A mesoporous WN co-doped titania nanomaterial with enhanced photocatalytic aqueous nitrate removal activity under visible light†
Received
3rd February 2011
, Accepted 29th March 2011
First published on 28th April 2011
Abstract
This manuscript deals with the increased and selective nitrate reduction under visible light over a WN co-doped titania photocatalyst. A series of WN co-doped titania nanomaterials were synthesized with varying tungsten content and were characterized by TEM, XRD, UV-vis and nitrogen adsorption–desorption studies. Tungsten doped materials are found to be noodle shaped and mesoporous with a stable anatase phase after 500 °C calcination. Peak shifting in the XRD pattern indicates the tungsten doping in the crystal lattice. Visible light absorption increases with the increasing tungsten amount. Among the studied hole scavengers, formic acid was found to be the best for nitrate reduction with more than 94% nitrogen gas selectivity. The amount of hole scavenger also controls the reaction efficiency and product selectivity. Both nitrate reduction and nitrogen gas selectivity increases with WN co-doping in comparison to only N doped titania. High nitrate reduction with formic acid is attributed to the formation of CO2˙− species having high reduction potential. The material with 2% tungsten shows the highest surface area and the best photocatalytic activity under visible light. Presence of anion like chloride increases the nitrate photoreduction. Overall high nitrate reduction can be attributed to the synergistic effect of tungsten and nitrogen co-doping, optimum surface hydroxyl group, mesoporosity and appreciable visible light absorption of the materials.
1. Introduction
With the rapid development of industry and agriculture, nitrate pollution in groundwater has been one of the most serious environmental problems.1,2Nitrate is very mobile in the environment and cannot be held up well in any type of materials. The strong oxidizing power of nitrate causes detrimental effects in the environment. Nitrate and its metabolites are toxic to human health and are particularly harmful to infants. The WHO recommends a maximum nitrate concentration of 10 mg L−1 in drinking water. Conventional water treatment is not effective in removing nitrate in water due to its better solubility and stability than other pollutants. Catalytic reduction to nitrogen gas proposed in recent years is a feasible method to remove nitrate.3 Therefore, liquid-phase catalyticnitrate hydrogenation has been extensively studied since 1989, when a Pd–Cu bimetallic catalyst was found to be active by Vorlop et al.4 However, the formation of by-products, nitrites and/or ammonium, is generally too high with respect to the European standards applied to drinking water, which are [NO2˙−] < 0.1 mg L−1 (0.002 mmol L−1) and [NH4+] < 0.5 mg L−1 (0.028 mmol L−1). Furthermore, the formation of undesired products, the safety of hydrogen, and the efficient contact among three phases are still major problems to be faced in future applications. In this context, a negative view of application prospects of liquid phase catalytic hydrogenation and conventional methods like reverse osmosis as alternatives were expressed by Ruiz-Beviá,5 but they were soon defended by Corma.6 Therefore, nitrate removal still is an important challenge which needs to be looked into seriously by different green approach.
Nowadays the most interesting application of titanium dioxide is its photocatalytic activity for environment protection since it can decompose a large number of organic and inorganic pollutants.7–11 However, because of the wide band gap of titanium dioxide, only a small fraction of solar light (3–5%) can be utilized. Many researchers have paid much attention on ion doped photocatalysts since modification of TiO2 with various ions is a powerful way to extend the light absorption from UV to visible area. Since the first report of Asahi et al. on high visible light photocatalytic activities of nitrogen doped titanium oxide,12 many efforts have been made by doping of titanium dioxide with nitrogen and other nonmetals, such as B, C, S, and F, so as to efficiently extend the photoresponse from UV to visible light.13–15 It was commonly reported that the improvement of the photocatalyst was attributed to the decrease of the band gap, which is due to the mixture of the states of non-metals with O2p on the top of the valence band. Besides that, another key factor that affects the wide application of the photocatalyst is its catalytic efficiency, which is controlled by the electron/hole recombination rate, as it controls the availability of photo-excited sites on the catalyst surface.16,17 It is widely accepted that doping metal ions with changing valence is an optimal method to increase the anatase phase stabilisation and decrease the recombination rate.18–21 In this context a series of zirconium,22tungsten23 and cerium24 doped titania materials were studied with improved photocatalytic activity. Recently it was demonstrated that tungsten doping can extend the visible light absorption of the titania material to the visible light range and prohibit the hole electron pair recombination, thus increasing the photocatalytic activity.25 In particular, doping of nitrogen with a metal on titania will increase the potential use of indoor or solar light. Gao et al. prepared a series of N-doped TiO2 coupled with WO3 showing higher photocatalytic activity under both UV and visible light.26 However, there should be further improvement in their works since these preparation processes were too complicated and the TiO2–N–WO3 material prepared from immersing TiO2–N into tungstic acid may result in most of WO3 adsorbed on the TiO2 surface instead of inserted into the TiO2 lattice, which may accelerate the photo-electron and holes recombination rate and decrease the photocatalytic activity. So there is a need to look into new method of synthesis for tungsten and nitrogen co-doped TiO2 as a potential catalyst under visible light.
Though photocatalysis under visible/solar light is a green process but limited studies were carried out on the photocatalytic nitrate reduction process.27–29 Metal doped titania is found to reduce nitrate selectively and efficiently under UV light.28 Mostly silver doped titania is reported to be the better catalyst for nitrate reduction. Recently Zn doped WO3 is reported as a potential photocatalyst under visible light.30 Due to the importance of solar light mediated photoreduction process it is highly imperative to find out an appropriate photocatalyst which can efficiently reduce nitrate to nitrogen gas. However, the present photocatalytic nitrate removal study is mainly limited to the metal doped titania only which operates under UV light. Therefore, there is a requirement to find out a suitable visible light sensitive photocatalyst for the said reduction process for future application. To the best of our knowledge NW co-doped titania material is not studied for the nitrate reduction reaction till date. Here we report the detail investigation of NW co-doped mesoporous titania material for enhanced nitrate reduction with high nitrogen gas selectivity (> 94%) under visible light. Importance of W and N co-doping, hole scavenger and the effect of other anion is evaluated for better understanding of the reduction process.
2. Experimental
2.1 Material synthesis
Tungsten doped titanium oxides (WTi) were synthesized by varying the tungsten amount from 2–10 wt% through controlled hydrolysis of titanium isopropoxide and ammonium tungstate (Aldrich) at pH 4.0. Titanium isopropoxide in required amount was diluted to 20 ml with n-propanol and slowly added to 100 mL of aqueous solution of ammonium tungstate at pH = 4.0 under stirring condition at 27 °C. Nitrogen doping of WTi suspension was achieved by adding ammonia to the suspension up to the pH 9 and the mixture was kept under stirring condition for another 12 h. Subsequently the mixture was slowly evaporated up to drying. Obtained N-doped materials (WTiN) were calcined at different temperatures for further use. For comparison, N doped pure titania (TiN) was synthesized following the same procedure. In the sample code number indicates the wt% of tungsten doped in the material.
2.2. Material characterisation
XRD patterns of all the synthesized samples calcined at different temperatures were recorded on a Siemens (model: D-500) semiautomatic diffractometer using Cu-Kα radiation source in the range of 20–80°.
Samples for TEM measurements were prepared by dispersion of obtained powdered samples in isopropanol and subsequently placing a drop of the colloidal solution on carbon coated copper TEM grid. The film on the TEM grid was allowed to dry prior to the measurement. TEM measurements were performed on a JEOL (2100F field Emission TEM) instrument operated at an accelerating voltage of 120 kV.
UV-vis
diffuse reflectance spectra of all the synthesized materials were recorded on the Shimadzu 2550 spectrophotometer using BaSO4 as the reference material.
Raman spectra of the powdered materials were recorded on Nicolet Almega XR (Thermo) dispersion Raman spectroscopy.
X-Ray photoelectron spectra (XPS) was recorded by UHV analysis system (SPECS, Germany) with an Mg-Kα twin anode X-ray source (E = 1253.6 eV). The instrument was operated at a pressure 6 × 10−9 Torr in the analysis chamber. Photoelectrons were collected with pass energy of 80 eV for surveys, and 20 eV for high-resolution spectra. The binding energies were collected with a reference to the maximum intensity of the C 1s (285.0 eV). Analysis of the spectra was done using CASA XPS software.
BET surface area was determined by N2 adsorption desorption method at liquid nitrogen temperature using Nova 4000e (Quantachrome, USA). Prior to adsorption desorption measurements, the samples were degassed at 473 K at 10−3 Torr for 5 h.
2.3 Photocatalytic activity
Prepared materials after calcination at 500 °C were used as photocatalyst for the reductive removal of nitrate. Stock solution of nitrate was prepared by dissolving required amount of potassium nitrate salts in double distilled water. All the photocatalytic experiments were carried out in a 200 mL capacity three necked glass reactor centrally fitted with 125 W high pressure Hg lamp under magnetic stirring condition. A constant reaction temperature was maintained through water circulation in the outer jacket of the glass reactor. All the experiments were performed in the presence of air at atmospheric pressure maintaining 0.1 g per 100 mL of catalyst. The initial concentration of nitrate was maintained at 80 ppm in each experiment. During reaction nitrate is reduced to nitrite, nitrogen gas and ammonia. Concentrations of nitrite, nitrate and ammonium ions were estimated using advanced ion chromatography (Model-861, Metrohm). Nitrogen gas collected in air bag during reaction is also estimated by GC (NUCON-5765, India) with TCD detector. For kinetic experiment around 10 mL of the reaction mixture was pipetted out at different interval of reaction time. From the reaction mixture solid catalyst was separated by filtration or centrifuge. The effect of anion was studied with the use of required amount of KCl to maintain the concentration at 40 ppm.
3. Results & discussion
3.1 Textural properties
The TEM image (Fig. 1) of the material shows an elongated noodle type structure having a width of 100–150 nm. The enlarged image shows that the noodles are composed of nanoparticles of 10–20 nm. The phase structure, crystallite size and crystallinity of TiO2 play an important role in the photocatalytic activity and many studies have confirmed that the anatase phase of titania shows higher photocatalytic activity than brookite or rutile phase.31XRD was used to investigate the changes of phase structure of TiO2 samples prepared with different ammonium tungstate addition. XRD patterns of synthesized materials calcined at 500 °C for 4 h with varying W concentration are presented in Fig. 2. The major crystalline phase detected in all samples is anatase. It is worth noting that no WO3 phase could be observed in the XRD patterns, even the doping amount reaches 10 wt%. As seen in the inset, the 101 peak shifts towards lower d value in all the WN co-doped materials when compared to the pure anatase titania. Shifting is same irrespective of increased tungsten amount. Shifting anatase (101) peaks towards higher two theta value indicates the contraction of d in the lattice. This can be explained in case of W doping as the ionic radius of W6+ (60 pm) is lower than the Ti4+ (68 pm). This confirms the tungsten doping in the anatase crystal. Again with the increasing tungsten amount, crystallinity of the materials decreases. Thus, we can propose that some of the tungsten ions are incorporated into the titania lattice and replace titanium ions to form W–O–Ti bonds. The rest of the tungsten atoms are located at grain boundaries as tungsten oxide.
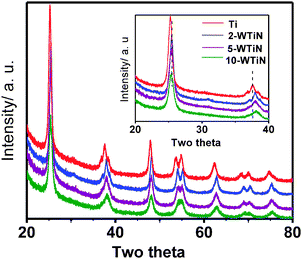 |
| Fig. 2
XRD pattern of the synthesized materials calcined at 500 °C. | |
The average size of crystallites was estimated based on the broadening of (101) peak at 2θ = 25.38 using the Scherrer equation, d = kλ/B(2θ)cosθ, where k is a shape factor of the particle (it equals to 0.89 if a spherical shape is assumed), λ and θ the wavelength and the incident angle of the X-rays, and B the full width half maximum (FWHM) of the peak, respectively. A change in the crystallite (Table 1) size is negligible when the materials are synthesized under same procedure. So the tungsten amount has no major role on the crystallite size.
Table 1 Surface area and crystallite size data of the synthesized materials
Samples |
Average pore radius/Å |
Pore volume/cc g−1 |
Surface area/m2 g−1 |
Crystallite size/nm |
TiN |
59.2 |
0.181 |
55 |
13.8 |
2-WTiN
|
18.2 |
0.238 |
100.6 |
13.8 |
5-WTiN
|
18.0 |
0.153 |
67 |
13.7 |
10-WTiN
|
18.1 |
0.079 |
35.6 |
11.2 |
Degussa P25 |
16.0 |
0.16 |
51 |
21.0 |
Fig. 3(a) shows the Raman spectra of TiO2 and WTiN samples in the 100–1000 cm−1 region. The observed characteristic bands at 144, 196, 396, 516, and 638 cm−1, assigned to the Eg, B1g, A1g, B2g, and Eg vibrational modes of TiO2, respectively, indicating the presence of the anatase phase in all these samples.32Fig. 3(b) presents the Raman spectra of the WTiN samples in the region of 700–1100 cm−1. Small peak at 795 cm−1 is observed for tungsten-doped samples, which is attributed to the second-order feature of anatase TiO2,33 while the broad peak within 925–950 cm−1 is attributed to the symmetrical W
O stretching mode of the dispersed tungsten oxide species on the surface of TiO2.34 This broad peak is mostly observed for 10-WTiN sample only. This confirms the dispersion of excess tungsten oxide on the surface of anatase phase and hence XRD does not show any WO3 peaks.
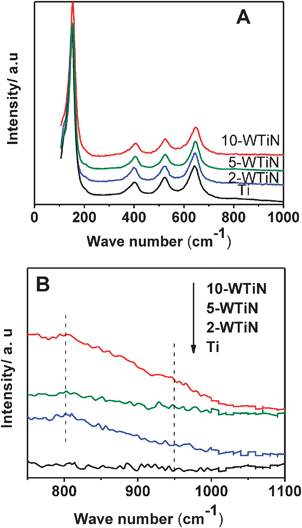 |
| Fig. 3
Raman spectra of synthesized materials (a) full scale and (b) enlarged view in the range of 700–1100 cm−1. | |
In the UV-vis spectra (Fig. 4) there is little change in absorption towards the visible light range due to W doping. However, the colour of the material changes to light yellow after ammonia addition and also shows absorption in the visible light range. This change in colour, after calcination, indicates the nitrogen doping in the material. In particular, visible light absorption of NW co-doped material increases with the increasing W concentration. So it can be concluded that the visible light absorption of the material not only depends on N doping but also on the tungsten co-doping. Appreciable visible light absorption indicates that WN co-doped materials can be used as a photocatalyst under visible/solar illumination.
3.2 Surface properties
Since it is widely accepted that heterogeneous photocatalysts are influenced by the surface area and pore structure, we carefully investigated the effects of the tungsten doping amount on the pore structure and BET surface area of the synthesized samples based on nitrogen adsorption and desorption measurements. Table 1 lists the physicochemical properties of W, N doped TiO2 series with different W concentration. Fig. 5(a) shows the nitrogen adsorption–desorption isotherm of pure and W,N co-doped TiO2 samples, which is type-IV like isotherm indicating the presence of mesoporosity in the synthesized materials. Moreover, Fig. 5(b) shows the pore size distribution plots calculated using the BJH equation from the desorption branch of the isotherm. The pore size distribution measurement indicates that the noodle type W, N co-doped titania sample has pronounced porosity with narrow pore size distribution in a range of 10–40 Å. Whereas pore size distribution in case of pure titania extends up to 100 Å. With 2 wt% tungsten doping surface area increases nearly two fold. However, further increasing the tungsten amount decreases the surface area and hence 10-WTiN shows the least surface area. An increased surface area with narrow pore size distribution will certainly contribute to the photocatalytic activity due to the availability of more surface active sites. It is clearly marked that due to the W doping average pore size shifts towards the narrow range. It can be inferred that tungsten doping in the lattice helps in the creation of porous network with narrow pores. An increase in the tungsten amount above 2 wt% results in the accumulation of tungsten oxide in the pores thereby decreasing the surface area drastically. This result illustrates that doping with W and N species does significantly change the textural properties of TiO2. Some amount of W embedded in the TiO2 network thus restructuring the pores and hence pore size distribution shifts towards the narrow range.
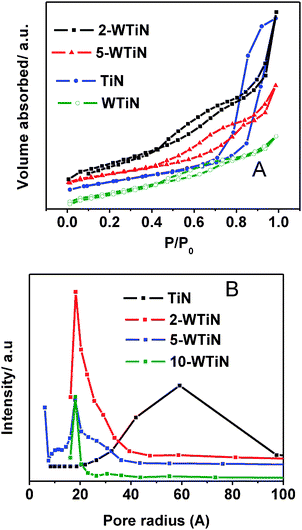 |
| Fig. 5 N2 adsorption desorption (a) isotherms and (b) pore size distribution plot. | |
3.3 Surface chemical properties (XPS)
XPS spectra of O1s of pure titania and the 2-WTiN is presented in Fig. 6. Two peaks are observed at 529.9 and 532 eV. Interestingly intensity of the 532 signal is high for the tungsten material in comparison to pure TiO2. This shoulder at 532 is due to the presence of hydroxyl group and hence indicates the increased hydroxyl concentration due to tungstate doping. This may suggest that a greater adsorption of hydroxyl groups or water take place due to increasing W concentration. Keller et al.35 reported that tungsten doping of the TiO2 leads to the appearance of Lewis and Brønsted acid sites and therefore an increase of the surface acidity is observed. Such surfaces have a higher affinity to the species with unpaired electrons and can easily adsorb the hydroxyl groups and water, which are necessary for the hydroxyl radical generation thus facilitating the photocatalytic process. The high surface concentration of hydroxyl group is also associated with the high photocatalytic activity. O1s peak of 2-WTiN also shifts towards the higher binding energy compared to pure titania.
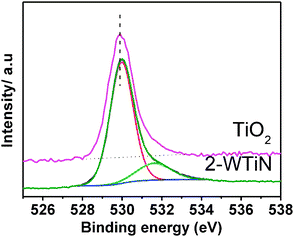 |
| Fig. 6 O1s XPS spectra of 2-WTiN and pure titania materials. | |
The assignment of the nitrogen 1s peak in the XPS spectra is under debate in the literature. Mostly the N 1s peak at 397 eV is due to the nitrogen doping in the titania lattice.12 Whereas the formation of stable NO species22,36 gives rise to a peak at 401 eV. In both cases visible light absorption increases thus enhancing the visible light photocatalytic activity. N 1s spectrum (Fig. 7(a)) shows a single peak at 401 eV, which is mostly due to the presence of NO species. This broadly confirms that the nitrogen is doped into the material. The W (4f) doublet of the 2-WTiN sample annealed at 500 °C (Fig. 7(b)) showed an unusual asymmetry due to overlapping with the Ti (3p) component. After convolution presence of W 4f5/2 and 4f7/2 is clear justifying the W doping in the material.
According to literature data, in WOx/ZrO2 system, where all W atoms are in octahedral coordination, the BE values reported for W6+ and W5+ atoms are 35.5 ± 0.2 and 34.6 ± 0.3 eV, respectively.37 Peak at 35.7 eV clearly supports the presence of W6+ in the material.
3.4 Role of hole scavenger
A preliminary experiment shows that the nitrate reduction is quite low without the use of hole scavenger. The addition of a hole scavenger increases the reduction activity to a substantial level. This justifies the major role played by hole scavenger in the reduction process. In this context a range of hole scavengers were tested and presented in Fig. 8, which confirms formic acid as the best hole scavenger. Obtained result is in consistent with the earlier reported one27 using metal modified TiO2 as the catalyst. Surprisingly, nitrate conversion is >98% with formic acid while with other hole scavengers conversion is below 10%. So simple hole scavenging is not enough to increase the reduction activity. It is believed that initial oxidation of formic acid takes place by the consumption of photo-degenerated hole to form CO2˙− species. The large reduction potential of the CO2˙− species [E0CO2/CO2˙− = 1.8 V]38 in comparison to photo-degenerated electron (E0 = 0.29 V) is the driving force for the efficient reduction of nitrate ions. It is well evident from eqn (1) that the CO2˙− species can be easily formed from formic acid in comparison to the other hole scavengers and hence the increased conversion of nitrate in similar condition. | HCOO− + H+ → H+ + CO2˙− | (1) |
Nitrogen gas selectivity is highest with the formic acid unlike the reported result for acetic acid over metal doped TiO2 (28). Hole scavenger concentration also plays an important role in the nitrate conversion and N2 selectivity (ESI, Fig. S1)†. With the initial increase in the formic acid concentration nitrate conversion and the nitrogen gas selectivity increases. However, increasing the hole scavenger amount above 200 ppm, conversion percentages slightly decreases though the nitrogen selectivity remains same. Therefore, for further studies, hole scavenger concentration is fixed at 200 ppm.
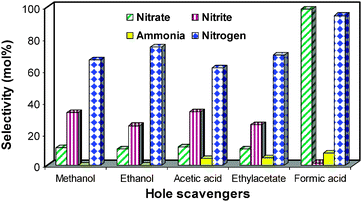 |
| Fig. 8 Effect of different hole scavengers on the nitrate reduction. | |
3.5 Photocatalytic activity
During the nitrate photocatalytic reduction reaction three types of products are formed with varying selectivity. Nitrite, ammonia and the nitrogen gas are the detected products. Most desirable product is nitrogen gas as it ensures the complete removal of nitrate. Other two products remain in the solution without minimising the toxicity. Due to the higher nitrate conversion only formic acid is used as the hole scavenger for the photocatalytic investigation. Performance of different synthesized catalysts was monitored varying reaction time and the product selectivity. It is observed (Fig. 9) that all the nitrogen doped materials show increased nitrate conversion in comparison to that of undoped material. In particular W and N co-doped materials performance is substantially higher in comparison to that of nitrogen doped TiO2. Even the product selectivity varies with the variation in catalyst material (ESI, Fig. S2).†Nitrogen gas selectivity is around 96% with 2-WTiN whereas the same selectivity is around 50% with TiN material. This emphasizes the importance of W doped material for increased conversion as well as nitrogen gas selectivity. However, increasing the tungsten amount beyond 2 wt% has detrimental effect. XRD and Raman spectra shows that a small amount of W6+ is doped into the lattice and the rest is available as homogeneously distributed tungsten oxide. The presence of tungsten oxide increases the acidity of the material thus facilitating the availability of surface OH group. This may be an optimum level of acidity that favours the nitrate reduction and any further increase or decrease has a detrimental effect on the photocatalytic conversion. This optimum surface hydroxyl is achieved with 2 wt% tungsten doping. It seems that increased surface acidity and hydroxyl group is quite essential for higher photocatalytic nitrate reduction. Therefore, tungsten doping facilitates the high rate of nitrate reduction in comparison to TiN. Increased surface area of 2-WTiN also facilitates the reduction reaction due to the availability of more surface active sites. It was observed that the visible light absorption increases with the increasing tungsten amount. However, nitrate reduction does not increase beyond the 2 wt% of tungsten. The 2-WTi material shows the least nitrate reduction as the visible light absorption is very low. Therefore, visible light absorption is required but the amount of visible light absorption is not the important criteria for the increased photocatalytic activity. Rather, the surface area and the optimum surface acidity play the crucial role in increasing the nitrate photoreduction activity.
The change in the product selectivity with reaction time is presented in the Fig. 10. Initial few minutes of reaction shows the high nitrite selectivity and low N2 selectivity. However after 20 min of reaction nitrogen selectivity approaches 90% and thereafter slowly increases to 94.6%. Ammonia selectivity nearly remains constant throughout the reaction. This indicates that nitrate reduction to nitrogen gas proceed vianitrite formation. Therefore initial selectivity of nitrite is high and these nitrite subsequently reduced to nitrogen gas. So the nitrate reduction can be expressed as bellow which is facilitated by the presence of CO2˙− species (eqn (2–4)).
| NO3− + HCO2− + H+ → NO2− + CO2 + H2O | (2) |
| NO3− + 3HCO2− + 7H+ → NH4+ + 3CO2 + 3H2O | (3) |
| 2NO3− + HCO2− + 11H+ → N2 + CO2 + 6H2O | (4) |
It is understood that both nitrite and
ammonia are undesirable products. So the importance of the present material lies in the
nitrogen gas selectivity which is around 95%.
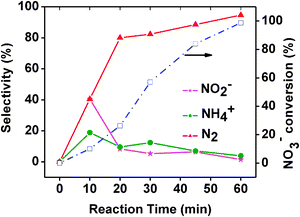 |
| Fig. 10 Correlation of nitrate photoreduction and product selectivity with reaction time over 2-WTiN photocatalyst. | |
Generally, chloride is present both in waste and drinking water with other anions and cations. The effect of interfering anions like chloride (40 ppm) on the nitrate reduction activity was studied and is presented in Fig. S3 (ESI).† It is observed that the nitrate reduction substantially increases in the presence of chloride ions. However, in the absence of formic acid chloride is unable to activate the reduction of nitrate. This indicates that chloride can not be used as a hole scavenger but in combination with the formic acid presence of chloride ion can enhance the reduction process. Moreover, the nitrogen gas selectivity slightly decreases in the presence of chloride ions. In this study, the oxidation of chloride to chlorine gas was not noticed. This is an interesting result and we are trying to evaluate the exact role of the chloride in the nitrate reduction process. Overall the increased nitrate removal with high nitrogen selectivity can be attributed to the synergistic effect of tungsten and nitrogen doping, surface hydroxyl group and mesoporosity.
4. Conclusion
Here we describe the synthesis of noodle shaped, mesoporous and thermally stable WN co-doped titanium oxide nanomaterials with comparatively high photocatalytic activity towards selective nitrate reduction under visible light. XRD and Raman spectra confirm the stabilisation of the anatase phase at 500 °C calcination with partial tungsten doping in the lattice. XPS confirm the nitrogen and tungsten doping in the material. It was observed that the visible light absorption increases with the increasing tungsten amount. Among the studied hole scavengers, formic acid is found to be the best one with 94.6% nitrogen gas selectivity. The amount of hole scavenger also controls the reaction efficiency and product selectivity. High nitrate reduction with formic acid is attributed to the formation of CO2˙− species having high reduction potential. Among all the materials 2-WTiN shows the highest surface area and nitrate photoconversion. It is observed that chloride ion can not be used as a hole scavenger but in combination with the formic acid, rate of the reduction process can enhance. Overall, the increased nitrate removal with high nitrogen gas selectivity over WN co-doped titania can be attributed to the synergistic effect of tungsten and nitrogen co-doping, surface hydroxyl group and mesoporosity. Present material can be further evaluated for potential use for nitrate removal from drinking water under solar light.
Acknowledgements
The authors are thankful to Dr S. Srikanth, Director, NML for his encouragement and permission to publish the work. We are also thankful to DST, India for providing funding for the project under the Green Chemistry Scheme.
References
- J. L. Costa, H. Massone and D. Martinez, Agric. Water Manage., 2002, 57, 33 CrossRef.
- J. Van Maanen, A. Van Dijk and K. Mulder, Toxicol. Lett., 1994, 72, 365 CrossRef CAS.
- A. Pintar, J. Batista and J. Levec, Water Sci. Technol., 1998, 37, 177 CrossRef CAS.
- K. D. Vorlop and T. Tacke, Chem. Ing. Tech., 1989, 61, 836 CrossRef CAS.
- F. Ruiz-Beviá, J. Catal., 2004, 227, 563 CrossRef CAS.
- A. Corma, A. E. Palomares, F. Rey and J. G. Prato, J. Catal., 2004, 227, 561 CrossRef CAS.
- L. Linsebigler, G. Q. Lu and J. T. Yates, Chem. Rev., 1995, 95, 735 CrossRef CAS.
- T. N. Fujishima and D. A. Rao, J. Photochem. Photobiol., C, 2000, 1, 1 CrossRef CAS.
- D. W. Kormann, M. Bahnemann and R. Hoffmann, J. Phys. Chem., 1988, 92, 5196 CrossRef CAS.
- S. Klosek and D. Raftery, J. Phys. Chem. B, 2001, 105, 2815 CrossRef CAS.
- S. Quadawi and S. R. Salman, J. Photochem. Photobiol. A, 2002, 48, 161.
- R. Asahi, T. Morikawa, T. Ohwaki, K. Aoki and Y. Taga, Science, 2001, 293, 269 CrossRef CAS.
- J. Ovenstone, J. Mater. Sci., 2001, 36, 1325 CrossRef CAS.
- J. Moon, H. Takagi, Y. Fujishiro and M. Awano, J. Mater. Sci., 2001, 36, 949 CrossRef CAS.
- J. C. Yu, J. G. Yu, W. K. Ho, Z. T. Jiang and L. Z. Zhang, Chem. Mater., 2002, 14, 3808 CrossRef CAS.
- V. Puddu, R. Mokaya and G. L. Puma, Chem. Commun., 2007, 4749 RSC.
- G. Marci, L. Palmisano, A. Sclafani, A. M. Venezia, R. Campostrini, G. Carturan, C. Martin, V. Rives and G. Solana, J. Chem. Soc., Faraday Trans., 1996, 92, 819 RSC.
- V. Keller, P. Bernhardt and F. Garin, J. Catal., 2003, 215, 129 CrossRef CAS.
- Y. T. Kwon, K. Y. Song, W. L. Lee, G. J. Choi and Y. R. Do, J. Catal., 2000, 191, 192 CrossRef.
- M. Miyauchi, A. Nakajima, K. Hashimoto and T. Watanabe, Adv. Mater., 2000, 12, 1923 CrossRef CAS.
- H. Tade, A. Kokubu, M. Iwasaki and S. Ito, Langmuir, 2004, 20, 4665 CrossRef CAS.
- N. Aman, T. Mishra, R. K. Sahu and J. P. Tiwari, J. Mater. Chem., 2010, 20, 10876 RSC.
- J. Li, J. Xu, W.-L. Dai, H. Li and K. Fan, Appl. Catal., B, 2008, 82, 233 CrossRef CAS.
- T.-Y. Ma, J.-L. Cao, G.-S. Shao, X.-J. Zhang and Z.-Y. Yuan, J. Phys. Chem. C, 2009, 113, 16658 CrossRef CAS.
- A. Khan, L. Sajjad, S. Shamaila, B. Tian, F. Chen and J. Zhang, Appl. Catal., B, 2009, 91, 397 CrossRef CAS.
- B. Gao, Y. Ma, Y. Cao, W. Yang and J. Yao, J. Phys. Chem. B, 2006, 110, 14391 CrossRef CAS.
- F. Zhang, R. Jin, J. Chen, C. Shao, W. Gao, L. Li and N. Guan, J. Catal., 2005, 232, 424 CrossRef CAS.
- J. Sa, C. A. Aguera, S. Gross and J. A. Anderson, Appl. Catal., B, 2009, 85, 192 CrossRef CAS.
- N. Wehbe, M. Jaafar, C. Guillard, J.-M. Herrmann, S. Miachon, E. Puzenat and N. Guilhaume, Appl. Catal., A, 2009, 368, 1 CrossRef CAS.
- X. F. Cheng, W. H. Leng, D. P. Liu, J. Q. Zhang and C. N. Cao, Chemosphere, 2007, 68, 1976 CrossRef CAS.
- O. Carp, C. L. Huisman and A. Reller, Prog. Solid State Chem., 2004, 32, 33 CrossRef CAS.
- H. Liu, W. Yang, Y. Ma, Y. Cao and J. N. Yao, New J. Chem., 2002, 26, 975 RSC.
- M. A. Vuurman, I. E. Wachs and A. M. Hirt, J. Phys. Chem., 1991, 95, 9928 CrossRef CAS.
- X. Li, M. Shen, X. Hong, H. Zhu, F. Gao, Y. Kong, L. Dong and Y. Chen, J. Phys. Chem. B, 2005, 109, 3949 CrossRef CAS.
- V. Keller, P. Bernhardt and F. Garin, J. Catal., 2003, 215, 129–138 CrossRef CAS.
- X. Chen, Y. Lou, A. C. S. Samia, C. Burda and J. L. Gole, Adv. Funct. Mater., 2005, 15, 41–49 CrossRef CAS.
- A. Chica, A. Corma and M. E. Domine, J. Catal., 2006, 242, 299–308 CrossRef CAS.
- D. M. Stanbury, Adv. Inorg. Chem., 33, 69–138 Search PubMed.
Footnote |
† Electronic supplementary information (ESI) available. See DOI: 10.1039/c1cy00042j |
|
This journal is © The Royal Society of Chemistry 2011 |