
- W. Tremel, R. Seshadri and E. W. Finckh, Chem. Unserer Zeit, 2001, 35, 42 Search PubMed.
- S. Ross and A. Sussman, J. Phys. Chem., 1955, 59, 889 Search PubMed.
- S. Helveg, J. V. Lauritsen, E. Lægsgaard, I. Stensgaard, J. K. Nørskov, B. S. Clausen, H. Topsøe and F. Besenbacher, Phys. Rev. Lett., 2000, 84, 951 CrossRef CAS.
- J. V. Lauritsen, M. V. Bollinger, E. Lægsgaard, K. W. Jacobsen, J. K. Nørskov, B. S. Clausen, H. Topsøe and F. Besenbacher, J. Catal., 2004, 221, 510 CrossRef CAS.
- J. V. Lauritsen, M. Nyberg, R. T. Vang, M. V. Bollinger, B. S. Clausen, H. Topsøe, K. W. Jacobsen, E. Lægsgaard, J. K. Nørskov and F. Besenbacher, Nanotechnology, 2003, 14, 385 CrossRef CAS.
- L. S. Byskov, J. K. Nørskov, B. S. Clausen and H. Topsøe, J. Catal., 1999, 187, 109 CrossRef CAS.
- X. L. Li, J. P. Ge and Y. D. Li, Chem.–Eur. J., 2004, 10, 6163 Search PubMed.
- F. L. Deepak, A. Mayoral and M. José-Yacamán, Mater. Chem. Phys., 2009, 118, 392 CrossRef CAS.
- X. Zheng, L. Zhu, A. Yan, C. Bai and Y. Xie, Ultrason. Sonochem., 2004, 11, 83 Search PubMed.
- Y. Tian, Y. He and Y. F. Zhu, Mater. Chem. Phys., 2004, 87, 87 CrossRef CAS.
- Y. Feldman, E. Wasserman, J. Srolovitz and R. Tenne, Science, 1995, 267, 222 CrossRef CAS.
- W. J. Li, E. W. Shi, J. M. Ko, Z. Z. Chen, H. Ogino and T. Fukuka, J. Cryst. Growth, 2003, 250, 418 CrossRef CAS.
- R. H. Wei, H. B. Yang, K. Du, W. Y. Fu, Y. M. Tian, Q. J. Yu, S. K. Liu, M. H. Li and G. T. Zou, Mater. Chem. Phys., 2008, 108, 188 Search PubMed.
- V. G. Pol, S. V. Pol and A. Gedanken, Cryst. Growth Des., 2008, 8, 1126 Search PubMed.
- Z. Z. Wu, D. Z. Wang and A. K. Sun, J. Cryst. Growth, 2010, 312, 340 Search PubMed.
- L. Ma, W. X. Chen, H. Li and Z. D. Xu, Mater. Chem. Phys., 2009, 116, 400 Search PubMed.
- Z. Z. Wu, D. Z. Wang and A. K. Sun, J. Mater. Sci., 2010, 45, 182 Search PubMed.
- G. A. Camacho-Bragado and M. José-Yacamán, Appl. Phys. A: Solid Surf., 2006, 82, 19 CrossRef CAS.
-
HREM Research, Inc., http://www.hremresearch.com.
- M. H. Whangbo and R. Hoffmann, J. Am. Chem. Soc., 1978, 100, 6093 CrossRef CAS.
- R. Hoffmann, J. Chem. Phys., 1963, 39, 1397 CrossRef CAS.
- G. A. Landrum, The YAeHMOP package is freely available on www at: http//overlap.chem.Cornell.edu:8080/yaehmop.html. F orbitals are included in the calculations as version 3.0x, using W. V. Glassey's routine. W. V. Glassey, G. A. Popoian and R. Hoffman, J. Chem. Phys., 1999, 111, 893 Search PubMed.
- D. H. Galvan, J. Mater. Sci. Lett., 1998, 17, 805 Search PubMed.
- P. D. Fleischauer, R. Jeffrey, P. Lince, A. Bertrand and R. Bauer, Langmuir, 1989, 5, 1009 Search PubMed.
-
S. Álvarez, Tables of Parameters for Extended Huckel Calculations, Universitat de Barcelona, 1993 Search PubMed.
- S. Jiménez-Sandoval, D. Yang, R. F. Frindt and J. C. Irwin, Phys. Rev. B: Condens. Matter, 1991, 44, 3955 CrossRef.
- J. M. Chen and C. S. Wang, Solid State Commun., 1974, 14, 857 Search PubMed.
- T. J. Wieting and J. L. Verble, Phys. Rev. B: Solid State, 1971, 3, 4286 CrossRef.
- M. Virsek, A. Resih, I. Milosevic, M. Damnjanovic and M. Remskar, Surf. Sci., 2007, 607, 2868 Search PubMed.
- D. Yang, S. Jiménez-Sandoval, W. M. R. Divigalpitiya, J. C. Irwin and R. F. Frindt, Phys. Rev. B: Condens. Matter, 1991, 43, 12053 CrossRef CAS.
- X. R. Qin, D. Yang, R. F. Frindt and J. C. Irwin, Phys. Rev. B: Condens. Matter, 1991, 44, 3940 Search PubMed.
- C. Kisielowski, Q. R. Ramasse, L. P. Hansen, M. Brorson, A. Carlsson, A. L. Molenbroek, H. Topsoe and S. Helveg, Angew. Chem., Int. Ed., 2010, 49, 2708 Search PubMed.
- D. D. Whitehurst, T. Isoda and I. Mochida, Adv. Catal., 1998, 42, 3450 Search PubMed.
- R. Romero-Rivera, A. G. Camacho, M. Del Valle, G. Alonso, S. Fuentes and J. Cruz-Reyes, Top. Catal., 2011 DOI:10.1007/s11244-011-9620-2.
- G. Alonso-Núñez, G. Berhault and R. R. Chianelli, Inorg. Chim. Acta, 2001, 316, 468 Search PubMed.
- M. A. Albiter, R. Huirache-Acuña, F. Paraguay-Delgado, J. L. Rico and G. Alonso-Núñez, Nanotechnology, 2006, 17, 3473 Search PubMed.
- L. F. Mattheis, Phys. Rev. B: Solid State, 1973, 8, 3719 CrossRef CAS.
- A. J. Grant, T. M. Griffiths, G. D. Pitts and A. D. Yoffe, J. Phys. C: Solid State Phys., 1975, 8, L17 Search PubMed.
- M. José-Yacamán, H. López, P. Santiago, D. H. Galvan, I. L. Garzón and A. Reyes, Appl. Phys. Lett., 1996, 69, 1065 CrossRef CAS.
- J. H. Warner, M. H. Rümmeli, T. Gemming, B. Büchner and G. A. D. Briggs, Nano Lett., 2009, 9, 102 CrossRef CAS.
- L. Ci, L. Song, C. Jin, D. Jariwala, D. Wu, Y. Li, A. Srivastava, Z. F. Wang, K. Storr, L. Balicas, F. Liu and P. M. Ajayan, Nat. Mater., 2010, 9, 430 CrossRef CAS.
Footnote |
† Electronic supplementary information (ESI) available. See DOI: 10.1039/c1cy00055a |
|
This journal is © The Royal Society of Chemistry 2011 |