DOI:
10.1039/B915149B
(Critical Review)
Chem. Soc. Rev., 2011,
40, 340-362
The role of porphyrin chemistry in tumor imaging and photodynamic therapy
Received
5th January 2010
First published on 9th August 2010
Abstract
In recent years several review articles and books have been published on the use of porphyrin-based compounds in photodynamic therapy (PDT). This critical review is focused on (i) the basic concept of PDT, (ii) advantages of long-wavelength absorbing photosensitizers (PS), (iii) a brief discussion on recent advances in developing PDT agents, and (iv) the various synthetic strategies designed at the Roswell Park Cancer Institute, Buffalo, for developing highly effective long-wavelength PDT agents and their utility in constructing the conjugates with tumor-imaging and therapeutic potential (Theranostics). The clinical status of certain selected PDT agents is also summarized (205 references).
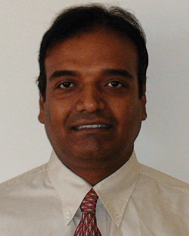
Manivannan Ethirajan
| Manivannan Ethirajan, PhD was born in Chennai, India. He obtained his PhD from IIT-Bombay. In 2001, he joined Prof. Gregory R. Cook's research group at NDSU, where he worked on small molecule inhibitors for MMP enzymes and then he moved to Prof. Jin K. Cha's research group at Wayne State University, where he focused on synthesizing carbocycles using palladium as a catalyst. Currently, he is working with Prof. Ravindra. K. Pandey at Roswell Park Cancer Institute and his research interest focuses on the synthesis of certain chemotherapeutic agents and porphyrin-based compounds, Silica and PAA-based nanoparticles for tumor-imaging and phototherapy (theronostics). |
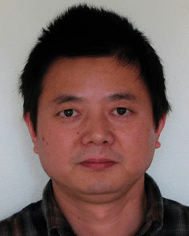
Yihui Chen
| Yihui Chen, PhD is a research scientist in Roswell Park Cancer Institute and research assistant professor in Nuclear Medicine Department of State University of New York at Buffalo. He obtained his BS degree from Jiangxi Normal University, China and MS degree from Chinese Academy of Sciences. In 2002, he finished his PhD from State University of New York under the tutelage of Professor Ravindra K. Pandey. His publications are widely cited and his research expertise is the interdisciplinary field of organic chemistry, nuclear chemistry, medicinal chemistry and molecular biology. |
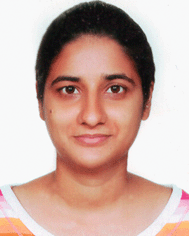
Penny Joshi
| Penny Joshi, PhD was born in Haldwani, India. She obtained her BS degree (2001) from Kumaun University, Nainital (India). After completing her Masters in Chemistry (2003) from University of Delhi, she joined Professor Diwan. S. Rawat, University of Delhi to earn her PhD in Chemistry (2008). Presently, she is working as a Post doctoral fellow with Prof. Ravindra. K. Pandey at Roswell Park Cancer Institute and her research work involves the synthesis of Porphyrin based “Multifunctional Agents” for tumor imaging with or without PDT. |
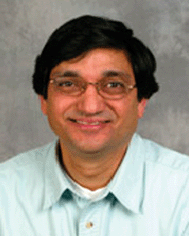
Ravindra K. Pandey
| Ravindra K. Pandey, PhD is currently a Distinguished Professor of the PDT Center and Director, Pharmaceutical Chemistry at Roswell Park Cancer Institute, Buffalo, NY, USA. His research work is mainly focused on developing (i) porphyrin-based compounds for tumor-imaging and therapy (PDT, chemotherapy) and (ii) nanoparticles as delivery vehicles. One of the photosensitizers synthesized by his research group is currently in Phase II clinical trials and a few PDT/imaging compounds are at advanced preclinical stages. Several therapeutic and imaging compounds developed in his laboratory are licensed to pharmaceutical companies for clinical trials and commercialization. |
A. Introduction
Cancer is one of the deadliest diseases of our time and throughout the world, billions of dollars are spent annually on research in order to cure or improve the life-standards of the cancer treatment. The traditional cancer treatments, including surgery, radiation therapy and chemotherapy, (Fig. 1) result in serious side effects caused by the loss of normal organ function.
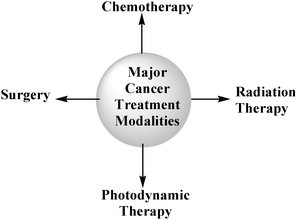 |
| Fig. 1 Major cancer treatment therapies. | |
In contrast, photodynamic therapy (PDT) is more controllable and has the potential to selectively destroy malignant cells while sparing the normal tissues. PDT, a relatively new therapy has several advantages over other traditional therapies: (i) it can be applied to places where surgery cannot be performed, (ii) the older patients and others who are vulnerable to surgery, chemotherapy or radiation therapy can be treated with this modality, (iii) the current treatment modalities, e.g., chemotherapy, often lead to nausea and vomiting and in some cases leading to depress the immune system which leads to various immune disorders. Similarly, cancer treatment by radiation also has side effects such as severe damage to epithelial surfaces, infertility, swelling of soft tissues, and other ill effects. On the other hand, the photosensitizers alone do not exhibit any organ toxicity. Their presence in liver and kidney for days or months do not produce any damage to these organs, (iv) it is relatively a non-invasive treatment and (v) it can be used either as a primary or adjunctive treatment for solid cancers of the bladder, esophagus, head and neck, brain, lung, prostate, intraperitoneal cavity, breast, prostate and skin.1–6
The application of PDT is not only limited to oncology, but is also being explored for the treatment of cardiovascular, dermatological, ophthalmic and infectious diseases.7
The PDT procedure consists of administering the photosensitizer to cancer patients by either intravenous or intraperitoneal injection. Depending on the pharmacokinetic characteristics of the drug(s), the tumor is irradiated with light at the time of its maximum drug uptake. This procedure ultimately produces singlet oxygen, a cytotoxic agent, which is believed to be responsible for tumor destruction (Fig. 1 and section C.1).7,8
When the photosensitizer returns to its ground state through inter system crossing, the energy is transferred to triplet molecular oxygen to produce singlet oxygen, which is extremely cytotoxic and is believed to play a major role in cell killing, promoting vascular shutdown leading to tumor destruction (Fig. 2).
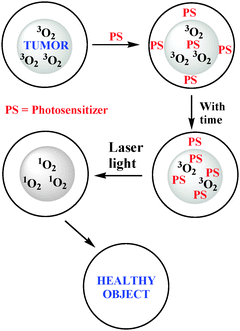 |
| Fig. 2 In PDT, singlet oxygen is a key cytotoxic agent that is responsible for the destruction of tumor. | |
In recent years, a large number of review articles on photodynamic therapy (PDT) have been published. Some of them deal with general discussions on PDT9–11 while others summarize the progress on specific aspects12–18 or its application in special fields such as dermatology19,20 Barrett's esophagus and other diseases.21–24 A few articles are focused on particular classes of photosensitizers including 5-aminolevulinic acid25,26 (ALA), phthalocyanines, naphthalocyanines27–30 and their metallated analogues such as aluminium, cobalt, gallium, silicon and zinc complexes27 or expanded porphyrins related to texaphyrin.31–36
The present article is mainly focused on (i) a brief history on PDT and its active component, (ii) a summary on approved photosensitizers or those which are at advanced stages of clinical trials and finally (iii) the strategies developed at the Roswell Park Cancer Institute, Buffalo for the development of effective PDT and multifunctional agents for the use in tumor-imaging (MRI, PET, fluorescence) and phototherapy.
B. A brief history of PDT-drug development
The first observation of chemical sensitization of tissue by light was reported in 1900 by Rabb.37 The idea of using light to treat cancer dates from 1903 when Tappeiner and Jesionek used topically applied eosin and sunlight to treat skin cancer patients. However, it was not until early 1980 when an active component of hematoporphyrin derivative (HpD) was isolated and partially identified.11,38 Additional studies by Roswell Park and other groups38–52,11,25 demonstrated that this “active” fraction of HpD (Photofrin®) consists of a mixture of porphyrin dimers and higher oligomers in which the porphyrin units are joined by ether, ester and carbon–carbon bonds. Since then, a rekindled interest in photodynamic action arose all over the world. Currently Photofrin® has been approved by health organization in the United States, Canada, Europe, and Japan, for the treatment of various types of cancers.11 Though Photofrin® is an effective photosensitizer, it suffers from several limitations, especially, skin photosensitivity and relatively weak long wavelength absorption which affords less than optimal light penetration depth. To overcome these problems, a set of second generation photosensitizers such as Levulan®, Radachlorin®, Visudyne® and Foscan® were developed in various laboratories. Radachlorin® is a product of Radapharma, Russia, which absorbs at 662 nm. Visudyne®, also known as verteporfin™ is a product of Novartis pharmaceuticals; the clinical studies revealed that it is quite effective for age-related macular degeneration (AMD). Foscan® is a chlorin based photosensitizer (652 nm) and is approved in Europe for the treatment of head and neck cancer patients. Certain chlorophyll-a and bacteriochlorophyll-ae.g. HPPH, purpurinimide, bacteriopurpurinimde and Tookad® are also at various stages of advanced clinical and preclinical studies (See the section Clinical Status of Selected Photosensitizers).
C. Active components of PDT
As discussed before, PDT requires three elements for an effective treatment: photosensitizer, light, and oxygen. Singlet oxygen is believed to be the main reactive oxygen species (ROS) which destroys tumor cells by necrotic and apoptotic cell-death mechanisms.
C.1 Singlet oxygen
Following the absorption of light, the photosensitizer is transformed from its ground singlet state (S0) into an electronically excited triplet state (T1, ∼10−2 s) via a short-lived excited singlet state (S1, ∼10−6 s). The excited triplet can undergo two kinds of reactions as shown in the Jablonski diagram (Fig. 3). First, it can participate in an electron-transfer process with a biological substrate to form radicals and radical ions, which, after interaction with oxygen, can produce oxygenated products such as superoxide ion, O2− (type I reaction). Alternatively, it can undergo a photochemical process known as a type II reaction, which results in the conversion of stable triplet oxygen (3O2) to the short-lived but highly reactive singlet oxygen (1O2), the putative cytotoxic agent. Singlet oxygen is produced by inverting the spin of one of the outer most electrons. For ground-state oxygen,25 the two electrons of highest energy reside separately in the outermost antibonding orbitals with same spin: (σ2p)2(π2px)2(π2py)2(π2px*)1(π2py*)1, while in the singlet oxygen, the two highest energy electrons reside together in the same antibonding orbital in opposite spin: (σ2p)2(π2px)2(π2py)2(π2px*)2.
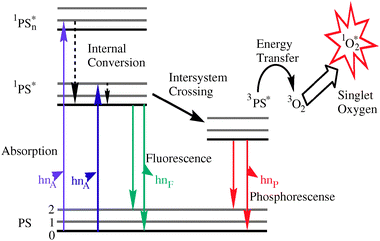 |
| Fig. 3 Modified Jablonski diagram showing the formation of singlet oxygen. | |
That is why singlet oxygen is much more reactive than ground state oxygen. In most organic solvents, the lifetime is 10–100 μs 26 (Fig. 3). This restricts its activity to a spherical volume 10 nm in diameter, centered at its point of production. In water, the lifetime of 1O2 is reduced to approximately 1–3 μs because the excited-state energy of singlet oxygen is almost equal to the energy of oxygen–hydrogen (O–H) stretching in water molecule and is easy to dissipate as heat by the stretching and vibrational motions of water molecules.25 However, the lifetime of 1O2 is considerably shorter in cellular systems, ranging from 100 ns in the lipid regions of membranes to 250 ns in the cytoplasm. Therefore, the diffusion range of 1O2 is predicted to be limited to approximately 45 nm in cellular media.53 Because the diameter of human cells ranges from approximately 10 to 100 μm, they cannot diffuse more than a single cell length. Thus, the site of the primary generation of 1O2 determines which sub cellular structures may be accessed and attacked. Singlet oxygen is a powerful, fairly indiscriminate oxidant that reacts with a variety of biological molecules. It is generally agreed that singlet oxygen is the key agent for cellular damage produced by irradiating the porphyrin-based photosensitizers (Fig. 4).53
C.2 Importance of tumor tissue oxygenation
As discussed above, for the Type II photochemical reactions ground state molecular oxygen (3O2) is necessary. When tumors are not well oxygenated, conditions such as anoxia and hypoxia have the potential to arise. During an anoxic condition the excited PS transfers its energy to organic substrates by oxidizing the substrate and reducing the PS. During a hypoxic condition the already reduced PS interacts with 3O2 to produce superoxide anions (O2−) and then eventually produces highly reactive hydroxyl radicals (OH˙).
Several research groups have suggested that the Type II reaction dominates, while Type I occurs only when the PS is highly concentrated in the tumor or if the tumor is hypoxic in nature. Therefore, the success of PDT has been weighted heavily upon the dependence or presence of oxygen within the tumor treatment site.54,55 Researchers have been observing oxygen diffusion models in which lower fluence rates generate singlet oxygen more efficiently so that more 1O2 is exposed to the tumor over longer periods of time. Busch et al., have demonstrated that oxygen is depleted as a function of fluence rate.56 Other conditions such as necrosis and vascular damage can also aid in the destruction of the tumor and microvasculature by starving the diseased tissue of additional nutrients and oxygen, which can result in enhancement of tumor tissue hypoxia.57
C.3 Importance of light dosimetry
The most frequently used PDT light sources are lasers. They produce highly coherent monochromatic light that can be focused, passed down through an optical fiber and directly delivered to the target site through specially designed fiber optics. Early on, most laser systems used (such as the Argon-pumped dye laser) were bulky and expensive. These lasers were only fit for laboratory research purposes, not for clinical environment. Recently, diode lasers are now well developed; they are portable, lightweight, less expensive, thus making PDT more economical and practical.
A critical parameter for consideration in discussing the efficacy of PDT is the depth of light penetration through tissue. The light penetration is dependant on the optical properties of the tissue including reflection, scattering, transmission and absorption. Therefore, pigment-rich tissues, such as those of melanoma, are resistant to PDT. Tissue penetration is also affected by the wavelength of the light. It was demonstrated that light with a wavelength near 800 nm passes most deeper in tissues.38 Both absorption and scattering of light by tissue increase as the wavelength decrease. Light with wavelength shorter than 580 nm is not suitable because of strong absorption by hemoglobin. Typically, the depth of penetration is from 3 to 8 mm for light in the range from 630 to 800 nm.
Light dosimetry is another important vector to determine the efficiency of PDT treatment. It includes two parameters: fluence and fluence rate. Fluence is the total energy of exposed light across a sectional area of irradiated spot (energy per unit area of exposed light, J/m2). Fluence rate is the radiant energy incident per second across a sectional area of irradiated spot (power per unit area of light, W/m2, 1 W = 1 J/s). As the fluence rate lowers, the exposure time gets longer to provide the same fluence (dose) of light. Clinically, high fluence rates are usually chosen to reduce treatment time. However it was reported that high fluence rate may not be appropriate for eliciting an optimal effect. It has been reported by Wilson et al.58 that when fluence rates are high, the depletion of oxygen can occur to such an extent that it results in a reduced photodynamic effect since there is not an effective production of the cytotoxic species. Studies are underway in several laboratories to develop oxygen diffusion models in which lower fluence rates generate singlet oxygen more efficiently so that more singlet oxygen is exposed to the tumor over longer periods of time. The degree of damage to healthy tissue is also reduced, possibly, because the healthy tissue can repair minor damage more efficiently, avoiding a greater inflammatory response.
C.4 Photosensitizers
Photosensitizers (PS) are the key element in PDT. After the approval of Photofrin® for the PDT treatment, researchers from all over the world got actively involved in developing efficient PS. An ideal PS should fulfill the following requirements.
(1) It should be able to produce singlet oxygen efficiently because singlet oxygen is believed to be the key cytotoxic agent responsible for the destruction of tumors by PDT.
(2) It should have a high absorption coefficient in the long wavelength region (700 nm to 800 nm). Porphyrin-based photosensitizers have a strong absorption band around 400 nm called the “Soret” band, and a satellite absorption band (Q-band) between 600 and 800 nm. Only the Q-band is useful for PDT treatment. Porphyrins, chlorins and bacteriochlorins are aromatic molecules having 22-, 20-, and 18-π electrons, respectively. Besides exhibiting a strong Soret band, the long-wavelength absorption of porphyrins is usually in the region of 600–650 nm. Chlorins' Q-bands are around 630 nm to 700 nm The bacteriochlorins' long-wavelength absorption falls between 700 nm and 800 nm depending on the nature of substituents present at the peripheral positions, photosensitizers at this wavelength region are capable of treating large and deeply seated tumors.
(3) It should have no dark toxicity, minimal skin photosensitivity and should selectively accumulate in tumor tissue (preferential tumor retention, fast tumor accumulation and rapid clearance from other organs).
(4) The distribution of a PS is important in PDT processes and is influenced by its chemical structure. It is particularly useful if a PS is amphiphilic water-soluble but also containing a hydrophobic matrix.
(5) It should be stable and easy to dissolve in injectable solvents (formulation).
(6) It should be chemically pure and can be prepared via a short synthetic route involving simple technique(s) with high yield.
C.5 Basic Structures of tetrapyrrole-based photosensitizers and the IUPAC approved numbering for nomenclature
Porphyrins are 18π-electron aromatic macrocycles that exhibit characteristic optical spectra with a strong π–π* transition aroud 400 nm (Soret band) and usually four Q bands in the visible region. Two of the peripheral double bonds in opposite pyrrolic rings are cross-conjugated and are not required to maintain aromaticity. Thus reduction of one or both these cross-conjugated bonds maintains much of the aromaticity (chlorin and bacteriochlorin, respectively). A change in symmetry results in red shifted Q-bands (640 to 800 nm) with high extinction coefficient. Phthalocyanines are a class of tetrapyrroles in which the C- at meso-positions (5-, 10- 15 and 20) is replaced by N. The longest wavelength absorption in this class of compounds falls near 700 nm.
D. Light penetration depth of photosensitizers
As discussed above, the diffusion range of 1O2 is predicted to be limited to approximately 45 nm in cellular media.58–60 So the tissue-penetration distance of light is an important consideration for PDT. It is well established that both absorption and scattering of light by tissue increase as the wavelength decreases.38 The tissue absorption components include nucleic acids, amino acids, hemoglobin, melaninetc. For nucleic acids and amino acids, their absorption wavelengths are usually from 250 to 300 nm, so that they have little effect on the absorption of longer wavelength of light over 600 nm. The intrinsic absorption of most tissue is dominated by hemoglobin. Hemoglobin in the oxygenated state is referred to as oxyhemoglobin (HbO2); in the reduced state, it is called deoxyhemoglobin (Hb). The strong absorption peaks of hemoglobins are found at wavelengths shorter than 620 nm. The tails of these bands grow weaker with increasing wavelength from 620 to 800 nm. Another endogenous chromophore is melanin, which is a polymer60 built by condensation of tyrosine molecules and has a broad absorption spectrum exhibiting stronger absorption at shorter wavelengths. All these factors account for the fact that light penetration is very low at the wavelengths <550 nm. However, as shown in Fig. 5, it almost doubles from 550 nm to 630 nm38 (where Photofrin® is activated) and doubles again in going to 700 nm. This is followed by a 10% increase in tissue penetration as the wavelength moves towards 800 nm. The optical window for PDT studies is in the range of 600 nm to 900 nm.Wavelength beyond 900 nm is not desirable.
![Relative diffusion depth of penetration with light at various wavelengths (assuming the depth of tissue penetration at 800 nm is 100%) [The Porphyrin Hand].](/image/article/2011/CS/b915149b/b915149b-f5.gif) |
| Fig. 5 Relative diffusion depth of penetration with light at various wavelengths (assuming the depth of tissue penetration at 800 nm is 100%) [The Porphyrin Hand]. | |
E. Clinical status of selected photosensitizers
Two different approaches- (i) active and (ii) passive drug drug development approaches have been followed for designing photosensitizers in various laboratories. Among the two approaches, the passive approach, mainly based on a structure–activity relationship (SAR), has been more successful. The active approach, which basically deals with targeting the PS to certain receptors known for a high experssion in tumor cells showed promising efficacy in vitro, but have produced limited in vivo activity and tumor-selectivity.
In general, amphiphilicity (the presence of hydrophilic and hydrophobic groups at various positions of the molecule that intereact with the solvent independently), which can be correlated to partition coefficient values, seems to play an important role in tumor uptake of the photosensitizes. It can also affect the aggregation characteristics of the highly conjugated tetrapyrrolic systems, which in turn could alter the photophysical properties and intracellular site(s) of localization of the molecules. Aggregation shortens the triplet-state lifetime and decreases the singlet-oxygen quantum yield by dissipating the energy through internal conversion. Therefore, highly aggregated photosensitizers in vivo are considered to be less effective. However, the aggregation characteristics of the photosensitizer in vitro could be different than the in vivo environment, and, not much is known about the behavior of these agents in vivo.61
A brief description on selected photosensitizers approved by various health organizations or are at various stages of human clinical trials are as follows:
E.1 Photofrin
An effort led by Dougherty to prepare purified version HpD in large quantities following the US FDA regulations produced the first approved photosensitizing drug, Photofrin® 1, for clinical use. Photofrin442,62,63 is a mixture enriched in the more active dimeric and oligomeric components. Photofrin has been approved for clinical use in the treatment of early- and late-stage lung cancers, esophageal cancer, bladder cancer, malignant and nonmalignant skin diseases and early-stage cervical cancer. It is also being considered as a potential therapy against Kaposi's sarcoma, Barrett's esophagus with high-grade dysplasia, psoriasis and cancers of the head, brain, neck and breast. For early-stage lung cancer, Photofrin-mediated PDT is a relatively recent addition to the already well-established treatment methods of surgical resection, radiotherapy and chemotherapy.64 Successful application of Photofrin-based PDT has been achieved in patients with nonsmall cell lung cancer (NSCLC), each of which were considered suitable surgical candidates prior to PDT treatment. Of one group of 13 patients, 10 patients demonstrated complete response following the initial PDT treatment. The remaining patients required a second treatment for a complete response to be obtained. In a pilot study using Photofrin-mediated PDT in patients with inoperable NSCLC, 10 of 11 stage 1 NSCLC patients achieved complete remission. The remaining patient and 11 out of 15 stage III NSCLC patients showed only a partial response to treatment.
Temoporfin
652 (meso-tetra-hydroxyphenyl-chlorin, mTHPC, Foscan®; biolitex AG, Jena, Germany) is a tissue-based photosensitizer that is activated 2–5 days after intravenous administration. It accumulates in the skin, and requires protection of the eyes and skin from sunlight for up to 6 weeks. Temoporfin was the first sensitizer to be used in a formal clinical study of PDT for prostate cancer, which was conducted in 2002. The study was performed by Nathan and colleagues65,66 at University College London, London, UK. The authors assessed the effects of PDT using Temoporfin in the postradiotherapy setting. The first five patients in the study received low light doses (20 J/cm2), and small areas of devascularization were seen on postprocedure CT. Four of these patients chose to undergo re-treatment at a higher light dose, and an additional nine patients were then given Temoporfin and a light dose of 50 J/cm2. The study design allowed the treatment of selected areas of the prostate on the basis of results from biopsy and pretreatment imaging. The selected treatment area could have been the whole of the peripheral zone from base to apex; whole-gland treatment was not performed, in order to avoid adverse effects. Post-treatment imaging showed areas of necrosis, which were often patchy. Volumes of necrosis varied from 0.9 cm3 to 13.4 cm3, with up to 91% necrosis of the prostate for a bilateral treatment (nine treatments were bilateral). In 2006, this same group carried out a pilot study of PDT in the primary setting.67 Six patients were included, and a total of 10 PDT treatments were administered; patients received a second treatment if residual cancer was detected on post-treatment biopsy. The two patients who received only one treatment also showed residual cancer. However, one patient chose to continue on an active surveillance program, and the other chose to undergo radiotherapy. Treatment was aimed at areas of cancer (identified on biopsy or imaging) and the adjacent peripheral lobe. Only one lobe of the prostate was treated during each session. A combination of bare-tip and cylindrical light diffusers were used, with total light doses of up to 1800 J per lobe. The volumes of necrosis, as measured by post-treatment MRI, varied from 1.2 cm3 to 51.1 cm3. Adverse effects were minimal in this group. One patient required intravenous antibiotics for Gram-negative sepsis 24 h after light delivery. Only one of the patients reported deterioration in erectile function, which he attributed to worsening angina and performance anxiety. His erectile dysfunction resolved spontaneously after 5 months. These two studies demonstrated the potential efficacy of temoporfin in the treatment of prostate cancer; however, owing to the financial collapse of the company that produced temoporfin, and the subsequent takeover of the drug's manufacture by a company who did not wish to continue prostate PDT research, further work with temoporfin in prostate cancer was not carried out. m-THPC was also reported for the treatment of breast cancer,68 head and neck cancer,69 and pancreatic cancer.70
Several types of skin conditions are among the first to be studied due to their accessibility to photosensitizer and external light. The RPCI group pioneered skin cancer PDT in the 1970s using HpD and red light from a xenon arc lamp in patients who suffered primary or secondary skin cancers. This early study demonstrated that the primary skin cancers which showed a complete response (CR) included squamous cell carcinomas (SCCs, 20%), basal cell carcinomas (BCCs, 70–80%) and malignant melanomas (50%), and the secondary cancers originated from primary breast cancer, colon cancer, and endometrium cancer (80%).71,72 Interstitial PDT might be an option for subcutaneous and cutaneous tumors of a larger volume.73 Since the discovery of endogenous protoporphyrin IX (PpIX) 3 photosensitization induced by exogenous administration of ALA74 skin premalignant and malignant lesions also become a favorite target of ALA-PDT with the exception of the pigmented malignant melanomas due to limited light penetration. Studies demonstrated ALA-PDT to be effective, safe, and well tolerated by patients, and led to the US FDA approval in 2000 which marked another historic event for PDT. ALA-PDT was also approved for moderate inflammatory acne vulgaris in US in 2003. A number of other nonmalignant conditions (e.g., psoriasis, viral warts, and hair removal) are currently under clinical investigation worldwide.75–78 Clinical investigational studies of ALA-PDT have also extended to BCCs, basaloid follicular hamartomas, SCCin situ (Bowen's disease), cutaneous T-cell lymphoma, and sebaceous gland hyperplasia in recent years.79–88 Zaak and colleagues89 have assessed the use of ALA in patients with prostate cancer. The authors initially investigated whether ALA would be taken up by the cancer cells: ALA was given orally, at a dose of 20 mg/kg, 4 h before patients underwent radical retropubic prostatectomy; prostatectomy specimens were then analyzed by fluorescence microscopy. ALA was detected in cancer cells, but not the surrounding epithelial cells or stroma. This study is interesting because of the selectivity of the photosensitizer for prostate cancer cellsversus benign epithelial cells. The investigators then administered interstitial PDT to six patients using 633 nm light (250 J/cm via a 1 cm diffusing fiber) to activate the ALA. The results were quite encouraging. Besides ALA corresponding ester analogs are also being used for the treatment of treating a variety of superficial tumors.
E.4
Motexafin lutetium
Motexafin lutetium
4 (MLu, LuTex; Lutrin, a member of the family of texaphyrin molecules), which is predominantly a vascular-acting photosensitizer, has undergone testing in a canine model90 and has also been used in the postradiotherapy setting in human patients of prostate cancer. In a phase I trial, PDT using this photosensitizer was performed in 17 patients, 8 of whom had previously undergone external beam radiotherapy and 9 of whom had undergone brachytherapy.91–95 This study used a dose-escalation design; therefore, a wide variation in drug dose, light dose and drug–light interval was used. High-dose PDT was defined as that using the highest drug dose (2 mg/kg), the highest light dose (150 J/cm2), and the shortest drug-light interval (3 h); this group included 8 patients. Those patients undergoing high-dose PDT experienced the greatest initial rise in PSA level, followed by a reduction in PSA levels to below baseline values. By contrast, patients who received low-dose PDT (drug dose 0.5 mg/kg, light dose 25 J/cm2, and drug-light interval 24 h) had a treatment-related rise in PSA level that did not subsequently fall below baseline. So that it seems that high-dose PDT by LuTex is more promising than low-dose PDT. However, the authors did not report any post-treatment imaging. Changes in PSA levels are difficult to interpret in such a small number of patients. Motexafin lutetium was also reported for successful treatment of breast cancer96 and ophthalmic disease.97
E.5 Padoporfin and padeliporfin
Padoporfin 598–100(WST-09, Tookad®) and padeliporfin 6 (WST-11, Stakel®) are palladium bacteriopheophorbide photosensitizers. Padoporfin is a lipophillic photosensitizer, and so needs a carrier (e.g. cremaphor) in order to be given as an intravenous infusion; padeliporfin is water-based, and does not need a carrier to be given intravenously. Padoporfin was the first of this family of photosensitizers to be used in prostate clinical studies, and padeliporfin, which was developed later, is now being investigated for the same indication. Both are vascular-acting photosensitizers. Padoporfin is administered by a 20 min continuous venous infusion. Delivery of the activating light begins within a few minutes of starting the infusion. Extensive skin testing has shown that no skin phototoxicity occurs 3 h after the procedure, and that patients could be treated in the ambulatory care setting.101 The first padoporfin trial included 28 Canadian patients who had recurrent prostate cancer following radiation therapy. A drug dose of 2 mg/kg was shown to be most effective. Response was assessed one week after treatment by dynamic gadolinium-enhanced MRI, and by biopsy and further MRI at 6 months post-treatment. In patients who received at least the threshold light dose, 60% were complete responders—that is, the one-week MRI showed extensive avascular areas, and the 6-month biopsy did not show any evidence of residual cancer. Two patients developed rectourethral fistulae, one of which healed spontaneously, and the other one was still causing intermittent problems at 6 months post-PDT. Other irregular and extraprostatic treatment effect was also reported.
Initial work with padoporfin has been carried out in Europe in men who have not received any other treatment for prostate cancer, and who are suitable candidates for active surveillance. Preliminary results from this study have shown that it is possible to create ablative lesions within the prostate that show good conformation to the prostate boundaries, and that vary dependent on light dose.102 This group has gone on to use padeliporfin in their next study with men with previously untreated prostate cancer. Studies have been also published of its use in choroidal vessel occlusion in rabbits and rats, and in a melanoma tumor model.103
E.6
Npe6
Npe67b (LS11, mono-L-aspartyl chlorine, talaporfin sodium and laserphyrin) is a chemically pure second generation photosensitizer which has been approved in Japan for treatment of early centrally located lung cancer. It is a hydrophilic chlorin, mainly due to the presence of an aspartyl residue, which is derived from Chl-a.104,105 Phase I clinical trials assessing the safety and tolerability of NPe6 in recurrent subcutaneous tumors have also been completed. In all cases, tumor necrosis was observed followed by regression of tumor 24–48 h post-treatment, eventually leading to formation of an eschar over the site of the tumor. A Phase II trial investigating the effect of NPe6-PDT in patients with early superficial SCC of the lung has also been performed. A complete response rate of 84.6% was attained with the 39 lesions treated and, on a per patient basis; a complete response rate of 82.9% was achieved. Minimal skin photosensitivity and pulmonary toxicity were also observed.
There was quite-a-bit of controversey over the structure of the title compounds, which was finally assigned as 7b instead of 7a reported previously.106
E.6
Radachlorin
Radachlorin® (RADA-PHARMA Ltd., Russia) is the registered trade mark of a chlorophyll-a derivative. This drug substance represents an aqueous solution of three chlorins, including sodium chlorin e6 (90–95%), sodium chlorin p6 (5–7%), and a third chlorin with unknown structure (1–5%). The drug as a mixture has been reported to be stable in aqueous solution by the authors.107Radachlorin is in clinical trials for several cancer indications in Russia.
E.7 Purlytin
Purlytin 10 (Tin ethyl etiopurpurin, also called)108 is a second generation photosensitizer which has shown significant potential in Phase I/II studies in the treatment of metastatic breast adenocarcinoma, basal cell carcinoma (BCC) and Kaposi's sarcoma in patients with acquired immunodeficiency syndrome.109 The application of SnET2 in the treatment of prostate cancer in a canine model has been assessed. Significant amounts of SnET2 were found to be retained within the prostate up to 168 h postadministration. The greatest volume reduction of prostate tumor (60%) was observed using multiple diffusers for transperineal interstitial light delivery compared with transurethral and transperineal illumination (52%). Studies have assessed the efficacy of tin ethyl etiopurpurin in a single patient suffering from BCC with 13 lesions in total. A complete response was observed in the patient treated with Purlytin-PDT, with the effect maintained up to 6 months post-treatment.
Phthalocyanines (Pc) are a group of second generation photosensitizers which are structurally related to porphyrins. The first known appearance of the blue colored pigment was observed by Braun and Tcherniac in 1907; however, it was not until 1933 that the structure of Pc was elucidated. There are numerous commercial uses associated with Pc in the textile, photography and electrical industries, which have been broadly published over the years; however, Pc have attracted attention over the last number of decades in the area of PDT due to their optimal photophysical and photochemical properties. Pc exhibit strong absorption bands at 670–770 nm in the red region of the spectrum and the presence of an appropriate central atom, such as zinc, aluminium or silicon, yields high singlet oxygen production with long-lived triplet states. Thus far, studies using the silicon phthalocyanine photosensitizer Pc4 both in vitro and in vivo are promising and certainly warrant further assessment. Clinical evaluation of Pc4 has been assessed in cutaneous and subcutaneous lesions from diverse solid tumor origins.110,111
A Phase I clinical trial of topically applied Pc4 11 for treatment of mycosis fungoides, the most common form of cutaneous T-cell lymphoma is currently ongoing.
E.9 Verteporfin
Verteporfin 12 (BPD-MA, benzoporphyrin derivative monoacid ring A) was synthesized in the mid-1980s with an intention of cancer treatment. However, it has been used primarily for ocular PDT and approved for age-related macular degeneration (AMD) worldwide since 2000. Verteporfin in photodynamic therapy involves intravenous administration of Visudyne® (verteporfin) followed by activation through an ophthalmoscope equipped with a 690 nm diode laser while the photosensitizer is still in the general circulation. Several well designed clinical studies in North America and Europe showed that AMD patients treated with verteporfin-PDT were more likely to experience stabilized vision than those given a placebo.112 Verteporfin therapy should be considered as a first-line therapy in these difficult-to-manage conditions such as subfoveal choroidal neovascularisation secondary to AMD, pathological myopia or presumed ocular histoplasmosis syndrome.
E.10 Photosensitizer derived from pyropheo- phorbide-a (alkyl ether analogs)
The RPCI group was the first to use the concept of structure–activity relationship (SAR) and quantitative structure–activity relationship (QSAR) in developing effective PDT agents. An initial study was performed in the pyropheophorbide-a series. In brief, Pandey et al.113 synthesized and evaluated a series of the alkyl ether derivatives of pyropheophorbide 13 (derived from chlorophyll-a) with variable lipophilicity. The study indicated that overall lipophilicity of the molecule played an important role in biological efficacy. The results from the in vivo quantitative structure–activity relationship (QSAR) study114 displayed a parabolic relationship in that there was a minimal PDT response with analogs of Log P ≤ 5, with activity peaking between Log P of 5.5–6.0 and then eventually decreasing at Log P ≥ 6. In summary, the in vivo photosensitizing efficacy was optimal for the hexyl ether analog (C6). Currently, the hexyl ether analog 14, HPPH (λmax = 665 nm), is progressing through Phase I/II human clinical trials for BCC, obstructive esophageal, head and neck cancers and Barrett's esophagus with high grade dysplasia.115 The early clinical results are promising (see the section Clinical PDT). F.11a. HPPH or Photochlor.
HPPH 14 derived from chlorophyll-a is an excellent PDT agent with limited skin phototoxicity.116,117 It is currently in advanced clinical trials for various indications: lung, esophagus, including Barrett's and head and neck cancer. The clinical results are excellent. In contrast to to Photofrin or Foscan, none of the patients treated with HPPH showed any significant long-term skin phototoxicity. The animal and human clinical data of HPPH-PDT,118–129 shows its enormous potential in treating certain types of cancers and are briefly summarized:
E.10a Treatment of squamous cell carcinoma.
In general, PDT has been shown to be effective treatment modality for surface-oriented neoplasms of the skin, esophageal, gastro- intestinal, and urogenital systems. Magne et al.126 at U.C. Davis investigated the use of HPPH 14 in the treatment of squamous cell carcinomas of the feline facial skin. Tumors were exposed to the laser light (665 nm) at 24 h post injection of HPPH. Following treatment, tumors were evaluated for complete response rates and local control duration. Complete response rates as well as local control durations were significantly (p, 0.05) related to stage (Fig. 6).
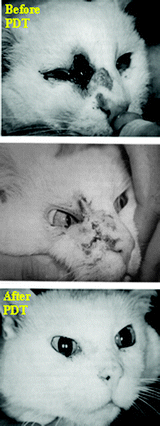 |
| Fig. 6 Squamous carcinoma treated with HPPH-PDT (Cat). | |
For example complete response was achieved in 100% of the T1 tumors, 56% of T1b tumors and 18% of T2b tumors. One year control rate was 100% for T1a tumor, 53% for T1b tumors. Clinical, hematological and biochemical evidence of toxicity was not seen in any cat following drug administration. HPPH was also investigated in horses bearing squamous carcinoma in eyelids. In this case, HPPH 14 was formulated in DMSO and used topically. Light treatment (665 nm) produced a complete response (Fig. 7). Please note that both type of animals were previously treated with radiation and surgery, but showed significant tumor-recurrence.
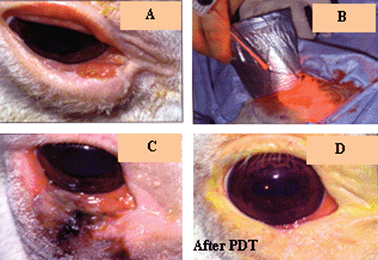 |
| Fig. 7 Squamous carcinoma treated with HPPH-PDT (Horse) (Lasers in surgery and Medicine 2006, 5, 445). | |
E.10b Treatment of canine tumor.
Another significant outcome of HPPH-PDT was also observed in a canine tumor. HPPH 14 at a dose of 0.3 mg/kg and 24 h postinjection (light dose: 135J/cm2, 75 mW/cm2) produced 100% tumor cure. (Fig. 8).
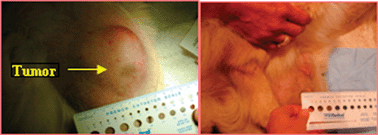 |
| Fig. 8 Treatment of canine tumor by HPPH-PDT. The tumors were treated with light at 24 h postinjection. | |
E.10c
HPPH for treating human cancers (skin, lung, high grade dysplasis in Barrett's esophagus, obstructing esophagus and oral cancers (head and neck).
Central airway obstruction is a common complication of lung cancer, and local failure with endobronchial recurrence is frequently seen in patients who have been previously treated with radiotherapy or chemotherapy. While PDT with FDA approved drug Photofrin is a highly effective treatment modality, the persistence of Photofrin in skin necessitates complete protection from sunlight and other sources of bright light for periods typically 30 to 60 days. However, certain 2nd and 3rd generation photosensitizers including HPPH show minimal skin phototoxicity.120
HPPH
14 has been entered into PhaseI/II trials at Roswell Park Cancer Institute for early and late stage lung cancer. INDs were also obtained for treatment of partially obstructing esophageal carcinoma, high grade dysplasia in Barrett's esophagus, and basal cell carcinoma of the skin. Pharmacokinetics studies of 25 patients revealed estimated half-lives of 7.77 h (3.46–17.6 h) and 596 h (120–2951 h), respectively. No metabolites were detected in serum. An extensive study of skin phototoxicity in 48 patients with HPPH receiving variable drug doses and solar simulator light doses has demonstrated that even one day after drug administration the highest drug and light doses elicited a response of only erythema without edema. Even these mild responses declined over a few days.
A total of 16 lung cancer patients have been treated with HPPH-PDT. All patients except one have had complete or partial response and skin phototoxicity has been negligible (Fig. 9).
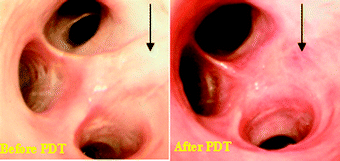 |
| Fig. 9 Lung tumors (human) treated with HPPH-PDT. | |
Among esophageal cancer patients including Barretts' esophagus or obstructing esophageal carcinoma, the HPPH-PDT response was excellent (Fig. 10).
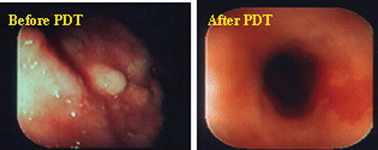 |
| Fig. 10 Esophageal tumor (human) treated with HPPH-PDT (Lasers in Surgery and Medicine, 2006, 4, 445). | |
Another indication where Photofrin-PDT has shown excellent outcome is in head & neck cancer patients, especially in oral cancer. A number of patients with oral cancers are not suitable for most of the current treatment modalities (surgery, chemotherapy or radiation), but Photofrin-PDT has elicited excellent results (Fig. 11).
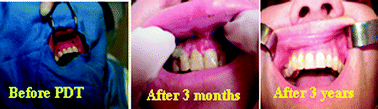 |
| Fig. 11 Oral cancer treated by photodynamic therapy. | |
At Roswell Park Cancer Institute the treatment of oral-cancer with HPPH-PDT (Phase I/II) is underway and the results are as good as reported by using Photofrin as a photosensitizer, but without any significant skin phototoxicity.
F. Photosensitizers (700–800 nm) under advanced preclinical studies
This part mainly focuses on the description of certain effective long-wavelength photosensitizers38,130 derived from methyl pyropheophorbide-a 15purpurin-18 methyl ester 16, methyl bacteriopheophorbide-a 17, methyl bacteriopurpurin 18 and developed at the PDT Center, Roswell Park Cancer Institute, Buffalo.
F.1 Photosensitizers derived from N-substituted purpurinimide (alkyl ether analogs)
As discussed above, the SAR approach followed in developing effective photosensitizer from a series of alkyl ether analogs of pyropheophorbide-a was extremely useful in selecting the highly effective photosensitizer HPPH. We therefore extended this approach to the purpurinimide system and synthesized a series of the corresponding alkyl ether analogs with variable carbon units (Log P 5.32–16.44) at two different positions (3-(11-O-alkyl) and 132-N-alkyl) with long-wavelength absorption near 700 nm (εmax = 45
000 in CH2Cl2) and efficient 1O2 generation (57–60%).131 Interestingly in this series, in contrast to the alkyl ether of pyropheophorbide-a, which showed a parabolic relationship between the log P value and PDT efficacy, the purpurinimide analogs demonstrated a linear relationship. These data suggest that overall lipophilicity (log P) could be an important property in selecting the best candidate in a particular series, but the use of these values in designing photosensitizers with another system may not be beneficial. We extended this approach in synthesizing certain fluorinated analogs in which the alkyl ether side chain was replaced with trifluoromethyl benzyl ether groups. Among these photosensitizers, compared to non fluorinated analogs, the corresponding fluorinated derivatives showed improved PDT efficacy.132 However, in the purpurinimide series, a photosensitizer 19 containing the N-butyl and O-butyl side chains is currently being evaluated for toxicological studies and was selected on the basis of its in vivo PDT efficacy and ease of synthesis.
F.2 Photosensitizers derived from N-Substituted bacteriourpurinimide (alkyl ether analogs)
Bacteriochlorins are a class of porphyrins in which two pyrrole rings diagonal to each other are reduced. Such systems exhibit long-wavelength absorption in the range of 720–800 nm depending upon the nature of substituents and the fused aromatic ring present at the peripheral positions. Pandey and coworkers133,134 converted the bacteriochlorophyll-a (isolated from Rb. sphaeroides) to a series of N-substituted bacteriopurpurin imides 20 in a sequence of reactions. The acetyl-group present at position-3 was then replaced with a variety of substituents. Among the compounds investigated so far the methyl 3-(1′-butyloxyethyl)-3-deacetyl-bacteriopurpurin-18-N-butylimide was found to be most effective in various tumor models at 24 h post treatment. The toxicological studies of this compound are currently in progress. Interestingly, in this series similar to the purpurinimide system, a direct relationship between PDT efficacy and overall lipophilicity was observed. This could be due to similar structural features between the two systems.
F.3 Photophysical characteristics of highly effective photosensitizers selected from pyropheo phorbide-a, purpurinimides and bacteriopur purinimides
Photosensitizers derived from chlorophyll-a and bacteriochlorophyll-a produce their long-wavelength absorptions bacteriochlorins developed at RPCI, Buffalo in the range of 660 to 800 nm. For example, the hexyl ether derivative of pyropheophorbide-a (HPPH 14) shows the long-wavelength absorption at 660 nm (in vitro). Replacing the five member iso-cyclic ring with a fused a six member imide ring system gives a significant red-shift (40 nm) and shows a strong peak at 700 nm. Interestingly, a similar system in which instead of only ring-D, both ring-B and ring-D are reduced (diagonal to each other) a further red-shift in the electronic absorption spectrum was observed, exhibiting the long-wavelength absorption near 800 nm. This example illustrates a very simple and well designed SAR approach for the development of highly effective long wavelength photosensitizers.135 See Fig. 12.
Unlike naturally occurring bacteriochlorophylls, the modified analogs are quite stable and show high singlet oxygen producing efficiency in the range of 45–50%.
F.4 Structural modifications of pyropheo phorbide chlorin e6, chlorinp6 and purpurinimide systems
During the last 15 years several new classes of porphyrin based photosensitizers related to benzochlorin, benzoporphyrin derivatives and bacteriochlorins have been evaluated for PDT efficacy. Some of these compounds were found to be quite effective in both in vitro and in vivo models. Here a brief summary on the synthesis of such analogs mainly derived from methyl pyropheophorbide-a (a chlorophyll-a analog) is presented.
F.4a Benzochlorins.
The reduced ring-D in methyl pyrophrophorbide-a was converted into the corresponding Ni(II) derivative, which on hydrogenation produced Ni(II) mesopyropheophorbide-a 21. This product on subsequent Vilsmeier formylation with dimethyl aminoacrolein and phosphorous oxychloride gave the 20-(2-formylvinyl) derivative 21a. Reaction of this intermediate under acidic conditions and subsequent treatment with DDQ yielded the desired benzochlorin derivative 22.136 In both in vitro and animal models this compound showed photosensitizing activity, but compared to HPPH 14 it was less active.
F.4b
Benzoporphyrin and benzobacteriochlorin derivatives.
Benzoporphyrin was initially prepared by Johnson et al.137 on reacting propotoporphyrin IX dimethyl ester with dimethylacetylenedicarboxylate under Diels Alder reaction conditions. By following a similar methodology, a series of pyropheophorbide-a analogs related to 24 were synthesized by Pandey and Smith et al.138
Pandey et al.139 also extended this approach for the construction of bacteriochlorin 26 from 8-vinyl mesopyro pheophorbide-a 25, which was obtained in a sequence of reactions from methyl pheophorbide-a 23.
F.4c Ketobacteriochlorins.
Porphyins on reacting with osmium tetroxide produce diols or triols, which on following Pinacol–Pinacolone reaction conditions generate the corresponding ketochlorins or bacteriochlorins. Pandey et al.140 used this approach in pyropheophorbide-a 15 and other chlorin systems resulting into the corresponding ketobacteriochlorins 27 and 28. The nature and position of the substituents present at the peripheral position(s) exhibited a remarkable difference in long wavelength absorption of the resulting chromophores.
F.4d Regioselective synthesis of ring-Bvs. ring-D chlorins.
Oxidation of chlorins, bacteriochlorins and their stability under in vitro and in vivo conditions has been a subject of discussion for a long time. Several oxidizing agents have been used to oxidize the pyrrole-ring reduced system. To understand the biological efficacy of ring-Bvs.ring-D reduced chlorophyll-a analogs in PDT efficacy, Pandey and coworkers141 have reported a highly effective FeCl3 mediated oxidation of a certain bacteriochlorin analog to produce ring-B reduced chlorins. Interestingly, both ring-B and ring-D reduced chlorins (29 and 30, respectively) derived by FeCl3 and DDQ mediated oxidation showed mitochondrial localization and similar in vivo PDT efficacy (BALB/c mice bearing Colon26 tumors).
F.4e Target-specific photosensitizers.
Since the approval of Photofrin®, efforts are underway in various laboratories to develop target-specific photosensitizers, but with limited success. Attempts have been made to target photosensitizers to known cellular targets by creating photosensitizer conjugates, where the non-porphyrin molecule is a ligand that is specific for targeting the tumor (e.g.cholesterol, certain chemotherapeutic drugs, DNA intercalatorsetc.).38Conjugation of certain peptides and monoclonal antibody conjugates showed promising activity in vitro with limited in vivo target-specificity.142 However, cRGD peptide, a most common integrin binding motif having the sequence of Arg-Gly-Asp (RGD) and carbohydrate conjugates (31 and 32) have shown promising photosensitizing activity both in vitro and in vivo models. Starting from pyropheophorbide-a and N-substituted purpurinimides, Pandey et al. have prepared a series of photosensitizers in which the targeting groups (carbohydrate,143cRGD144 or folic acid144 moieties) were introduced at variable peripheral position(s) of the parent molecules. Interestingly, the position of the substitent(s) made a significant difference in target-specificity.
During the last 15 years, the RPCI group synthesized and evaluated a series of PDT and imaging agents following the both active and passive approaches of drug development and the observed findings can be summarized as follows:
(a) Compared to the active, the passive approach based on SAR/QSAR studies was found to be more useful in identifying the improved PDT and PDT/imaging agents.
(b) The overall lipophilicity established in one series of compounds for identifying the most effective candidate could not be translated to other series of compounds. For example, the alkyl ether analogs of pyropheophorbide-a showed a parabolic relationship between the PDT efficacy and partition coefficient values and the compounds with log P values in the range of 5.5–6.00 produced the best efficacy. In contrast, the alkyl ether analogs of purpurinimide and bacteriopurpurprinimide showed a linear relationship between the lipophilicity and PDT efficacy. The log P value of the most effective candidates were in the range of 10.5–12.5 (determined by the PALLAS program). The extremely hydrophobic compounds were difficult to purify. Therefore, in these series of compounds, the most effective candidate was selected not only on the basis of best efficacy, but also the ease of synthesis.
(c) Presence of central metal (e.g. In, Ga, Pd and Zn) showed a remarkable enhancement in singlet oxygen yields and PDT efficacy is certain photosensitizers.
(d) Aggregation is another factor which seems to play an important role in altering the photophysical properties of the photosensitizers and should be considered an important factor in designing effective PDT agents.
(e) Most of the effective photosensitizers in pyropheophorbide series (e.g. HPPH) showed mitochondrial localization. However, the effective photosensitizers related to purpurinimide and bacteriopurpurinimides showed both mitochondrial and lysosomal localization. Interestingly, some of the highly effective carbohydrate analogs were mainly localized lysosomes.
(f) Depending on the nature of photosensitizer(s), the mechanism of tumor-uptake (cell-uptake) of the photosensitizer was determined to be either via simple diffusion or endocytosis.
(g) Varying the lipophilicity makes a remarkable difference in pharmacokinetic and pharmacodynamic properties of the molecule and it could also play an important role in tumor uptake and biodistribution of the photosensitizer.
(h) Vascular shutdown seems to be an important factor for the destruction of tumor by PDT.
(i) In PDT, both apoptotic and necrotic mechanisms play important role in cell death.
(j) In pyrppheophorbide-a series, the HPPH was found to be a substrate for ABCG-2 (ATP–binding Cassette, Sub-Family G, Member 2) In contrast, the corresponding carbohydrate analogs (glucose and galactose derivatives) as well as the In(III)-HPPH were not the substrate for ABCG-2, and thus do not pump out quickly from tumor cells. This ould be one of the reasons for their higher efficacy than HPPH, which pumps out quickly.
(k) Recent results suggest that STAT-3 (Signal Transducer and Activator of Transcription-3) dimerization could be a useful biomarker to monitor the PDT response in vivo.
(l) Naturally occurring Chlorophyll-a and bacterio chlorophyll-a moities provide unique opportunities for developing effective agents with long wavelength absorption in the range of 660–800 nm.
(m) In general, photosensitizers containing the hydrophobic group(s) on one side of the molecule and hydrophilic substituent(s) on the opposite side of molecule were found to be more effective than those in which these substituents were introduced all over the molecule.
(n) Tumor-avid porphyrin-based compounds can be used as vehicles for delivering the desired imaging moiety to tumors. These “multifunctional” agents could be extremely useful for a “See and theTreat” approach.
G. Activatable photosensitizers
Another approach for designing improved photosensitizers with increased tumor-specificity is to take advantage of lesion-specific expression of enzymes such as proteases. Weissleder and Ntziachristos145 were the first to use this approach in developing multifunctional agents, which is currently being used in various laboratories not limited to PDT. Zheng et al.146 reported the utility of this approach in developing a photodynamic molecular beacon as an activator photosensitizer 33 (derived from methyl pyropheoporphide-a 15) based on protease-controlled singlet oxygen quenching and activation. In this report the authors describe the synthesis and characterization of an MMP7-triggered photodynamic molecular beacon using pyropheophorbide-a as the photosensitizer, another molecule as a dual fluorescence and singlet oxygen quencher, and a short peptide sequence as the MM7 cleavable linker, with the cleavage site between G and L aminoacids. However, no in vivo photosensitizing data of the conjugate are reported.
H. Utility of tumor-avid photosensitizer (HPPH) in developing “Multifunctional Agents” for tumor imaging and therapy (Theronostics)
The diagnosis of various diseases including cancer at its initial stages is of continued interest and quite challenging. In general, pathology provides the most widely used clinical method for elucidating chemical information from diseased tissues. Traditional techniques of histology can be used to probe the microscopic structural alternations of diseased tissue. Utilizing histological stains, many of the corresponding chemical alternations accompanying disease can be mapped on a microscopic scale.147,148 The main disadvantage of these techniques is that they can only be applied in vitro, necessitating the removal of tissue. It implies that, using histological techniques, only small areas of tissue, accessible either to biopsy forceps or needles, can be sampled. A more useful diagnostic technique would combine the advantages of pathological, radiological and nuclear (PET/SPECT) methods, allowing for real time imaging of tissue in vivo to extract diagnostically relevant structural and histological information.149 Currently several noninvasive standard clinical diagnostic modalities, e.g., magnetic resonance imaging (MRI),150 computed tomography (CT),151positron emission tomography (PET),152 X-ray, single photon emission computed tomography (SPECT)153 and ultrasound (US)154 that are used suffer with several major drawbacks resulting in data yielding high resolution anatomic (structural) images of soft tissue but little physiologic (functional) information. The ability to readily provide researchers and clinicians with both structural and functional information during a single examination would significantly advance the field of diagnostics. Considerable progress has been made in the in vitro and preclinical development of molecular imaging agents. Small molecule imaging agents that can detect specific proteins or nucleic acids within living human cells, including mutant oncoproteins, have been studied, and some progress has been made in preclinical models. Antibody therapeutics represents a class for where imaging is particularly promising. Cell surface receptors offer opportunities for antibody-mediated target imaging. At the clinical level, the value of molecular imaging has also become important. Target imaging can be extremely helpful in determining the outcome of a particular treatment. Currently, the development of compounds for tumor-imaging and therapy, so-called “multifunctional agents,” is of enormous interest because this provides an opportunity to use a single agent for tumor-imaging and therapy.
Porphyrin-based photosensitizing agents for PDT possess unique advantages due to their ability to be retained in tumors and to produce cytotoxic singlet oxygen upon exposure to an appropriate wavelength of light. In addition to therapy, the fluorescence characteristics of these porphyrinsin vivo have been exploited in very early studies by Policard and others that can be dated back to 1924 for the detection of various early-stage cancers of the lung and bladder, and cancers of other sites.155 Photofrin®, a hematoporphyrin-based photosensitizer, is selectively retained by tumor tissues compared to most normal tissues except for liver, spleen, and kidney. Consequently, the difference in concentration of Photofrin® in malignant tissue is the basis of fluorescence imaging. In an early diagnosis of microscopic lesions in pre-clinical and clinical studies, Mang et al.156 showed the utility of nontherapeutic Photofrin® doses that are 80–90% lower than those used therapeutically, for tumor fluorescence detection of metastatic diseases.
Unfortunately, Photofrin® and most of the tumor-avid long-wavelength photosensitizers (e.g.bacteriochlorins) have very small wavelength differences between their NIR absorption and emission bands (Stokes shifts). Such an inherent property limits application of these molecules for NIR tumor-imaging. The in vivo imaging is preferably performed in the NIR (700-900 nm) because of efficient optical tissue penetration and minimal auto-fluorescence in this spectral region. At NIR wavelengths, light penetrates several centimeters in tissue (however, light penetration also depends on tissue-type) so that the fluorophore can be imaged deep within the body.157 In recent years, a number of different NIR fluorophores (e.g. cyanine dyes) have been described.158 In spite of excellent photophysical properties, most of the cyanine dyes are not tumor-specific and are eliminated from tissue rapidly. Therefore, efforts are underway in various laboratories to develop tumor-specific optical imaging agents by conjugating these moieties with various target-specific agents.159–165 Targeted delivery of long-wavelength emitting fluorophores to tumors is a new and exciting method for cancer diagnosis, intra-operative tumor detection, and for investigations of drug delivery and tumor physiology. Targeting can be assessed in real time, with high sensitivity and resolution. Fluorescence imaging has been extended from microscopy to in vivo imaging of biological targets and disease.166Fluorescence imaging relies on the properties of the fluorochromes or fluorophores. These particular molecules absorb the energy carried by photons in a given band of wavelengths and give back a fraction of this energy by emitting new photons in a band of longer wavelengths. Optical contrast agents can provide planar and tomographic images at millimeter to centimeter depth, with millimeter to sub-millimeter resolution and very high sensitivity. For small animals, planar images are adequate, but optical tomographic reconstruction of fluorescence images is becoming feasible.165 Briefly, NIR optical tomography takes advantage of a “therapeutic window” between 700–900 nm in which tissues exhibit low absorbance, but high scattering capacity. As a result, light in this wavelength regime can scatter through several centimeters of tissues before being extinguished by absorption. The ability to detect diseased tissues with NIR light depends critically on the “optical contrast”, or the consistent differences between the absorption and scattering properties of normal and diseased tissue volumes. The potential clinical advantages of the fluorescence technique include high signal sensitivity, especially if point measurements are used, the suitability in examination of tissue surfaces (compared to the “volume” imaging of most radiological techniques), flexibility in the anatomical sites that can be interpreted with small diameter optical fiber probes, reduction in the use of random tissue biopsies, and ease of use by clinicians. Fluorescence imaging also offers the possibility of associating a given fluorophore with a particular molecule. Thus it is possible to label specifically and simultaneously different targets with different fluorophores that can be easily discriminated on the basis of their emission wavelengths.
By realizing the importance of porphyrin-based compounds in tumor-avidity and photosensitizing efficacy and the advantages of the photophysical properties of cyanine dyes, Pandey and co-workers conjugated a tumor-avid photosensitizer, e.g.HPPH, which is currently in Phase II multicenter clinical trials, to a cyanine dye.167,168 The resulting “bifunctional agent” 34 (Fig. 13 and 14) showed both PDT efficacy and tumor-imaging capabilities.
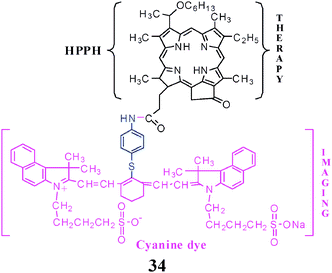 |
| Fig. 13
HPPH-Cyanine dye conjugate for tumor-imaging (fluorescence) and Therapy (PDT). | |
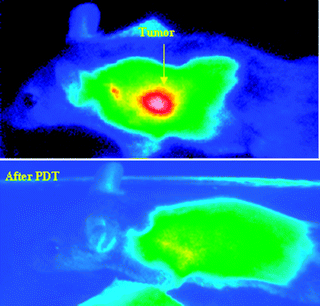 |
| Fig. 14 Whole body imaging of (A) BALB/c mouse bearing Colon 26 tumors (before PDT) at 24 h post injection of HPPH-CD conjugate 34 (dose: 0.03 μmol/kg). (B) Tumor image at 30 day post-treatment with the same conjugate shows complete tumor response. (Photochem. Photobiol. Sci. 2007, 6, 1257–1267). | |
At a therapeutic/imaging dose the conjugate did not show any significant skin phototoxicity. It was also found to be a promising imaging agent even at low doses. Results of whole body fluorescence imaging in RIF tumor-bearing C3H mice, with excitation of the fluorescent cyanine dye (780Ex and 865Emin vivo) 24 h post injection are shown in Fig. 13. As can been seen even at a dose of 0.03 μmol/kg, which was later found to be 8–10 fold less than the therapeutic dose, the conjugate produced significant tumor imaging capability. Under similar conditions, the unconjugated cyanine dye showed limited tumor-localizing ability. The bifunctional conjugate provides an opportunity to independently select and associate appropriate photosensitizers and fluorophores. For treating large and deeply seated tumors, photosensitizers exhibiting long-wavelengths absorption in the range of 780–800 nm and higher tumor-avidity than HPPH could generate more effective agents.
Planar imaging (epi- and trans-illumination) has gained wide popularity with significant advancements in the field of fluorescence molecular imaging. On the other hand, it suffers from significant drawbacks because fluorescence intensity has a strong non-linear dependence on lesion depth and on the optical properties of the lesion and the surrounding tissues.169,170 Therefore, having the same concentration of fluorochrome in tumors with differences in their vascularities and depth will yield different fluorescence intensities. Fluorescence tomography aims at three-dimensional reconstruction of the internal distribution of fluorochromes in tissues based on light measurements collected at the tissue boundary. Fluorescence tomography not only yields three-dimensional and “deep-tissue” imaging but also offers true quantification of optical contrast. A high resolution (512 × 512 CCD pixels) photographic image was obtained to get a structural map of each mouse imaged. This result clearly proved that tumor-avid HPPH-CD accumulated in tumor 24 h post injection. Liver uptake was also significant at this specific time point.171 Studies are underway to design improved agents with high tumor-avidity and reduced liver-specificity.
H.2 PS-MR contrast agents conjugates for MR imaging and PDT
Magnetic resonance (MR) imaging has become the method-of-choice for many procedures. Unlike nuclear imaging, conventional radiography, or computed tomography, MR often relies on “contrast-enhancing agents” to improve inherent contrast between normal and diseased tissue. MR signal intensity or signal intensity differences (contrast) are dependent upon longitudinal (1/T1) and transverse relaxation rates (1/T2) of tissue protons. Agents containing paramagnetic ions have been shown to effectively alter 1/T1 and/or 1/T2 by changing the local magnetic field when they come in close proximity to water protons. The paramagnetic ion most widely used in this regard is gadolinium [Gd(III)] because of its seven unpaired electrons and large paramagnetic moment.172 The Gd(III)-based contrast agents approved by the United States Food and Drug Administration (FDA) are Magnavist®, Omniscan®, and Prohance®, and all these are carboxylate-containing water-soluble complexes. Typical plasma clearance rates for FDA approved agents are on the order of 15 min or less, thereby limiting the usable time frame for MR data acquisition, which can take between 5–15 min/acquisition. The tumor selectivity of the current contrast enhancing agents is singularly dependent upon perturbations in normal vascular permeability; small foci of metastatic tumor cells, particularly those in minimally enlarged or normal sized lymph nodes, may not be detectable by current methods. However, by selective or nonselective binding of a MR contrast-enhancing agent to a tumor target or by simply slowing in vivo tumor clearance rates, several improvements can be made.
The use of tumor-avid porphyrins in delivering the desired MR imaging agent to tumor has been investigated by a independent studies of Hoffman et al.173 and Pandey and co-workers.174 Among these conjugates, gadolinium-based porphyrin contrast media, particularly gadoporphyrin-2 has been extensively studies and found to be necrosis-avid.175 Although such compounds may be useful for tumor imaging (5–30% mass is commonly necrotic), a MR agent that targets living tumor cells, particularly those that are actively undergoing metabolic processes, would be superior. As discussed previously, for quite some time Pandey and co-workers have been developing effective photosensitizers by following the SAR and QSAR approaches, which afforded a series of tumor-avid photosensitizers with long wavelength absorption in the range of 660 to 800 nm. Among these compounds HPPH 14 is currently undergoing Phase II clinical trials and shows limited skin phototoxicity. In order to develop a “bifunctional agent” for MR imaging and PDT, Pandey and co-workers initially conjugated HPPH to aminobenzyl-DTPAs with variable lipophilicity, which produced long-term PDT efficacy in mice with enhanced tumor contrast.175 For investigating the number of gadolinium units for MR imaging, HPPH 14 was conjugated with multiple magnetic resonance imaging (MRI) functional groups by following the methodology depicted in our laboratory.176 Among these analogs HPPH containing three gadolinium(III)aminobenzyldiethylene triamine pentaacetic acid [Gd(III)ADTPA] 35 (Fig. 15) produced the best tumor contrast and PDT efficacy. The in vivo studies performed in both mice and rat tumor models resulted in a significant MR signal enhancement of tumors relative to surrounding tissues at 24 h post-injection.
![MR imaging of HPPH-3 Gd(iii)ADTPA conjugate at 24 h postinjection (dose: 10.0 μmol/kg) [Bioconjugate. Chem. 2005, 16, 32–42].](/image/article/2011/CS/b915149b/b915149b-f15.gif) |
| Fig. 15 MR imaging of HPPH-3 Gd(III)ADTPA conjugate at 24 h postinjection (dose: 10.0 μmol/kg) [Bioconjugate. Chem. 2005, 16, 32–42]. | |
The water soluble (pH 7.4) HPPH-3Gd(III)amino benzylDTPA conjugate 35 also demonstrated great potential for in vivofluorescence imaging.176 An in vivo biodistribution study with the corresponding 14C-analog also showed a significant tumor-uptake at 24 h post-injection. Toxicological evaluations of the lead conjugate at therapeutic and higher imaging/therapeutic doses did not show any evidence of organ toxicity.177 The structures of the bifunctional conjugates with variable number of Gd(III)aminobenzyl DTPA are shown in Fig. 15. The dual agent showed the capability of tumor-imaging by MR at 10-fold lower dose than Magnavist, a clinical standard, and also produced significant photosensitizing activity.178 Currently, efforts by these authors are directed in developing tumor-targeted agents by introducing a variety of tumor-targeting moieties.
H.3 Radiolabeled PS for nuclear imaging (SPECT/PET) and PDT
With the advancement in “non-invasive” imaging technologies and imaging probes, the field of imaging science is growing exponentially and therefore, fusion of in vivo imaging techniques (CT, SPECT, PET, bioluminescence, fluorescence, MRI) to observe events at the molecular and cellular levels are expected to accelerate the drug discovery and development process.179 The imaging of specific molecular targets that are associated with cancer should allow for earlier diagnosis and better assessment of oncology patients. Whole-body positron emission tomography (PET) is a diagnostic modality that can noninvasively survey the entire body and sensitively detect various cancers. Positron emission tomography (PET) with 18F-fluorodeoxyglucose (FDG) has been developed to quantitatively assess local glucose metabolism.180 Because malignant tumors exhibit increased glucose metabolism, quantification of FDG uptake using PET helps to differentiate between benign and malignant tumours, to determine the degree of malignancy,181 to evaluate the effectiveness of chemotherapy or radiotherapy182 and to predict prognosis.183–185 Since the invention of the whole-body imaging technique,186 PET has also been used to depict hypermetabolic cancers. PET imaging has been shown to be sensitive enough to detect various cancers including lung, breast, colorectal, pancreatic, head and neck cancer, and malignant lymphoma and melanoma.180,187,188 It can also be used successfully in patients with an unknown primary tumor. Thus PET imaging has the potential to detect cancers of many types at early stages with a single study.
With recent advent of small animal micro-PET systems whose resolution could reach near 1.2 millimeters, PET has widened appeal for research at the drug development stage as it allows studying the drug distribution in vivo by using radiolabeled tracers, which are injected in non-therapeutic doses, 3-D images can be reconstructed using a computer to show the location(s) and concentration of the tracer, which then can be coupled with a therapeutic agent to treat the target. The approaches now focus on the development of a common molecule which is first used in low mass (as a radioactive material) to image and measure the target function, and then the mass of the molecule is increased by using the same unlabeled compound at therapeutic levels to treat the target function.189Drugs labeled with 11C (t1/2 = 20.4 min) and 18F (t1/2 = 110 min) are the most commonly used PET isotopes. The short half-life of 11C and 18F limits their use in studies involving monoclonal antibodies, and other molecules like photosensitizers (PS), which take a long time (hours instead of minutes) for accumulation in tumors. 124I is a more suitable candidate as it has a half-life of 4.2 days and is compatible with sequential imaging using microPET due to its longer biological half-life. Labeling techniques with radioiodine (123I, 124I, etc.) are well-defined and one can obtain the labeled products in good yield with radiochemical purity.190 Despite the complex decay scheme of 124I which results in only 25% abundance of positrons, (compared with 100% positron emission of 18F), in vivo quantitative imaging with 124I labeled antibodies has been successfully carried out under realistic conditions using a PET/CT scanner191 and 3D-dosimetry. As for now, 124I has been complexed to a variety of biomolecules such as, for example, monoclonal antibodies and dibodies,192 annexinV,193iodoazomycingalactoside (IAZG)194and 5-iodo-2'-fluoro-1-β-D-arabinofuranosyl uracil (FIAU).195 There are a few reports in the PDT field in which various 18F based radiotracers FDG,19618F-FHBG{9-(4-18F-fluoro-3-hydroxymethyl-butyl) guanine}197 and FLT (3′-deoxy-3′-18F-fluorothymidine) or 125I labeled photosensitizers198–200 have been used to monitor cellular events post PDT in mice at variable time intervals.
In their approach for developing “bifunctional agents” for PET and PDT, Pandey and co-workers have initially conjugated 3-(1′-hexyloxyethyl)-3-devinylpyropheophorbide-a (HPPH), an effective PS, to the mono- and di-bis-aminoethanethiols (N2S2 ligand).201 The in vivo biodistribution data of the related 99mTc complexes were collected in rats bearing Ward colon tumors at 4 h and 24 h post injection. However, the short 6 h half-life time of 99mTc was found to be incompatible with the 24 h imaging time (HPPH takes around 24 h to reach maximum accumulation in tumors), suggesting that the introduction of a longer lived isotope (e.g., 111In) in a tumor-avid PS could provide a more useful scanning agent with phototherapeutic properties.
To investigate the utility of 111In (half-life, 72 h) complexes of tumor-avid photosensitizer in tumor-imaging by SPECT, Chen et al. established the reaction conditions by reacting cold InCl3with HPPH 14 under various reaction conditions. The resulting In(III)-HPPH maintained its tumor avidity. In preliminary screening, compared to HPPH, the corresponding In(III) analog [In(III) HPPH] was found to be 40-fold more effective in vitro and 8-fold more effective in vivo with limited skin phototoxicity (no skin phototoxicity was observed at day 3 post injection of the photosensitizer).202 The SPECT (single photon emission computed tomography) imaging studies with the corresponding In-HPPH are currently in progress203
For synthesizing photosensitizers with PET imaging potential, the methyl 3-(1′-m-iodobenzyloxyethyl)-3-devinylpyropheo- phorbide-a36 (and the corresponding 124I-analog) were obtained in a sequence of reactions from pyropheophorbide-a and showed promising potential as a tumor-imaging phototherapeutic agent. The electrophilic aromatic iodination of the corresponding trimethylstannyl intermediate with Na124I in the presence of an Iodogen bead afforded 124I-labeled photosensitizer with >95% radioactive specificity.204 The non-labeled iodo-photosensitizer produced an excellent long-term tumor cure in mice. The photosensitizer also showed promising tumor fluorescence and PET imaging ability and this was the first report demonstrating the utility of an 124I-labeled photosensitizer as a “multimodality agent” for tumor imaging (PET/fluorescence) with and without PDT (Fig. 16, only comparative PET imaging with F-FDG is shown).204
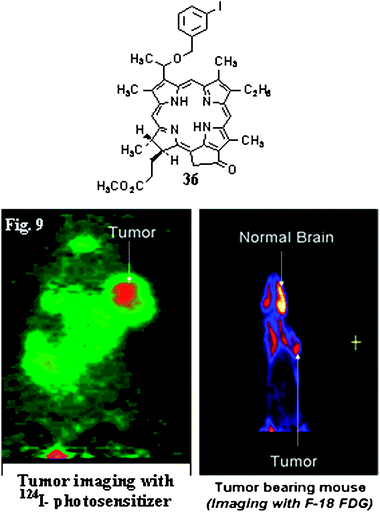 |
| Fig. 16 Comparative tumor-imaging potential of 124I-photosensitizer 36 and 18F-FDG in BALB/c mice bearing Colon26 tumors (J. Med. Chem. 2005, 48, 6286–6296). | |
This approach clearly demonstrates that relatively selective accumulation of the porphyrin-based PS provides an opportunity to be utilized as a single agent for imaging (PET and optical) and therapy. The techniques employed in this study are non-invasive and the use of a single agent rules out any chances for variability in the pharmacokinetic and pharmacodynamic pattern of the drug at any stage. This is in contrast to other studies where either more than one agent or a bioconjugate molecule in which tethered imaging probes are used, which could make significant changes in pharmacokinetic and pharmacodynamic characteristics of the individual moieties.
This methodology was further extended to purpurinimides, highly effective photosensitizers with long-wavelength absorption near 700 nm. A series of carbohydrate conjugated pyropheophorbides were also prepared to target certain carbohydrate recognized proteins, known for over-expression in certain tumors. Compared to non-carbohydrate analog, the corresponding galactose and glucose derivatives showed higher uptake in mice bearing either Colon26 or RIF tumors. However, the in vivo biodistribution results obtained from C3H mice bearing RIF tumors revealed that of the galactose and glucose conjugates, the galactose analog had higher tumor uptake.205 As expected, due to high uptake of the carbohydrate derivatives in liver and spleen, the parent molecule produced the best tumor contrast. However, using certain nanoparticles for blocking the liver and spleen uptake resulted in a remarkably reduced rate of uptake in these organs and produced substantial increase in tumor-uptake.
I. Future prospects
Though PDT has been mainly a cancer therapy, it has also shown enormous applications in many noncancerous conditions. Porphyrin-based photosensitizer (PpIX derived from ALA) fluorescence as a marker has proven capable of detecting lesions in the bladder not detectable by ordinary cystoscopy. FDA-approved fluorescence-endoscopic method called LIFE (Light Induced Fluorescence Excitation) has been shown at least 3-fold as likely to detect an early lesion when used with whitelight bronchoscopy. Unfortunately, porphyrin-based compounds have limited applications for detecting deeply seated tumors due to a small Stokes Shift between long-wavelength absorption and fluorescence bands, which limits their application in fluorescence imaging. Therefore, the recent approaches for developing photosensitizer-cynanine dye conjugates as bifunctional agents provides a unique approach for tumor-detection and therapy. The Roswell Park group has extended this approach in developing “Multifunctional Agents” for MR imaging-PDT and PET-imaging–PDT. Advances constantly being made in the design of targeting vehicles used for delivery therapies (e.g. multifunctional nanoparticles) could lead to the development of “Multifunctional Theranostics”.
Another application of PDT is for treating infectious disease. There has been an increasing international concern about multidrug-resistant bacteria and the spread of infectious disease, which has become untreatable by antibiotics and become a great concern in medical community. The potential of PDT with certain cationic photosensitizers (porphyrins and nonporphyrins) as antimicrobial agents is currently being explored as a simple and highly effective therapy for infectious disease.
Since the approval of Photofrin® by various health organizations, PDT has made many significant advances. The skin phototoxicity associated with most of the photosensitizers has significantly been reduced with new agents. For treating large and deeply seated tumors various 700–800 nm photosensitizers are currently in various stages of clinical trials. For developing PDT agents with imaging capability (multifunctional agents) improved tumor-specificity is still a challenge and several laboratories are investigating the use of activatable and nanoparticles approaches to achieve the desired goal of developing improved target-specific multimodality agents.
For future drug development, it is extremely important to have a strong collaboration between chemists, biologists, physicists and clinicians. The understanding of the effect of overall lipophilicity, aggregation characteristics and its impact on photophysical properties, the pharmacokinetic and pharmacodynamic characteristics of new compounds could be extremely useful in developing improved tumor-imaging and therapeutic agents.
Abbreviations
PDT
| Photodynamic Therapy |
NIR | Near Infra Red |
ROS |
Reactive Oxygen Species
|
PS
| Photosensitizer |
SAR | Structure–Activity Relationship |
QSAR
| Quantitative Structure Activity Relationship |
MRI | Magnetic Resonance Imaging |
PET |
Positron Emission Tomography |
CT | Computed Tomography |
SPECT
| Single Photon Emission Computed Tomography |
cRGD
| (cyclic-Arg-Gly-Asp) |
STAT
|
Signal Transducer and Activator of Transcription-3 |
ABCG-2
| ATPBinding Cassette, Sub-Family G, Member) |
Acknowledgements
RKP is highly thankful to all the members (past and present) of his research group and the collaborators, whose names are cited in the references. We are thankful to Dr Nestor Rigual and Michele Cooper, Clinical PDT for the clinical results (Fig. 9–11). The financial support from the NIH (CA 55791, CA 114053, CA-109914, CA 127369, CA 127369-02S1),CA-119358, Oishei Foundation, Oncologic Foundation of Buffalo and Roswell Park Alliance Foundation, The Mallinckrodt Medical Inc. St. Louis (USA) is highly appreciated. The authors are thankful to Mr Joseph Missert for his help in preparing this manuscript.
Notes and references
- R. K. Pandey, L. N. Goswami, Y. Chen, A. Gryshuk, J. R. Missert, A. Oseroff and T. J. Dougherty, Lasers Surg. Med., 2006, 38, 445–467 CrossRef.
- M. R. Detty, S. L. Gibson and S. J. Wagner, J. Med. Chem., 2004, 47, 3897–3915 CrossRef CAS.
- S. B. Brown, E. A. Brown and I. Walker, Lancet Oncol., 2004, 5, 497–508 CrossRef CAS.
- Z. Huang, Technology in Cancer Research & Treatment, 2005, 4, 283–293 Search PubMed.
- R. Bonnett and G. Martinez, Tetrahedron, 2001, 57, 9513–9547 CrossRef.
- T. J. Dougherty, C. J. Gomer, B. W. Henderson, G. Jori, D. Kessel, M. Korbelik, J. Moan and Q. Peng, J. Natl. Cancer Inst., 1998, 90, 889–905 CrossRef CAS.
-
M. R. Hamblin and P. Mroz, in Photodynamic Therapy in Advances in Photodynamic Therapy, Artech House, Boston, 2008 Search PubMed.
- I. J. MacDonald and T. J. Dougherty, J. Porphyrins Phthalocyanines, 2001, 5, 105–129 CrossRef CAS.
- L. D. Via and S. M. Magno, Curr. Med. Chem., 2001, 8, 1405–1418 CAS.
- J. S. McCaughan, Drugs Aging, 1999, 15, 49–68 CrossRef CAS.
- D. E. Dolmans, D. Fukumura and R. K. Jain, Nat. Rev. Cancer, 2003, 3, 380–387 CrossRef CAS.
- F.-P. Montforts, B. Gerlach and F. Hoeper, Chem. Rev., 1994, 94, 327–347 CrossRef CAS.
- H. Ali and J. E. Van Lier, Chem. Rev., 1999, 99, 2379–2450 CrossRef.
- I. Rosenthal, Photochem. Photobiol., 1991, 53, 859–870 CAS.
- D. Philips, Progress in Reaction Kinetics, 1997, 22, 175–300 Search PubMed.
- A. C. Tedesco, J. Rotta and C. G. Lunardi, Curr. Org. Chem., 2003, 7, 187–196 CAS.
- S. M. Ali and M. Olivo, Int. J. Oncol., 2003, 22, 1181–1191 CAS.
- D. Wöhrle, Russ. Chem. Bull., 1998, 47, 807–816 Search PubMed.
- K. Kalka, H. Merk and H. Mukhtar, J. Am. Acad. Dermatol., 2000, 42, 389–413 CrossRef CAS.
- E. l. Taylor and S. B. Brown, J. Dermatol. Treat., 2002, 13, s3–S11 CAS.
- C. J. Kelty, S. L. Marcus and R. Ackroyd, Dis. Esophagus, 2002, 15, 137–144 CrossRef CAS.
- T. G. Sutedjia and P. E. Postmus, J. Photochem. Photobiol., B, 1996, 36, 199–204 CrossRef.
- H. Kostron, A. Obwegeser and R. Jakober, J. Photochem. Photobiol., B, 1996, 36, 157–168 CrossRef CAS.
- J. Gahlen, R. L. Prosst and J. Stern, Chirurg, 2002, 73, 122–131 CrossRef CAS.
- N. L. Oleinick, R. L. Morris and I. Belichenko, Photochem. Photobiol. Sci., 2002, 1, 1 RSC.
- M. Otake, M. Nishiwaki, Y. Kobayashi, S. Baba, E. Kohno, T. Kawasaki, Y. Fujise and H. Nakamura, Br. J. Cancer, 2003, 89, 730–736 CrossRef CAS.
- R. K. Pandey and C. K. Herman, Chemistry & Industry, 1998, 21, 739–743 Search PubMed.
- N. Brasseur, T. L. Nguyen, R. Langlois, R. Ouellet, S. Marengo, D. Houde and J. E. Van Lier, J. Med. Chem., 1994, 37, 415–420 CrossRef CAS.
- B. C. Wilson and M. S. Patterson, Phys. Med. Biol., 2008, 53, R61 CrossRef CAS.
- C. M. Allen, W. M. Sharman and J. E. Van Lier, J. Porphyrins Phthalocyanines, 2001, 5, 161–169 CrossRef CAS.
- J. L. Sessler, T. Murai, V. Lynch and M. Cyr, J. Am. Chem. Soc., 1988, 110, 5586–5588 CrossRef CAS.
- J. L. Sessler, T. Murai and V. Lynch, Inorg. Chem., 1989, 28, 1333–1341 CrossRef CAS.
- B. G. Maiya, T. E. Mallouk, G. Hemmi and J. L. Sessler, Inorg. Chem., 1990, 29, 3738–3745 CrossRef CAS.
- J. L. Sessler, T. D. Mody, G. W. Hemmi and V. Lynch, Inorg. Chem., 1993, 32, 3175–3187 CrossRef CAS.
- S. W. Young, F. Qing, A. Harriman, J. L. Sessler, W. C. Dow, T. D. Mody, G. W. Hemmi, Y. Hao and R. A. Miller, Proc. Natl. Acad. Sci. U. S. A., 1996, 93, 6610–6615 CrossRef CAS.
-
T. D. Mody and L. Fu, J. L. Sessler, in Progress in Inorganic Chemistry, ed. K. D. Karlin, John Wiley and Sons, Chichester, 2001, vol. 49, pp. 551–598 Search PubMed.
- O. Rabb, Z. Biol. (Munich), 1900, 39, 524–546 Search PubMed.
-
R. K. Pandey and G. Zheng, in The Porphyrin Handbook, ed. K. M. Kadish, K. M. Smith and R. Guilard, Academic Press, Boston, 2000, vol. 6, pp. 157–230 Search PubMed.
- T. Tsuchida, G. Zheng, R. K. Pandey, W. R. Potter, D. A. Bellnier, B. W. Henderson, H. Kato and T. J. Dougherty, Photochem. Photobiol., 1997, 66, 224–228 CrossRef CAS.
- R. K. Pandey, F.-Y. Shiau, T. J. Dougherty and K. M. Smith, Tetrahedron, 1991, 47, 9571–9584 CrossRef CAS.
- T. J. Dougherty, J. E. Kaufman, A. Goldfarb, K. R. Weishaupt, D. Boyle and A. Mittleman, Cancer Res., 1978, 38, 2628–2635 CAS.
-
T. J. Dougherty and J. G. Levy, in Biomedical Photonics Handbook, ed. T. Vo-Dinh, CRC Press, New York, 2003, pp. 38-1–38-16 Search PubMed.
- T. J. Dougherty, Photochem. Photobiol., 1983, 38, 377–379 CrossRef CAS.
- D. Kessel, P. Thompson, B. Musselman and C. K. Chang, Photochem. Photobiol., 1987, 46, 563–568 CrossRef CAS.
- I. K. Morris and A. D. Ward, Tetrahedron Lett., 1988, 29, 2501–2504 CrossRef CAS.
- R. K. Pandey, T. J. Dougherty and K. M. Smith, Tetrahedron Lett., 1988, 29, 4657–4660 CrossRef CAS.
- P. A. Scourides, R. M. Böhmer, A. H. Kaye and G. Morstyn, Cancer Res., 1987, 47, 3439–3445 CAS.
- D. Kessel, P. Thompson, B. Musselman and C. K. Chang, Cancer Res., 1987, 47, 4642–4645 CAS.
- R. K. Pandey and T. J. Dougherty, Cancer Res., 1989, 8, 2042–2047.
- R. K. Pandey, F.-Y. Shiau, C. J. Medforth, T. J. Dougherty and K. M. Smith, Tetrahedron Lett., 1990, 31, 7399–7402 CrossRef CAS.
- M. C. Berenbaum, R. Bonnett and P. A. Scourides, Br. J. Cancer, 1982, 45, 571–581 CAS.
- C. J. Byrne, L. V. Marshallsay and A. D. Ward, J. Photochem. Photobiol., B, 1990, 6, 13–27 CrossRef CAS.
- W. M. Sharman, C. M. Allen and J. E. Van Lier, Methods Enzymol., 2000, 319, 376–400 CAS.
- J. Moan and S. Sommer, Cancer Res., 1985, 45, 1608–1610 CAS.
- J. D. Chapman, C. C. Stobbe, M. R. Arnfield, R. Santus, J. Lee and M. S. McPhee, Radiat. Res., 1991, 126, 73–79 CrossRef CAS.
- T. M. Busch, P. Wileyto, M. J. Emanuele, F. D. Piero, L. Marconato, E. Glatstein and C. J. Koch, Cancer Res., 2002, 62, 7273–7279 CAS.
- M. W. R. Reed, Surgery, 1989, 106, 94–99 CAS.
- B. C. Wilson, M. S. Patterson and L. Lilge, Lasers Med. Sci., 1997, 12, 182–199 CrossRef.
- G. Schermann, R. Schmidt, A. Völcker, H.-D. Brauer, H. Mertes and B. Franck, Photochem. Photobiol., 1990, 52, 741–744.
- B. Franck and A. Nonn, Angew. Chem., Int. Ed. Engl., 1995, 34, 1795–1811 CrossRef CAS.
- F. J. M. Hoeben, P. Johkheijm, E. W. Meijer and A. P. H. J. Schenning, Chem. Rev., 2005, 105, 1491–1546 CrossRef CAS.
- Y. Y. Tian, L. L. Wang and W. Wang, Laser Phys., 2008, 18, 1119–1123 CrossRef CAS.
- T. Sutedja, P. Baas, F. Stewart and N. van Zandwijk, Eur. J. Cancer, 1992, 28, 1370–1373 CrossRef.
- R. Whelpton, A. T. Michael-Titus, S. S. Basra and M. Grahn, Photochem. Photobiol., 1995, 61, 397 CrossRef CAS.
- T. R. Nathan, D. E. Whitelaw, S. C. Chang, W. R. Lees, P. M. Ripley, H. Payne, L. Jones, M. C. Parkinson, M. Emberton, A. R. Gillams, A. R. Mundy and S. G. Bown, J. Urol., 2002, 168, 1427–1432 CrossRef CAS.
- C. M. Moore, T. R. Nathan, W. R. Lees, C. A. Mosse, A. Freeman, M. Emberton and S. G. Bown, Lasers Surg. Med., 2006, 38, 356–363 CrossRef CAS.
- S. C. Chang, G. A. Buonaccorsi, A. J. MacRobert and S. G. Brown, Int. J. Cancer, 1996, 67, 555–562 CrossRef CAS.
- P. Wyss, V. Schwarz, D. Dobler-Girdziunaite, R. Hornung, H. Walt, A. Degen and M. K. Fehr, Int. J. Cancer, 2001, 93(5), 720–724 CrossRef CAS.
- A. K. D'Cruz, M. H. Robinson and M. A. Biel, Head Neck, 2004, 26, 232–40 CrossRef.
- L. Ayaru, S. G. Bown and S. P. Pereira, Int. J. Gastrointest. Cancer, 2005, 35, 1–14 Search PubMed.
- S. C. Chang, G. A. Buonaccorsi, A. J. MacRobert and S. G. Brown, Prostate, 1997, 32, 89–98 CrossRef CAS.
- T. J. Dougherty, G. Lawrence, J. H. Kaufman, D. Boyle, K. R. Weishaupt and A. Goldfarb, J. Natl. Cancer Inst., 1979, 62, 231–237 CAS.
- C. P. Lowdell, D. V. Ash, I. Driver and S. B. Brown, Br. J. Cancer., 1993, 67, 1398–403 CAS.
- J. C. Kennedy and R. H. Pottier, J. Photochem. Photobiol., B, 1992, 14, 275–292 CrossRef CAS.
- D. J. Robinson, P. Collins, M. R. Stringer, D. I. Vernon, G. I. Stables, S. B. Brown and R. A. Sheehan-Dare, Acta Derm.-Venereol., 1999, 79, 451–455 CrossRef CAS.
- I. M. Stender, R. Na, H. Fogh, C. Gluud and H. C. Wulf, Lancet, 2000, 355, 963–966 CrossRef CAS.
- W. Hongcharu, C. R. Taylor, Y. Chang, D. Aghassi, K. Suthamjariya and R. R. Anderson, J. Invest. Dermatol., 2000, 115, 183–192 CrossRef CAS.
. - I. M. Stender, J. Lock-Andersen and H. C. Wulf, Clin. Exp. Dermatol., 1999, 24, 154–159 CrossRef CAS.
- F. Cairnduff, M. R. Stringer, E. J. Hudson, D. V. Ash and S. B. Brown, Br. J. Cancer, 1994, 69, 605–608 CAS.
- G. I. Stables, M. R. Stringer, D. J. Robinson and D. V. Ash, Br. J. Dermatol., 1997, 136, 957–960 CrossRef CAS.
- C. A. Morton, C. Whitehurst, J. H. McColl, J. V. Moore and R. M. MacKie, Arch. Dermatol., 2001, 137, 319–324 CAS.
. - J. A. Leman, D. C. Dick and C. A. Morton, Clin. Exp. Dermatol., 2002, 27, 516–518 CrossRef CAS.
- T. Horio, O. Horio, H. Miyauchi-Hashimoto, M. Ohnuki and T. Isei, Br. J. Dermatol., 2003, 148, 1274–1276 CrossRef CAS.
- E. A. Coors and P. Von den Driesch, J. Am. Acad. Dermatol., 2004, 50, 363–367 CrossRef.
- M. H. Gold, V. L. Bradshaw, M. M. Boring, T. M. Bridges, J. A. Biron and T. L. Lewis, J. Drugs Dermatol., 2004, 3, S6–S9 Search PubMed.
- A. R. Oseroff, S. Shieh, N. P. Frawley, R. Cheney, L. E. Blumenson, E. K. Pivnick and D. A. Bellnier, Arch. Dermatol., 2005, 141, 60–67.
- D. W. Hunt, Rostaporfin (Miravant Medical Technologies), IDrugs, 2002, 5, 180–186 Search PubMed.
- B. Krammer and K. Plaetzer, Photochem. Photobiol. Sci., 2008, 7, 283–9 RSC.
- D. Zaak, R. Sroka, M. Höppner, W. Khoder, O. Reich, S. Tritschler, R. Muschter, R. Knüchel and A. Hofstetter, Med. Laser Appl., 2003, 18, 91–95 CrossRef.
- R. A. His, A. Kapatkin, J. Strandberg, T. Zhu, T. Vulcan, M. Solonenko, C. Rodriguez, J. Chang, M. Saunders, N. Mason and S. Hahn, Clin. Cancer Res., 2001, 7, 651–660 CAS.
- J. H. Pinthus, A. Bogaards, R. Weersink, B. C. Wilson and J. Trachtenberg, J. Urol., 2006, 175, 1201–1207 Search PubMed.
- K. Verigos, D. C. Stripp, R. Mick, T. C. Zhu, R. Whittington, D. Smith and A. Dimofte, J. Environ Pathol. Toxicol. Oncol., 2006, 25, 373–387 Search PubMed.
- H. Patel, R. Mick, J. Finlay, T. C. Zhu, E. Rickter, K. A. Cengel, S. B. Malkowicz, S. M. Hahn and T. M. Busch, Clin. Cancer Res., 2008, 14, 4869–4876 CrossRef CAS.
- D. C. H. Stripp, R. Mick, T. C. Zhu, R. Whittington, D. Smith, A. Dimofte, J. Finlay, J. Miles, T. M. Busch, D. Shin1, A. Kachur, Z. A. Tochner, S. B. Malkowicz, E. Glatstein and S. M. Hahn, Proc. of SPIE 5315, 2004, 88–99 Search PubMed.
- T. C. Zhu, A. Dimofte, J. C. Finlay, D. Stripp, T. Busch, J. Miles, R. Whittington, S. B. Malkowicz, Z. Tochner, E. Glatstein and S. M. Hahn, Photochem. Photobiol., 2005, 81, 96–105 CrossRef CAS.
- A. Dimofte, T. C. Zhou, S. M. Hahn and R. A. Lustig, Lasers Surg. Med., 2002, 31, 305–312 CrossRef.
- J. I. Lim, Ophthalmology Clinics of North America, 2002, 15, 473–478 Search PubMed.
- M. R. Gertner, A. Bogaards, R. A. Weersink, S. A. McCluskey, M. A. Haider, C. K. K. Yue, J. Savard, S. Simpson, P. H. Brun, P. Cohen, A. Scherz, Y. Salomon, A. G. Aprikian, M. M. Elhilali, B. C. Wilson and J. Trachtenberg, Eur. Urol. Suppl., 2004, 3, 212 CrossRef.
- N. E. Martin and S. M. Hahn, Photodiag. Photodyn. Therapy 1, 2004, 123–136 Search PubMed.
- R. A. Weersink, A. Bogaards, M. Gertner, S. R. H. Davidson, K. Zhang, G. Netchev, T. Trachtenberg and B. C. Wilson, J. Photochem. Photobiol., B, 2005, 79(3), 211–222 CrossRef CAS.
- M. Berdugo, R. A. Bejjani, F. Valamanesh, M. Savoldelli, J.-C. Jeanny, D. Blanc, H. Ficheux, A. Scherz, Y. Salomon, D. BenEzra and F. Behar-Cohen, Invest. Ophthalmol. Visual Sci., 2008, 49, 1633–1644 CrossRef.
- C. Moore, D. Huebler, T. Zimmermann, L. A. J. Heinemann, F. Saad and D. M. Thai, Eur. Urol., 2004, 46, 80–87 CrossRef.
- O. Mazor, A. Brandis, V. Plaks, E. Neumark, V. Rosenbach-Belkin, Y. Salomon and A. Scherz, Photochem. Photobiol., 2005, 81, 342–351 CrossRef CAS.
- A. Ferrario, D. Kessel and C. J. Gomer, Cancer Res., 1992, 52, 2890–2893 CAS.
- L.-M. W. Song, K. K. Wang and A. R. Zinsmeister, Cancer, 1998, 82, 421–427 CrossRef.
- J. A. Hargus, F. R. Fronczek, M. G. Vicente and K. M. Smith, Photochem. Photobiol., 2007, 83, 1006–15 CrossRef CAS.
- A. Juzeniene, Photodiagn. Photodyn. Ther., 2009, 6(2), 94–96 CrossRef.
- J. Jankun, L. Lilge, A. Douplik, R. W. Keck, M. Pestka, M. Szkudlarek, P. J. Stevens, R. J. Lee and S. H. Selman, J. Urol., 2004, 172, 739–743 CrossRef CAS.
- S. H. Selman and R. W. Keck, J. Urol., 1994, 152, 2129–2132 Search PubMed.
- W. M. Sharman, C. M. Allen and J. E. van Lier, Drug Discovery Today, 1999, 4, 507 CrossRef CAS.
- A. E. O'Connor, W. M. Gallagher and A. T. Byrne, Photochem. Photobiol., 2009, 85, 1053–1074 CrossRef CAS.
- S. J. Keam, L. J. Scott and M. P. Curran, Drugs, 2003, 63, 2521–2554 CrossRef CAS.
- R. K. Pandey, A. B. Sumlin, W. R. Potter, D. A. Bellnier, B. W. Henderson, M. Aoudia, M. R. Rodgers, K. M. Smith and T. J. Dougherty, Photochem. Photobiol., 1996, 64, 194–205 CrossRef CAS.
- B. W. Henderson, D. A. Bellnier, W. R. Greco, A. Sharma, R. K. Pandey, L. A. Vaughan, K. R. Weishaupt and T. J. Dougherty, Cancer Res., 1997, 57, 4000 CAS.
- N. Rigual, et al., unpublished results.
- G. M. Loewen, R. K. Pandey, D. Bellnier, B. W. Henderson and T. J. Dougherty, Lasers Surg. Med., 2006, 38, 364 CrossRef.
- D. A. Bellnier, W. R. Greco, G. M. Loewen, H. Nava, A. R. Oseroff and T. J. Dougherty, Lasers Surg. Med., 2006, 38, 439 CrossRef.
- D. A. Bellnier, W. R. Greco, G. M. Loewen, A. O. Oseroff and T. J. Dougherty, Cancer Chemother. Pharmacol., 2006, 57, 40 CrossRef CAS.
- Y. Chen, R. Miclea, T. Srikrisnan, B. Balsubramanium, T. J. Dougherty and R. K. Pandey, Bioorg. Med. Chem. Lett., 2005, 15, 3189 CrossRef CAS.
- D. A. Bellnier, W. R. Greco, G. M. Loewen, H. Nava, A. Oseroff, R. K. Pandey and T. J. Dougherty, Cancer Research, 2003, 63, 1806 CAS.
- D. A. Bellnier, W. R. Greco, H. Nava, G. M. Loewen, A. R. Oseroff and T. J. Dougherty, Cancer Chemother Pharmacol., 2004 Search PubMed.
- T. M. Anderson, T. J. Dougherty, D. F. Tan, A. Sumlin, J. M. Schlossin and P. M. Kanter, Anticancer Research, 2003, 23, 3713 CAS.
- J. Lobel, I. MacDonald, M. J. Ciesielski, T. Barone, W. R. Potter, J. Pollina, R. J. Plunkett, R. A. Fenstermaker and T. J. Dougherty, Lasers Surg. Med., 2001, 29, 397 CrossRef CAS.
- T. J. Dougherty, R. K. Pandey, H. Nava, J. A. Smith, H. O. Douglass, S. B. Edge, D. A. Bellnier, L. O'Malley and M. Cooper, Proc. SPIE, 2000, 3909, 25 CAS.
- W. R. Potter, B. W. Henderson, D. A. Bellnier, R. K. Pandey, L. A. Vaughan, K. R. Weishaupt and T. J. Dougherty, Photochem. Photobiol., 1999, 70, 781 CAS.
- M. L. Magne, C. O. Rodriguez, A. A. Autry, B. F. Edwards, A. P. Theon and B. R. Madewell, Lasers Surg. Med., 1997, 20, 202 CrossRef CAS.
- K. Furukawa, D. H. Green, T. S. Mang, H. Kato and T. J. Dougherty, Proc. SPIE, 1994, 2133, 170 CAS.
- K. Furukawa, D. H. Green, T. S. Mang, H. Kato and T. J. Dougherty, Proc. SPIE, 1994, 2371, 510.
- D. A. Bellnier, B. W. Henderson, R. K. Pandey, W. R. Potter and T. J. Dougherty, J. Photochem. Photobiol., B, 1993, 20, 55 CrossRef CAS.
- T. J. Dougherty and J. G. Levy, Photodynamic Therapy and Clinical Applications, in Biomedical Photonics Handbook, ed. T. Vo-Dinh, CRC Press, Boca Raton, ch. 38, pp. 1–16 Search PubMed.
- G. Zheng, W. R. Potter, S. H. Camacho, J. R. Missert, G. Wang, D. A. Bellnier, B. W. Henderson, M. A. J. Rodgers, T. J. Dougherty and R. K. Pandey, J. Med. Chem., 2001, 44, 1540 CrossRef CAS.
- A. Gryshuk, A. Graham, R. K. Pandey, W. R. Potter, J. R. Missert, A. Oseroff, T. J. Dougherty and R. K. Pandey, Photochem. Photobiol., 2002, 76, 555 CrossRef CAS.
- Y. Chen, A. Graham, W. Potter, J. Morgan, L. Vaughan, D. A. Bellnier, B. W. Henderson, A. Oseroff, T. J. Dougherty and R. K. Pandey, J. Med. Chem., 2002, 45, 255 CrossRef CAS.
- A. Gryshuk, Y. Chen, L. N. Goswami, S. K. Pandey, J. Missert, T. Ohulchanskyy, W. Potter, P. N. Prasad and R. K. Pandey, J. Med. Chem., 2007, 50, 1754 CrossRef CAS.
- R. K. Pandey, Synthetic Strategies in Designing Porphyrin-Based Photosensitizers for Photodynamic Therapy, in Organic Photochemistry and Photobiology, ed. W. Horspool and F. Lenci, CRC Press, Boca Raton, ch. 144, p. 1–21 Search PubMed.
- S. Mettath, M. Shibata, J. L. Alderfer, M. O. Senge, K. M. Smith, R. Rein, T. J. Dougherty and R. K. Pandey, J. Org. Chem., 1998, 63, 1646 CrossRef CAS.
- H. J. Callott, A. W. Johnson and A. J. Sweeny, J. Chem. Soc., Perkin Trans. 1, 1973, 1424 RSC.
- R. K. Pandey, W. R. Potter, I. Meunier, A. B. Sumlin and K. M. Smith, Photochem. Photobiol., 1995, 62, 764 CrossRef CAS.
- G. Li, A. Graham, Y. Chen, M. P. Dobhal, J. Morgan, A. Oseroff, T. J. Dougherty and R. K. Pandey, J. Med. Chem., 2003, 46, 5349 CrossRef CAS.
- R. K. Pandey, M. Isaac, I. MacDonald, M. O. Senge, T. J. Dougherty and K. M. Smith, J. Org. Chem., 1997, 62, 1463 CrossRef CAS.
. - C. Liu, M. P. Dobhal, M. Ethirajan, J. R. Missert and R. K. Pandey, J. Am. Chem. Soc., 2008, 130, 14311 CrossRef CAS.
- M. Ethirajan, N. J. Patel and R. K. Pandey, Porphyrin-based multifunctional agentsd for tumor-imaging and therapy, in The Porphyrin Handbook, ed. K. M. Kadish, K. M. Smith and R. Guilard, Academic Press, Boston, 2010, in press Search PubMed.
- X. Zheng, J. Morgan, S. K. Pandey, Y. Chen, E. Tracy, H. Baumann, J. R. Missert, C. Batt, J. Jackson, D. A. Bellnier, B. W. Henderson and R. K. Pandey, J. Med. Chem., 2009, 52, 4306 CrossRef CAS.
- A. Srivatsan, et al., unpublished results.
- R. Weissleder and V. Ntziachristos, Nat. Med., 2003, 9, 123 CrossRef CAS.
- J. Chen, T. W. B. Liu, P. C. Lo, B. C. Wilson and G. Zheng, Bioconjugate Chem., 2009, 20, 1836 CrossRef CAS.
- T. Lammers, V. Subr, P. Peschke, R. Kühnlein, W. E. Hennink, K. Ulbrich, F. Kiessling, M. Heilmann, J. Debus, P. E. Huber and G. Storm, Br. J. Cancer, 2008, 99, 900 CrossRef CAS.
- J. Culver, W. Akers and S. Achilefu, J. Nucl. Med., 2008, 49, 169 CrossRef.
- J. E. Bowsher, V. E. Johnson, T. G. Turkington, R. J. Jaszczak, C. E. Floyd Jr. and R. Edward Coleman, IEEE Trans. Med. Imaging, 1996, 15, 673 CrossRef CAS.
- C. Catana, D. Procissi, Y. Wu, M. S. Judenhofer, J. Qi, B. J. Pichler, R. E. Jacobs and S. R. Cherry, Proc. Natl. Acad. Sci. U. S. A., 2008, 105, 3705 CrossRef CAS.
- M. Rudin and R. Weissleder, Nat. Rev. Drug Discovery, 2003, 2, 123 CrossRef CAS.
- S. S. Gambhir, Nat. Rev. Cancer, 2002, 2, 683 CrossRef CAS.
- F. J. Bonte, T. S. Harris, L. S. Hynan, E. H. Bigio and C. L. White III, Clin. Nucl. Med., 2006, 31, 376 CrossRef.
- D. O. Cosgrove, H. B. Meire, A. Lim and R. J. Eckersley, in Ultrasound: General Principles, ed. R. G. Grainger and D. J. Allison, Diagnostic Radiology: A Textbook of Medical Imaging, Churchill Livingstone, New York, 2001, ch. 3 Search PubMed.
- A. Policard, J. Soc. Biol., 1924, 91, 1423 Search PubMed.
- T. S. Mang, C. McGinnis, C. Liebow, U. O Nseyo, D. H. Crean and T. J. Dougherty, Cancer, 1993, 71, 269 CrossRef CAS.
- R. K. Pandey, N. James, Y. Chen and M. P. Dobhal, Cyanine dye-based compounds for tumor imaging with and without photodynamic therapy, in Heterocyclic Polymethine Dyes, ed. L. Strekowski, Springer-Verlag, Berlin, 2008, p. 41 Search PubMed.
- S. Achilefu, S. Bloch, M. A. Markiewicz, T. Zhong, Y. Ye, R. B. Dorshow, B. Chance and K. Liang, Proc. Natl. Acad. Sci. U. S. A., 2005, 102, 7976 CrossRef CAS.
- D. Artemov, N. Mori, B. Okollie and Z. M. Bhujwalla, Magn. Reson. Med., 2003, 49, 403 CrossRef CAS.
- L. Josephson, U. Mahmood, P. Wunderbaldinger, Y. Tang and R. Weissleder, Mol. Imaging, 2003, 2, 18 CrossRef.
- J. E. Bugaj, S. Achilefu, R. B. Dorshow and R. Rajagopalan, J. Biomed. Opt., 2001, 6, 122 CrossRef CAS.
- Z. Zhang and S. Achilefu, Org. Lett., 2004, 6, 2067 CrossRef CAS.
- J. H. Flanagan Jr., S. H. Khan, S. Menchen, S. A. Soper and R. P. Hammer, Bioconjugate Chem., 1997, 8, 751 CrossRef CAS.
- W. K. Moon, Y. Lin, T. O'Loughlin, Y. Tang, D. Kim, R. Weissleder and C. Tung, Bioconjugate Chem., 2003, 14, 539 CrossRef CAS.
- V. Ntziachristos, J. Ripoll, L. V. Wang and R. Weissleder, Nat. Biotechnol., 2005, 23, 313 CrossRef CAS.
- J. V. Frangioni, Curr. Opin. Chem. Biol., 2003, 7, 626 CrossRef.
- Y. Chen, A. Gryshuk, S. Achilefu, T. Ohulchansky, W. Potter, T. Zhong, J. Morgan, B. Chance, P. N. Prasad, B. W. Henderson, A. Oseroff and R. K. Pandey, Bioconjugate Chem., 2005, 16, 1264 CrossRef CAS.
- S. K. Pandey, Y. Chen, R. H. Zawada, A. Oseroff and R. K. Pandey, Proceedings of SPIE 2006, vol. 6139, p. 613905 Search PubMed.
- S. C. Davis, B. W. Pogue, H. Dehghani and K. D. Paulsen, J. Biomed. Opt., 2005, 10, 050501 CrossRef.
- R. W. Redmond, Photophysics and Photochemistry, in Photodynamic Therapy in Advances in Photodynamic Therapy, ed. M. R. Hamblin and P. Mroz, Artech House, Boston, 2008 Search PubMed.
- D. Muffoletto, A. Gupta, Z. Xu, C. Mahrer, G. Bauer, S. Galas, R. K. Pandey and U. Sunar, Proceedings of SPIE, 2009, 7164, 716409 Search PubMed.
- Y. Ni, K. Adzamli, Y. Miao, E. Cresens, J. Yu, M. P. Periasamy, M. D. Adams and G. Marchal, Invest. Radiol., 2001, 36, 97 CrossRef CAS.
- J. Krzystek, J. Telser, L. A. Pardi, D. P. Goldberg, B. M. Hoffman and L. Brunel, Inorg. Chem., 1999, 38, 6121 CrossRef CAS.
- G. Li, A. Slansky, M. P. Dobhal, L. N. Goswami, A. Graham, Y. Chen, P. Kanter, R. A. Alberico, J. Spernyak, J. Morgan, R. Mazurchuk, A. Oseroff, Z. Grossman and R. K. Pandey, Bioconjugate Chem., 2005, 16, 32 CrossRef.
- B. Hofmann, A. Bogdanov Jr., E. Marecos, W. Ebert, W. Semmler and R. Weissleder, J. Magn. Reson. Imaging, 1999, 9, 336 CrossRef CAS.
- R. K. Pandey, et al., unpublished results.
- R. K. Pandey, et al., unpublished results.
- R. K. Pandey, et al., unpublished results.
- K. Licha and C. Olbrich, Adv. Drug Delivery Rev., 2005, 57, 1087 CrossRef CAS.
- X. Zhang, W. Cai, F. Cao, E. Schreibmann, Y. Wu, J. C. Wu, L. Xing and X. Chen, J. Nucl. Med., 2006, 47, 492 CAS.
- T. Bury, A. Dowlati, P. Paulus, R. Hustinx, M. Radermecker and P. Rigo, Eur. J. Nucl. Med. Mol. Imaging, 1996, 23, 204 CrossRef CAS.
- L. P. Adler, H. F. Blair, J. T. Makley, R. P. Williams, M. J. Joyce, G. Leisure, N. Al-Kaisi and F. Miraldi, J. Nucl. Med., 1991, 32, 1508 CAS.
- P. Price and T. Jones, European Journal of Cancer Part A: General Topics, 1995, 31, 1924 Search PubMed.
- J. Okada, H. Oonishi, K. Yoshikawa, J. Itami, K. Uno, K. Imaseki and N. Arimizu, Ann. Nucl. Med., 1994, 8, 187 Search PubMed.
- B. Nakata, Y. Chung, S. Nishimura, T. Nishihara, Y. Sakurai, T. Sawada, T. Okamura, J. Kawabe, H. Ochi and M. Sowa, Cancer, 1997, 79, 695 CrossRef CAS.
- M. Oshida, K. Uno, M. Suzuki, T. Nagashima, H. Hashimoto, H. Yagata, T. Shishikura, K. Imazeki and N. Nakajima, Cancer, 1998, 82, 2227 CrossRef CAS.
- T. M. Guerrero, E. J. Hoffman, M. Dahlbom, P. D. Cutler, R. A. Hawkins and M. E. Phelps, IEEE Trans. Nucl. Sci., 1990, 37, 676 CrossRef.
- P. S. Conti, D. L. Lilien, K. Hawley, J. Keppler, S. T. Grafton and J. R. Bading, Nucl. Med. Biol., 1996, 23, 717 CrossRef CAS.
- D. Delbeke, D. M. Rose, W. C. Chapman, C. W. Pinson, J. K. Wright, R. D. Beauchamp, Y. Shyr and S. D. Leach, J. Nucl. Med., 1999, 40, 1784 CAS.
- M. E. Phelps, Proc. Natl. Acad. Sci. U. S. A., 2000, 97, 9226 CrossRef CAS.
- I. Verel, G. W. M. Visser and G. A. Van Dongen, J. Nucl. Med., 2005, 46, 164S.
- K. S. Pentlow, M. C. Graham, R. M. Lambrecht, N. V. Cheung and S. M. Larson, Med. Phys., 1991, 18, 357 CrossRef CAS.
- F. T. Lee, C. Hall, A. Rigopoulos, J. Zweit, K. Pathmaraj, G. J. O'Keefe, F. E. Smyth, S. Welt, L. J. Old and A. M. Scott, J. Nucl. Med., 2001, 42, 764 CAS.
- D. R. Collingridge, M. Glaser, S. Osman, H. Barthel, O. C. Hutchinson, S. K. Luthra, F. Brady, L. Bouchier-Hayes, S. J. Martin, P. Workman, P. Price and E. O. Aboagye, Br. J. Cancer, 2003, 89, 1327 CrossRef CAS.
- P. Zanzonico, J. O'Donoghue, J. D. Chapman, R. Schneider, S. Cai, S. Larson, B. Wen, Y. Chen, R. Finn, S. Ruan, L. Gerweck, J. Humm and C. Ling, Eur. J. Nucl. Med. Mol. Imaging, 2004, 31, 117 CrossRef.
- J. J. Bennett, J. Tjuvajev, P. Johnson, M. Doubrovin, T. Akhurst, S. Malholtra, T. Hackman, J. Balatoni, R. Finn, S. M. Larson, H. Federoff, R. Blasberg and Y. Fong, Nat. Med., 2001, 7, 859 CrossRef CAS.
- J. V. Moore, M. L. Waller, S. Zhao, N. J. F. Dodd, P. D. Acton, A. P. Jeavons and D. L. Hastings, Eur. J. Nucl. Med. Mol. Imaging, 1998, 25, 1248 CrossRef CAS.
- D. Dong, L. Dubeau, J. Bading, K. Nguyen, M. Luna, H. Yu, G. Gazit-Bornstein, E. M. Gordon, C. Gomer, F. L. Hall, S. S. Gambhir and A. S. Lee, Hum. Gene Ther., 2004, 15, 553 CrossRef CAS.
- P. Westermann, T. Glanzmann, S. Anderjevic, D. R. Braichotte, M. Forrer, G. A. Wagnieres, P. Monnier, H. D. Van den Bergh, J. Mach and S. Folli, Int. J. Cancer, 1998, 76, 842 CrossRef CAS.
- M. Sugiyama, H. Sakahara, K. Sato, N. Harada, D. Fukumoto, T. Kakiuchi, T. Hirano, E. Kohno and H. Tsukada, J. Nucl. Med., 2004, 45, 1754 CAS.
- B. Ma, G. Li, P. Kanter, D. Lamonica, Z. Grossman and R. K. Pandey, J. Porphyrins Phthalocyanines, 2003, 7, 500 CAS.
- Y. Chen, X. Zheng, M. P. Dobhal, A. Gryshuk, J. Morgan, T. J. Dougherty, A. Oseroff and R. K. Pandey, J. Med. Chem., 2005, 48, 3692 CrossRef CAS.
- R. K. Pandey, et al., unpublished results.
- S. K. Pandey, A. L. Gryshuk, M. Sajjad, X. Zheng, Y. Chen, M. A. Abouzeid, J. Morgan, I. Charamisinau, H. A. Nabi, A. Oseroff and R. K. Pandey, J. Med. Chem., 2005, 48, 6286 CrossRef CAS.
- S. K. Pandey, M. Sajjad, Y. Chen, X. Zheng, R. Yao, J. R. Missert, C. Batt, H. A. Nabi, A. R. Oseroff and R. K. Pandey, J. Med. Chem., 2009, 52, 445 CrossRef CAS.
|
This journal is © The Royal Society of Chemistry 2011 |