Application of fly ash supported titanium dioxide for phenol photodegradation in aqueous solution
Received
17th January 2011
, Accepted 13th April 2011
First published on 24th May 2011
Abstract
The photodegradation of phenol in aqueous solution using fly ash supported titanium dioxide (TiO2/fly ash) was investigated. The TiO2/fly ash was prepared by immobilization of TiO2 on municipal solid waste fly ash using sol–gel-adsorption method, followed by calcination at different temperatures from 300 to 600 °C in a nitrogen atmosphere. The effects of calcination temperature, photocatalyst dosage, initial concentration of phenol and irradiation time on the photodegradation of phenol were studied. It was found that phenol could be removed rapidly from water by TiO2/fly ash and the sample calcined at 500 °C exhibited the highest phenol photodegradation efficiency, the degradation percentage of phenol could reach 94.35% when irradiation time was 4 h, the photodegradation of phenol was obviously improved when the photocatalyst was used. Kinetics analysis showed that the photocatalytic degradation reaction could be described by a first-order rate equation. In addition, the possibility of cyclic usage of the photocatalyst was also confirmed, the photocatalytic activity of TiO2/fly ash remained ca. 90% of photocatalytic activity of the fresh sample after being used four times. Moreover, TiO2 was tightly bound to fly ash and could be easily handled and recovered from water.
1. Introduction
The health threat of phenol is well-known, and the presence of phenol derivatives in a wide range of locations from industrial waste water to agricultural refuse represents an important challenge for environmental protection. All of their structures have benzene rings, which present a strong inhibitive function for biological degradation, so they are very difficult to degrade into small inorganic molecules by using common methods. Degradation of these toxic substances by photocatalysis in aqueous medium could be the basis of a waste treatment method.1–4 Photocatalysis is a method based on irradiation of a solid semiconductor with light of the appropriate wavelength. The reason why the procedure is of high significance is that organic pollutants can be efficiently degraded by conversion of solar energy into chemical energy.5–7
Titanium dioxide, an inexpensive, non-toxic and biocompatible material, is one of the most important and widely investigated photocatalysts,8 because of its potential application in decomposition of various environmental pollutants in both gaseous and liquid phases.9–12 However, fine TiO2, which represents a high photoactivity and a significant quantum effect is commonly in nanometre size, and there are also problems of separation and recovery of photocatalyst from the reaction media, therefore, supported TiO2 is one of the choice for the field application of the photocatalyst. Much research has been devoted to immobilize TiO2 photocatalyst on porous supporting matrices, such as silica,13alumina,14zeolites15,16 and activated carbon (AC).17,18 In this study, we attempted to immobilize TiO2 particles on the surface of municipal solid waste fly ash via chemical processes and high temperature coating technique. The aims of our study are: (a) to develop a simple and repeatable anchoring procedure of the TiO2 nanophase to the fly ash, (b) to perform accurate characterization of the structure and properties of the composite obtained by means of sol–gel technique, (c) to check photostability of the TiO2 particles and of the supporting fly ash upon prolonged exposure to light, (d) to investigate the photodegradation of kinetics and (e) to check the reuse of the TiO2-covered fly ash for pollutant adsorption-photodegradation cycles.
2. Experimental
2.1 Materials preparation
The composition of municipal solid waste fly ash (mass fraction) used in this work was listed in Table 1. Fly ash was first soaked in an acid solution (HCl 1mol L−1) for 24 h, rinsed with deionized water and then dried at 80 °C for 48 h. Fly ash was coated before use.
Table 1 Composition of fly ash (mass fraction)
Composition |
SiO2 |
Al2O3 |
Fe2O3 |
TiO2 |
CaO
|
MgO
|
K2O
|
Na2O
|
CO2 |
SO3 |
P2O5 |
MnO
|
Mass fraction (%) |
55.58 |
26.67 |
8.59 |
1.72 |
1.70 |
1.22 |
1.09 |
0.73 |
0.50 |
0.38 |
0.22 |
0.067 |
TiO2 sol was prepared by sol–gel method using tetra-n-butyl titanate (Ti(OC4H9)4) as the precursor. 34.0 mL Ti (OC4H9)4 (C.P.) was dissolved in 60.0 mL anhydrous ethanol with stirring for 30 min. Another solution containing 56.7 mL anhydrous ethanol, 14.5 mL deionized water and 40.0 mL acetic acid was slowly added into the above solution under magnetic stirring at room temperature. The mixture was hydrolyzed for 60 min under vigorous stirring, and the transparent sol was obtained. The sol was aged for 6 h at room temperature, and then was added drop-by-drop onto 50.0 g fly ash to be adsorbed. Then, it was dried at 80 °C in an oven and, finally, calcined at different temperatures for 2 h in a nitrogen atmosphere. This prepared method was named as the sol–gel-adsorption method in the present work. The same procedure except for the adsorption process on the fly ash was used to synthesize pure TiO2 powders.
2.2 Characterization
The BET specific surface area and pore volume of the TiO2/fly ash composites were obtained by N2 adsorption at 77 K using a micromeritics 2000 instrument (ASAP 2000, Micromeritics). The morphologies of TiO2/fly ash and fly ash were examined by a scanning electron microscope (SEM, Holland Philips, JSM-5800).
2.3 Measurement of photocatalytic efficiency
The photoreaction was conducted in a 500 mL cylindrical vessel with a water cooled quartz jacket. Irradiation was provided by a 300 W ultraviolet mercury lamp with major emission at 365 nm, located in the center of the quartz jacket. A magnetic stirrer was equipped at the bottom of the reactor to achieve effective dispersion. Air was bubbled through the reaction solution from the bottom to ensure a constant dissolved O2 concentration. In the batch reactor the airflow was 4.0 mL min−1. The initial concentration of phenol was fixed at 80 mg L−1 and the temperature of the reaction solution maintained at ca. 20 °C in an ordinary photocatalytic degradation. The pH of the suspension was adjusted either with 0.1 mol L−1HCl or 0.1 mol L−1NaOH. Sixty minutes adsorption time in dark conditions was allowed before the start of the photoreactions. Then, samples of the suspension were withdrawn after a definite time interval and filtered through 0.45 micron filter paper. The filtrates were analyzed for residual phenol concentration using a UV-Vis spectrophotometer (UV762, Shanghai Analysis Company) at 269.5 nm. In order to compare the photocatalytic activity of TiO2/fly ash, the pure TiO2 powders were also tested. The amount of TiO2 powders were chosen as 1.0 g L−1, which was adequate under our conditions without disturbing the UV light entering the reactor. The TiO2/fly ash samples were used repeatedly, and each cycle lasted 240 min. Before the beginning of the next cycle, the remaining solution was replaced with 80 mg L−1 fresh solution. All the photoreaction experiments were carried out in duplicate and the results given are the means with a relative standard deviation of less than 5%.
3. Results and discussion
3.1 Characterization of photocatalyst
The microstructural changes of the TiO2/fly ash photocatalyst compared with fly ash were listed in Table 2. As shown in Table 2, the deposited TiO2 contributed to a slight increase in the surface area and total pore volume. The increase of average pore diameter was attributed to the calcinations at 500 °C in nitrogen atmosphere.
Table 2 Microstructure of original fly ash and TiO2/fly ash catalyst
Sample |
TiO2 loaded (mg g−1) |
BET surface area (m2 g−1) |
Total pore volume (cm3 g−1) |
Average pore diameter (nm) |
Fly ash |
0 |
1.159 |
0.073 |
20.3 |
TiO2/fly ash |
61.2 |
1.985 |
0.101 |
28.9 |
The SEM micrographs of TiO2/fly ash and fly ash were shown in Fig. 1. Fig. 1(a) shows the SEM micrograph of fly ash, the image of TiO2 immobilized on fly ash and calcined at 500 °C was shown in Fig. 1(b). It was observed that the uncoated fly ash has rough surfaces, but the surface of the coated one appeared to be rougher because of the deposited TiO2. This roughness contributed to the increase of the specific surface area already mentioned for the TiO2/fly ash.
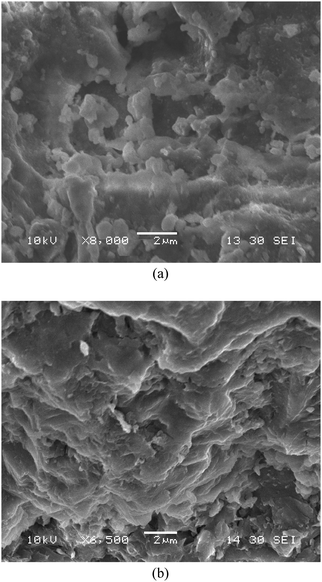 |
| Fig. 1
SEM images of samples. (a) fly ash; (b) TiO2/fly ash −500 °C. | |
3.2 Photocatalytic degradation of phenol
3.2.1 Effect of photocatalyst.
In order to evaluate the actual photocatalytic activity of the TiO2/fly ash photocatalyst calcined at 500 °C for 2 h, five phenol removal processes were compared, namely, photolysis, photocatalytic degradation by TiO2, pure fly ash, TiO2/fly ash, and adsorption of TiO2/fly ash, by corresponding experiments to assess the effect of catalyst on the overall degradation rate for an initial phenol concentration of 80 mg L−1), as shown in Fig. 2.
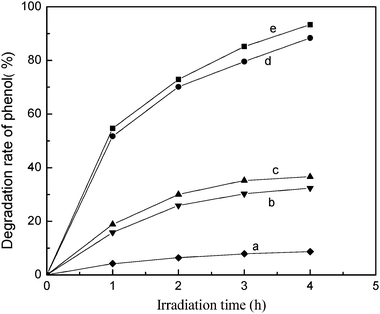 |
| Fig. 2 Effect of photocatalyst on the degradation rate of phenol. Initial conditions: phenol concentration = 80 mg L−1, pH = 4, calcinationT = 500 °C. (a) only with UV irradiation; (b) pure fly ash with UV irradiation; (c) TiO2/fly ash without UV irradiation; (d) TiO2 with UV irradiation; (e) TiO2/fly ash with UV irradiation. | |
Experimental results show that phenol could be degraded to a certain degree under UV irradiation [see Fig. 2(a)]. The adsorption of phenol on fly ash was saturated after 120 min under UV light irradiation, the degradation rate of phenol did not increase any further with prolonged UV light irradiation time. This indicated that pure fly ash does not have photocatalytic activity [see Fig. 2(b)]. Similarly, the TiO2/fly ash showed little photocatalytic activity without irradiation of UV light, and the saturated adsorption capacity of TiO2/fly ash is higher than that of pure fly ash [Fig. 2(c)]. This was a result of the higher surface area and polarity of the fly ash after the deposition of TiO2. The phenol degradation rates increased with UV light irradiation for the phenol/TiO2 system and phenol/TiO2/fly ash system, but the degradation percentages of phenol with TiO2/fly ash are higher than those of TiO2 [Fig. 2(d) and (e)]. By comparison of the amounts of phenol removed with and without UV (Fig. 2), it can be affirmed that the disappearance of phenol molecules was due to photocatalytic degradation instead of only to adsorption.
3.2.2 Effect of calcination temperature.
The photocatalytic activities of TiO2/fly ash samples at different calcination temperatures were shown in Fig. 3.
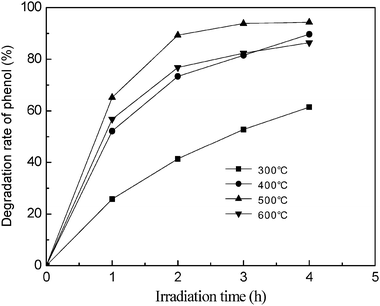 |
| Fig. 3 Effect of calcination temperature on the degradation rate of phenol. Initial conditions: phenol concentration = 80 mg L−1, photocatalyst dosage = 20 g L−1, pH = 4. | |
It can be seen that the calcination temperature significantly affected the photocatalytic activity of TiO2/fly ash. The samples exhibited some differences in phenol degradation rate, phenol degradation rate increased notably with increasing calcination temperature from 300 to 500 °C, but decreased when temperature was further raised to 600 °C, much in accordance with a previous report.19 This phenomenon can be attributed to the improvement in crystallinity of the anatase structure of TiO2 as calcination temperature increases. However, when the calcination temperature increases to 600 °C, the anatase is transformed to rutile, with a decrease in photocatalytic activity. Hence, the TiO2/fly ash used in the following experiments was all calcined at 500 °C.
3.2.3 Effect of photocatalyst dosage.
Photocatalytic activity increases with catalyst dosage, although, when the dosage exceeds the optimal amount, the suspension becomes too thick to admit enough light for photocatalysis. Fig. 4 shows the effect of TiO2/fly ash dosage (10–30 g L−1) on phenol degradation rates to increase markedly with catalyst dosage. At 20 g L−1, the degradation reaction rate is the highest, and this dosage was used in the following experiments.
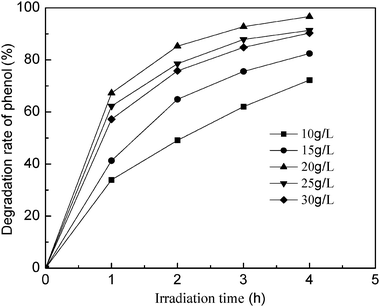 |
| Fig. 4 Effect of photocatalyst dosage on the degradation rate of phenol. Initial conditions: phenol concentration = 80 mg L−1, pH = 4, calcinationT = 500 °C. | |
3.2.4 Cyclic performances of TiO2/fly ash for the degradation of phenol.
In order to test the feasibility of the cyclic use of TiO2/fly ash photocatalyst, four cycles of photocatalytic degradation of phenol were carried out. The change in relative degradation percentage of phenol with cycling operation was shown in Fig. 5.
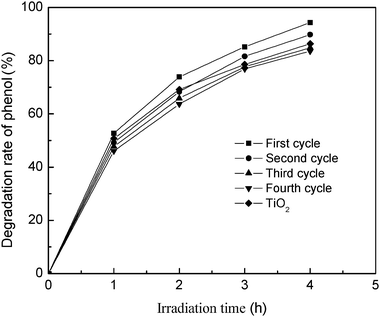 |
| Fig. 5 The cyclic performance of the photocatalyst. Initial conditions: phenol concentration = 80 mg L−1, pH = 4, photocatalyst dosage = 20 g L−1, calcinationT = 500 °C. | |
It was observed that phenol could be degraded by the present photocatalyst under UV irradiation. The photocatalytic reactivity of the present photocatalyst was just slightly reduced in stirred aqueous solution, and the TiO2/fly ash, after being used four times, remained at ca. 90% of photocatalytic activity of the fresh sample. The degradation percentage of phenol could reach 83.58% when the irradiation time was 4 h, and the amount of phenol removal was slightly lower than that for pure TiO2. Thus it is suggested that the deposited anatase TiO2 has firmly attached to the municipal solid waste fly ash surface, and can not be easily exfoliated from fly ash with mechanically stirred solutions for a long period. At the same time, it also proved that the final removal of phenol from solutions was caused by the photocatalytic degradation rather than the adsorption process that leads to saturated adsorption of phenol on the photocatalyst. These results indicated that cyclic usage of the TiO2/fly ash composite was possible and its stability in treating polluted water was satisfactory. Therefore, it is potentially employable for continuous photocatalytic degradation processes.
3.3
Phenol photocatalytic degradation kinetics and related parameters
The photocatalytic degradation of phenol was studied at various initial concentrations of phenol (C0). Fig. 6 shows the evolution of the concentrations of phenol in the aqueous TiO2/fly ash suspension during UV irradiation. As expected, phenol in the solution decreased with time at C0 = 7–80 mg L−1.
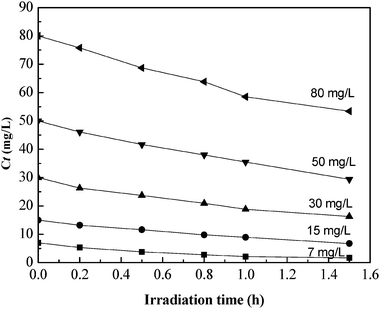 |
| Fig. 6 Kinetics of the photocatalytic degradation of phenol solution. | |
The kinetics of the degradation of phenol were investigated for different concentrations of phenol and an initial TiO2/fly ash concentration of 20 mg L−1. Fig. 6 shows that the concentration of phenol decreased exponentially during irradiation. Mathematically these results can be described by a first-order kinetic law, described by eqn (1):
where
Ct is the concentration of
phenol in solution at irradiation time
t and
k is the first-order rate constant.
According to this equation a linear plot of lnCtversus irradiation time is obtained [see Fig. 7], from which slope k can be estimated. Apparently, all the plots give linear relationships indicating that the photocatalytic degradation of phenol can be reasonably described by first-order kinetics. The coefficients of the first-order kinetics model are summarized in Table 3, showing that the squared correlation coefficient (R2) for all conditions is larger than 0.961, the observed first-order rate constant values decrease with increasing initial phenol concentration at constant TiO2/fly ash concentration, for initial phenol concentrations of 7–80 mg L−1k varied between 0.958 and 0.2222 h−1.
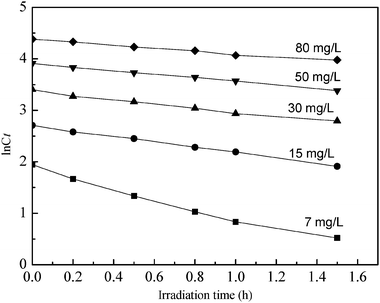 |
| Fig. 7 The linear plot of lnCtversus irradiation time. | |
Table 3 Kinetic parameters of the photocatalytic degradation of phenol solution
C
0 (mg L−1) |
k (h−1) |
t
1/2 (h) |
R
2
|
Kinetic equation |
7.0 |
0.959 |
0.723 |
0.961 |
lnCt = −0.959t + 1.861 |
15.0 |
0.523 |
1.325 |
0.996 |
lnCt = −0.523t + 2.701 |
30.0 |
0.404 |
1.716 |
0.974 |
lnCt = −0.404t + 3.370 |
50.0 |
0.347 |
1.998 |
0.998 |
lnCt = −0.347t + 3.909 |
80.0 |
0.278 |
2.494 |
0.978 |
lnCt = −0.278t + 4.376 |
The mechanism of phenol degradation reaction catalyzed by TiO2 is proposed as the following three steps: (1) adsorption of phenol on the surface of TiO2; (2) surface photodegradation of phenol; (3) desorption of final products from the surface of TiO2. For the photocatalysis of low concentration phenols, the photodegradation reaction of phenol predominantly proceeds with the mechanism of direct charge transfer from the semiconductor to the phenol adsorbed at the TiO2 center. Therefore, when the TiO2 catalyst was loaded onto the fly ash, phenol was selectively adsorbed on the fly ash, resulting in a higher pollutant environment around the loaded TiO2. Then the adsorbed phenol was transferred to the catalyst by diffusion and then decomposed there. After migration of the photocatalytic products from the TiO2 surface, the adsorption and photocatalytic abilities of the TiO2/fly ash system were regenerated.
In semiconductor photocatalysis, photogenerated holes (hvb+), electrons (ecb−), hydroxyl radicals (˙OH), and superoxide ions
may take part in redox reactions.20,21 The species hvb+, ecb−, ˙OH and
can thus oxidize phenol to intermediates, then the intermediates are further oxidized to CO2 and H2O. Under the conditions of the current study, the phenol degradation mechanistic pathways may be proposed as the following:
| 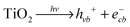 | (2) |
|  | (4) |
|  | (5) |
| phenol˙ + TiO2 → phenol˙+ + TiO2(e) | (6) |
|  | (7) |
It can be seen in
eqn (2)–(7) that the
phenol degradation reaction catalyzed by TiO
2/fly ash is not a simple elementary reaction, it is a complex reaction constituted of a series of chain reactions, so it was observed that the reaction rate constant values vary with increasing initial
phenol concentrations at constant TiO
2/fly ash concentration; the photocatalytic degradation reaction of
phenol is only an observed first-order reaction and
k is only the observed first-order rate constant.
4. Conclusions
In the present study, a supported photocatalyst was developed by immobilizing TiO2 onto municipal solid waste fly ash by sol–gel-adsorption method, followed by calcination in a nitrogen atmosphere. The TiO2 coating showed an enhancement of the adsorption of phenol in the photodegradation process. Photocatalytic degradation tests showed that phenol can be effectively degraded and mineralized by the synthesized TiO2/fly ash. The as-prepared TiO2/fly ash showed high photocatalytic activities in the photodegradation of phenol in comparison with pure fly ash. From the viewpoint of efficient mineralization of wastewater, the best dosage of TiO2/fly ash was 20 g L−1 and the degradation reaction of phenol can be described by first-order kinetics. Moreover, after repeated use for four cycles, the photocatalytic ability of TiO2/fly ash was just slightly reduced in stirred aqueous solution, and the separation of photocatalyst from solution was avoided. Therefore, it is potentially employable for continuous photocatalytic degradation processes.
Acknowledgements
This work was supported by National Natural Science Foundation of China (41073086) and Doctor Foundation of Shenyang University of Chemical Technology (No. 20063202).
References
- L. Körösi and I. Dėkány, Preparation and Investigation of Structural and Photocatalytic Properties of Phosphate Modified Titanium Dioxide, Colloids Surf., A, 2006, 280, 146–154 CrossRef.
- M. Ksibi, A. Zemzemi and R. Boukchina, Photocatalytic Degradability of Substituted Phenols over UV Irradiated TiO2, J. Photochem. Photobiol., A, 2003, 159, 61–70 Search PubMed.
- L. Palmisano, M. Sciavello, A. Sclafani, G. Martra, E. Borello and S. Coluccia, Photocatalytic Oxidation of Phenol on TiO2 Powders. A Fourier Transform Infrared Study, Appl. Catal., B, 1994, 3, 117–132 CrossRef CAS.
- A. Zaleska, J. W. Sobczak, E. Grabowska and J. Hupka, Preparation and Photocatalytic Activity of Boron-modified TiO2 Under UV and Visible Light, Appl. Catal., B, 2008, 78, 92–100 CrossRef CAS.
- M. R. Hoffmann, S. T. Martin, W. Choi and D. W. Bahnemann, Environmental Applications of Semiconductor Photocatalysis, Chem. Rev., 1995, 95, 69–96 CrossRef CAS.
- I. Ilisz, A. Dombi, K. Mogyorósi, A. Farkas and I. Dékány, Removal of 2-chlorophenol from Water by Adsorption Combined with TiO2 Photocatalysis, Appl. Catal., B, 2002, 39, 247–256 Search PubMed.
- J. Theurich, M. Lindner and D. W. Bahnemann, Photocatalytic Degradation of 4-chlorophenol in Aerated Aqueous Titanium-dioxide Suspensions: A Kinetic and Mechanistic Study, Langmuir, 1996, 12, 6368–6376 CrossRef CAS.
- B. Tryba, A. W. Phenolrawski and M. I. Nagaki, A New Route for Preparation of TiO2-mounted Activated Carbon, Appl. Catal., B, 2003, 46, 203–208 CrossRef CAS.
- M. Bissen, B. M. Vieillard, A. J. Schindelin and F. H. Frimmel, TiO2-catalyzed Photodegradation of Arsenite to Arsenate in Aqueous Samples, Chemosphere, 2001, 44, 751–757 CrossRef CAS.
- G. Guillard, S. Horikoshi, N. P. Watanabc, H. Hidaka and P. Pichat, Photocatalytic Degradation Mechanism for Heterocyclic Derivatives of Triazolidine and Triazole, J. Photochem. Photobiol., A, 2002, 149, 155–168 CrossRef CAS.
-
Photocatalytic Purification and Treatment of Water and Air, ed. D. F. Ollis and H. Al-Ekabi, Elsevier, Amsterdam, 1993 Search PubMed.
- J. Ryu and W. Choi, Effect of TiO2 Surface Modifications on Photocatalytic Degradation of MO: The Role of Superoxides, Environ. Sci. Technol., 2004, 38, 2928–2933 CrossRef CAS.
- L. J. Alemany, M. A. Banares, E. Pardo, F. Martin, M. Galan-Fereres and J. M. Blasco, Photodegradation of Phenol in Water Using Silica-supported Titania Catalysts, Appl. Catal., B, 1997, 13, 289–297 Search PubMed.
- W. Q. Huang, A. J. Duan, Z. Zhao, G. F. Wan, G. Y. Jiang, T. Dou, K. H. Chung and J. Liu, Ti-modified Alumina Supports Prepared by Sol–gel Method Used for Deep HDS Catalysts, Catal. Today, 2008, 131, 314–321 Search PubMed.
- S. Fukahori, H. Ichiura, T. Kitaoka and H. Tanaka, Photocatalytic Decomposition of Bisphenol A in Water Using Composite TiO2-zeolite Sheets Prepared by a Papermaking Technique, Environ. Sci. Technol., 2003, 37, 1048–1051 Search PubMed.
- Y. Hiromi and A. Masakazu, Local Structures and Photocatalytic Reactivities of the Titanium Oxide and Chromium Oxide Species Incorporated Within Micro- and Mesoporous Zeolite Materials: XAFS and Photoluminescence Studies, Curr. Opin. Solid State Mater. Sci., 2003, 7(6), 471–481 CrossRef CAS.
- J. M. Herrmann, J. Laine and J. Matos, Effect of the Type of Activated Carbons on the Photocatalytic Degradation of Aqueous Organic Pollutants by UV-irradiated Titania, J. Catal., 2001, 200, 10–20 CrossRef CAS.
- T. Tsumura, N. Kojitari, H. Umemura, M. Toyoda and M. Inagaki, Composites Between Photoactive Anatase-type TiO2 and Adsorptive Carbon, Appl. Surf. Sci., 2002, 196(1–4), 429–436 CrossRef CAS.
- R. S. Yuan, J. T. Zheng, R. B. Guan and Y. C. Zhao, Surface Characteristics and Photocatalytic Activity of TiO2 Loaded on Activated Carbon Fibers, Colloids Surf., A, 2005, 254, 131–136 Search PubMed.
- G. V. Buxton, C. L. Greenstock, W. P. Helman, A. B. Ross and W. Tsang, Critical Review of Rate Constants for Reactions of Hydrated Electrons Chemical Kinetic Data Base for Combustion Chemistry. Part 3: Propane, J. Phys. Chem. Ref. Data, 1988, 17(2), 513–886 CAS.
- M. M. Haque and M. Muneer, Photodegradation of Norfloxacin in Aqueous Suspensions of Titanium Dioxide, J. Hazard. Mater., 2007, 145, 51–57 Search PubMed.
|
This journal is © The Royal Society of Chemistry 2011 |