DOI:
10.1039/B901793N
(Tutorial Review)
Chem. Soc. Rev., 2011,
40, 306-319
Liquid-crystal nanoscience: an emerging avenue of soft self-assembly
Received
21st May 2010
First published on 2nd December 2010
Abstract
Liquid crystals are finding increasing applications in a wide variety of fields including liquid-crystal display technology, materials science, bioscience, etc., apart from acting as prototype self-organizable supramolecular soft materials and tunable solvents. Recently, keeping in pace with topical science, liquid crystals have entered into the fascinating domains of nanoscience and nanotechnology. This tutorial review describes the recent and significant developments in liquid-crystal nanoscience embracing contemporary nanomaterials such as nanoparticles, nanorods, nanotubes, nanoplatelets, etc. The dispersion of zero-, one- and two-dimensional nanomaterials in liquid crystals for the enhancement of properties, liquid-crystalline phase behavior of nanomaterials themselves, self-assembly and alignment of nanomaterials in liquid-crystalline media, and the synthesis of nanomaterials by using liquid crystals as ‘templates’ or ‘precursors’ have been highlighted and discussed. It is almost certain that the ‘fourth state of matter’ will play more prevalent roles in nanoscience and nanotechnology in the near future. Moreover, liquid-crystal nanoscience reflects itself as a beautiful demonstration of the contemporary theme “crossing the borders: science without boundaries”.
1. Introduction
Liquid crystals (LCs) are self-assembled dynamic functional soft materials which possess both order and mobility at molecular, supramolecular and macroscopic levels.1–3 Liquid crystals beautifully demonstrate the powerful general organization principle of matter by maximizing the interaction energy and minimizing the excluded volume. LCs exhibit extreme sensitivity to small external perturbations such as electric and magnetic fields, and surface effects, which is the basis for their information display applications.4 However, high-tech high-performance ‘hard’ materials like Kevlar which act as energy-absorbing bullet-proof guards used by defence personnel and law-enforcing agents, have been obtained from ‘soft’ liquid-crystalline materials.5 Liquid crystals are prototype self-assembling materials since they involve almost all kinds of supramolecular interactions such as van der Walls interaction, dipolar and quadrupolar interactions, charge transfer and π–π interaction, metal coordination and hydrogen bonding etc.4 The domain of liquid crystals spans across multiple disciplines of pure and applied science including bioscience and materials science.4
The liquid-crystalline state of matter exists between the crystalline solid and amorphous liquid states. For this reason LCs are referred to as intermediate phases or mesophases. However, the LC states are true thermodynamic stable states of matter like solids, liquids and gases are. Hence LCs are also often called as the fourth state of matter. Liquid crystals share the anisotropic (direction dependent) properties of crystalline solids and fluid properties of isotropic liquids. The constituents of the mesophases are called mesogens and the mesogens can be organic, inorganic and organometallics in nature.4 Molecular shape, microsegregation of incompatible parts, specific molecular interactions, self-assembly and self-organization are important factors that drive the formation of various LC phases in matter. Although there are various ways of classifying LCs depending on molecular features (i.e. calamitic, discotic and bent-core) and supramolecular assemblies (i.e. nematic, smectic, columnar etc.); the most commonly used classification is based on how the liquid-crystalline phases have been obtained, i.e. thermotropic and lyotropic phases. Thermotropic LC phases are obtained by the effect of temperature on pure compounds or a mixture of compounds either by heating the crystalline solid or by cooling an isotropic liquid. On the other hand, lyotropic liquid-crystalline (LLC) phases are achieved by dissolving amphiphilic compounds in suitable solvents over a range of concentrations and temperature.
Liquid crystals are even more promising because of their important role in biology.6 In a more general sense, the combination of order and mobility, as exhibited by liquid crystals, is a basic requirement for self-organization and structure formation in living systems. Major classes of biological compounds including lipids, proteins, carbohydrates and nucleic acids have been found to exist in various liquid-crystalline phases in vivo as well as in vitro under well-defined conditions.6 Their liquid-crystalline structure is believed to have a very important role in their biological functions and self-assembly processes.6 Moreover, LCs possess various properties analogous to living cells such as amplification and transmission of information and properties.2 Like living cells, they also respond to a large number of external stimuli such as light, heat and electric and magnetic fields as well as to the changes in chemical environment.4 Furthermore, the existence of liquid-crystalline phases of large and small DNAsin vivo and in vitro has already been related to the significant role they would have played in the evolution of biological information in the prebiotic world.7
Though liquid crystals are primarily known for their technological applications in liquid-crystal display (LCD) devices for information displays, nevertheless the ‘beyond-display’ applications of liquid crystals are equally important and numerous. Liquid crystals can potentially be used as new functional materials for electron, ion, molecular transporting, sensory, catalytic, optical and bioactive materials.1 Recently, their biomedical applications such as in controlled drug delivery, protein binding, phospholipid labeling and in microbe detection have been demonstrated.8 Owing to their dynamic nature, photochemically, thermally or mechanically induced structure changes of liquid crystals can be used for the construction of stimuli-responsive multifunctional materials.1
Liquid crystals are now playing a very significant role in nanoscience and nanotechnology.9–12 Liquid-crystal nanoscience primarily deals with the synergetic relationship between liquid crystals and nanomaterials. Nanoscale particles do not induce significant distortions of LC phases. Therefore various nanomaterials have been dispersed and studied in LC medium to enhance the physical properties of LCs.10 Moreover, alignment and self-assembly of nanomaterials themselves can be achieved in LC phases.9 Liquid crystals act as tunable solvents for the dispersion of nanomaterials, and being anisotropic media, they provide a very good support for the self-assembly of nanomaterials into larger organized structures in multiple dimensions. Hence LC mediated self-assembly can be efficiently used to organize different kinds of nanomaterials into soft and well-defined functional superstructures.10 Furthermore, since the liquid-crystal medium responds to small external stimuli, the dispersed materials can be forced to follow the order of the host medium which elaborates the controlled dynamic self-assembly and (re)orientations of the dispersed nanomaterials. It is interesting to note that LC nanoscience provides equal opportunity and credit to both thermotropic and lyotropic LCs, unlike materials science and life science which give preferences to the former and latter respectively. This review deals with liquid-crystalline nanomaterials, nanomaterials in liquid crystals, as well as synthesis of nanomaterials using LCs as ‘templates’ or ‘precursors’.
Nanoparticles (NPs) constitute a major class of nanomaterials. The diameters of nanoparticles vary between one to few hundred nanometres. NPs exhibit unusual features markedly different from those of the bulk materials. Moreover the electronic, optical, magnetic and chemical characteristics of nanoparticles are both size- and shape-dependent. NPs can be amorphous or crystalline in nature. Crystalline nanoparticles are referred to as nanocrystals. The dimensions of nanocrystals are so close to atomic dimensions that an unusually high fraction of the total atoms would be present on their surfaces. The increase in surface area possesses great significance in catalysis. Nanoscale particles are generally assumed to be spherical. However, an interesting interplay exists between the surface morphology and the packing arrangement, especially in small nanocrystals. Nanocrystals usually have a polyhedral surface morphology. Nanoparticles also lie in size between that of bulk materials and atoms and provide greater insights into how different properties evolve from atoms to bulk materials and vice versa. The search for the cross-over and/or emergence of properties have led to systematic investigation of nanoparticles by both ‘top-down’ and ‘bottom-up’ approaches in nanotechnology. The electronic absorption of nanocrystals of metals is dominated by the surface plasmon resonance (SPR) band which arises due to the collective coherent excitement of the free electrons within the conduction band. The focus of current research worldwide is to design nanomaterials that are able to self-assemble into functional superstructures in multiple directions. Nanoparticles assembly has been induced by several methods; however, using liquid crystals as an organizing medium to induce the self-assembly of nanoparticles is a powerful tool. Since a great variety of mesophase morphologies can be achieved to control the structure in one or more dimensions. The self-organization of nanomaterials in two- and three-dimensional space offered by the LC medium seems to be an ideal vehicle to explore and control the organization of matter on the nanometre to the micrometre scale which is a key to the further development of nanotechnology. One of the pursued classes of nanomaterials is metal nanoparticles. Gold nanoparticles (GNPs) are likely the best understood (chemical properties and stabilizing techniques) and therefore the most frequently studied nanoparticles. Consequently GNPs have been designed to exhibit liquid-crystalline behavior as well, as they have been dispersed into thermotropic and lyotropic liquid-crystalline phases, to modify the properties of the hosts.
The synthesis of monolayer-protected gold nanoparticles in organic solvents by Brust and co-workers has opened up a whole new field in materials science.13 The Brust method followed by several recent advances has allowed the facile synthesis of stable gold nanoparticles passivated with a variety of chemical functionalities. Alkane thiol-protected gold metal clusters are promising materials, since they can be handled in a similar manner as general organic compounds, due to their high stability to light and air, and solubility in conventional organic solvents. Moreover, a variety of chemical reactions can be performed on functionalized gold nanoparticles. By providing gold nanoparticles with liquid-crystalline properties, the ability to self-assembly could be introduced, and the reversible control of morphology of their aggregates by external stimuli could be accomplished. Furthermore, the reversible control of their properties would increase their chances of being potential functional nanomaterials. Keeping the above ideas in mind gold nanoparticles covered with a liquid-crystalline compound were successfully prepared.14 From its characteristic double-melting behavior in calorimetric studies, the existence of mesomorphism was speculated. However, neither the type of mesophase, nor its optical microscopy studies were disclosed. It is worth mentioning that precautions must be taken in purifying nanoparticles as the presence of unreacted liquid-crystalline material could be misleading. Nevertheless, the above report ignited the interest in designing both mesomorphic as well as mesogenic gold nanoparticles. Subsequently alkoxycyanobiphenyl-capped gold nanoparticles were synthesized, and from differential scanning calorimetry (DSC) and polarizing optical microscopy (POM) studies on them, the existence of a liquid-crystalline phase was reported.15 Since no distinct optical texture was observed the kind of mesophase was not known. However, spontaneous one-dimensional (1D) arrangement of gold nanoparticles was characterized by transmission electron microscopy (TEM) and X-ray diffraction (XRD) investigations on surfaces. The mesogens are held responsible for such an anisotropic organization of quasi-isotropic nanoparticles. Following these results Mehl and co-workers designed and synthesized gold nanoparticles fully covered with laterally attached calamitic (rod-like) liquid-crystal molecules.16 However, it failed to exhibit any LC behavior, and rather displayed a glass transition. At high level of surface coverage, the loss of mesomorphism was attributed to the lack of orientational mobility of the mesogens attached to the core, due to steric crowding. Finally, chemically stable, room-temperature nematic gold nanoparticles were obtained by the attachment of both hydrocarbon chains as well as mesogens in a two-step ligand-exchange synthetic process as shown in Scheme 1. The nature of the mesophase was confirmed by DSC and POM studies. Subsequently, Mehl and co-workers have highlighted structure–property relationships in mesomorphic gold nanoparticles.11 The two-step ligand-exchange process has now become more or less a general synthetic strategy to functionalize GNPs with calamitic, discotic as well as bent-core mesogens.
Recently Ungar and co-workers reinvestigated some of the nematic gold nanoparticles and obtained remarkable results about the 2D and 3D organization of gold nanoparticles in the orientationally ordered nematic phase.17 From grazing-incidence small-angle X-ray diffraction of aligned thin films it was revealed that gold nanoparticles self-assemble into strings and these gold strings organize in highly ordered rhombohedral and two-columnar (hexagonal and rectangular) structures, as shown in Fig. 1. The significance of these results may be appreciated in the light of the aim of liquid-crystal nanoscience to provide self-assembled supramolecular organized nanostructures by a ‘bottom-up’ approach. Moreover, it demonstrates that highly ordered superlattices of metal nanoparticles can be created by coating the particles with a laterally attached nematogenic ligand, which could be promising for self-assembled future metamaterials that function at optical frequencies.
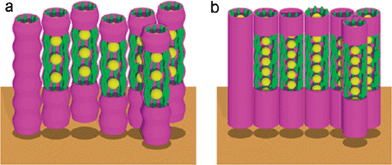 |
| Fig. 1 Schematic models of the gold string structures, (a) rhombohedral phase, (b) hexagonal columnar phase. Yellow: gold nanoparticles, green: mesogens. Reproduced with permission from ref. 17. | |
Donnio et al. have prepared mixed-monolayer protected non-mesogenic dendronized GNPs, which self-organize into a thermotropic cubic phase in the bulk or as a 2D hexagonal lattice on surfaces, which indicates a control of positional order in the system.18 Surprisingly, the system displays an unconventional ferromagnetic behavior in the bulk state. Recently, gold nanoparticles capped with a mixed-monolayer of alkanethiol and rodlike mesogenic terminal thiols with forklike end groups, were found to display liquid-crystalline behavior.19 Both smectic and columnar phases were exhibited by these GNPs depending on the end-group chain lengths. However, gold nanoparticles functionalized with a bent-core liquid-crystal molecule laterally attached to the metal cluster did not exhibit any mesomorphism. Hegmann and co-workers have also prepared gold nanoclusters protected by terminal thiol functionalized bent-core liquid-crystal molecules and studied their phase behavior and self-assembly.20 Although these materials did not show any liquid-crystalline behavior, they observed the formation of self-assembled nanoparticle arrays in solvent evaporated samples. They could also disperse these gold nanoparticles in structurally related bent-core liquid-crystal hosts, and investigated the effect of concentration of these nanoparticles on the thermal and electrooptical properties of the parent host. Interestingly, a shift of the SPR was observed, indicating host-dependent aggregation of the nanoparticles.
The columnar phases of discotic liquid crystals (DLCs) are unique nanostructures with remarkable electronic and optoelectronic properties.21–23 Owing to the quasi-one-dimensional energy and charge migration in columnar phases they have found applications in the field of one-dimensional conductors, photoconductors, light emitting diodes, photovoltaic solar cells, thin film transistors and gas sensors, etc. The incorporation of metal nanoparticles into the supramolecular order of DLCs may provide useful properties for device applications. Keeping these objectives in mind, our group has synthesized gold nanoparticles stabilized by simple alkanethiols, and dispersed them into the columnar matrix of different DLCs and studied the thermophysical properties of these novel nanocomposites.24 It was noticed that the columnar phases of DLCs could well-disperse the nanoparticles in their matrices. Unlike the calamitic nematic liquid crystals in which GNPs can be dispersed and stabilized, the discotic nematic liquid crystal could not well disperse the nanoparticles, but rather show their macroscopic phase separation. Increase in the conductivity of the columnar phase was observed owing to the presence of gold nanoparticles in the matrix. Interestingly, recent reinvestigation of hexahexylthiotriphenylene (HHTT) doped with gold nanoparticles by XRD studies provide convincing evidence for self-assembled superlattices of gold nanoparticles in the helical phase of HHTT.25 Subsequently we have designed gold nanoparticles fully covered with triphenylene discotics to see if such a functionalization could yield liquid-crystalline properties.26 Though a liquid-crystalline phase was not found, the discotic decorated gold nanoparticles could be dispersed in large quantities into the columnar phase of a related triphenylene derivative, owing to their chemical compatibility with one another. XRD studies of the bulk composites suggested random distribution of gold nanoparticles between the domain gaps of the columnar phase in a disordered fashion (Fig. 2). However, on surfaces the triphenylene functionalized nanoparticles self-assemble into beautiful hexagonal patterns believed to arise from the strong π–π interactions between the triphenylene ligands of adjacent nanoparticles. Remarkably, direct current (DC) conductivity measurements show an enhancement of electrical conductivity by more than million times upon doping under ambient conditions. Almost simultaneously but independently, Miyake et al. have reported similar self-assembly of triphenylene covered gold nanoparticles on surfaces.27 They have designed triphenylene protected nanoparticles with different spacer lengths and systematically investigated the self-assembled 1D stripes and hexagonal close packed or disordered organization of gold nanoparticles as a function of size of gold nanoparticles, alkyl chain lengths, interparticle π–π interaction and solvent hydrophilicity, as shown in Fig. 3. In addition to the dispersion of GNPs into thermotropic discotic columnar phases, we have also elaborated the dispersion of GNPs into normal lyotropic as well as inverse-hexagonal columnar phases formed by a non-ionic surfactant Triton X-100 and an anionic surfactant Aerosol-OT (AOT), respectively.28X-Ray diffraction investigations of these composites suggest that the gold nanoparticles are distributed outside the columns formed by both the surfactants. In the Triton X-100 system the gold nanoparticles seem to prefer the outer surfaces, due to large free space available in between the columns, however in the AOT system the outer surfaces of the columns are hydrophobic in nature, and hence the hydrophobic nanoparticles would naturally prefer this hydrophobic region.
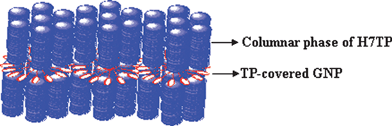 |
| Fig. 2 A schematic diagram illustrating arrangement of TP-GNPs in the inter domain spacing formed between the discotic columns. Reproduced with permission from ref. 26. | |
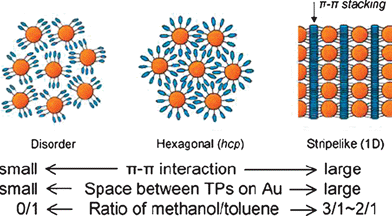 |
| Fig. 3 The possible arrangement of AuTP depends on factors investigated: π–π interaction, space around TPs on Au, and solvent hydrophilicity (ratio of methanol to toluene). Reproduced with permission from ref. 27. | |
Hegmann and co-workers have designed different kinds of gold nanoparticles (chiral, hydrophilic, mesogenic, etc.) by passivating them with different types of thiols and systematically studied the effect of these nanoparticles on the phase, alignment and electrooptic behavior of host liquid crystals with different phase structures. Optically active GNPs were prepared from a chiral aromatic thiol and chirality transfer to a non-chiral nematic LC host was established.29 It was also observed that gold nanoparticles in liquid-crystal cells form topological defects resulting in chain-like particle aggregates, separated by areas of homeotropic domains due to spatial separation of particle-rich and particle-poor domains, as shown in Fig. 4. In another contribution, electron microscopy, optical microscopy and XRD studies on dispersions of both hydrophilic and hydrophobic thiol-capped gold nanoparticles in bolaamphiphilic LCs, indicate random inclusion of nanoparticles in the LC matrix, with a concentration and monolayer-type-dependent effect on the mesophase stability of the LC host.30 Subsequently, compatibility and alignment effects of mixed-monolayer cyanobiphenyl (CB) LC-capped GNPs in CB liquid-crystal hosts has been investigated by focusing on improvement of miscibility in LC hosts.31 Surprisingly it is noticed that alkanethiol-capped GNPs are more compatible with cyanobiphenyl hosts in comparison to the nanoparticles decorated with cyanobiphenyl thiol. Although CB-GNPs induce homeotropic alignment of the host molecules between untreated glass plates, they clearly aggregate from the hosts.
 |
| Fig. 4 Simplified model for the organization of gold nanoparticles in the N-LCs (not to scale). (a) isolated stripe domain (centre) between two homeotropically aligned domains (left and right); (b) top view indicating particle-rich (stripe domain) and particle-poor areas (homeotropic domains) – LC molecules are omitted for clarity; and (c) 3D model with gold nanoclusters residing at both glass/N-LC interfaces in the homeotropic domain. Reproduced with permission from ref. 29. | |
The impact of GNPs on LCD devices has also been investigated in liquid-crystal cells. Hegmann and co-workers have beautifully demonstrated an unprecedented dual alignment mode and electrooptical response in planar nematic LC cells doped with GNPs which has potential impact on current LCD technology.32 By fine-tuning electric field, alignment, and temperature, etc. LCs doped with GNPs can be aligned and electrically reoriented either like nematic LCs with positive dielectric anisotropy in a planar cell, or as nematic LCs with a negative dielectric anisotropy in a homeotropic cell, remarkably both at lower threshold voltages than the pure nematic LC. Recently a non-volatile memory effect based on a gold nanoparticle doped deformed helix ferroelectric liquid crystal has been observed by Biradar et al.33 This observed memory effect has been attributed to electric field induced charge transfer from liquid-crystal molecules to the gold nanoparticles. Enhanced photoluminescence in GNP-doped ferroelectric liquid crystals has also been observed by the same group.34 The enhancement in the photoluminescence has been attributed to the coupling of localized SPR from metal nanoparticles with deformed helix ferroelectric liquid-crystal molecules, resulting in the increase in excitation and emission rate of the liquid-crystal molecules in the localized electromagnetic field. Similarly, surface-induced ordering of LC molecules in the vicinity of chemically functionalized GNPs have been observed to affect the localized SPR of the GNPs.35,36 Very recently, long-range reversible interactions between a pair of chemically functionalized GNPs in nematic LC phase have been reported.37 Such interactions may provide spontaneous formation of ordered nanoparticle arrays in anisotropic fluid hosts.
2.2 Other nanoparticles dispersed in liquid crystals
Similar to the dispersion and assembly of GNPs in liquid crystals, other metallic, semiconducting, inorganic, ferroelectric and ferromagnetic nanoparticles have also been dispersed to study their effect on various properties of liquid crystals. Long-range ordering of platinum nanoparticle assemblies that adopt the helical configuration of the cholesteric liquid-crystal phase has been demonstrated.38 The nanoparticles form periodic ribbons that mimic the fingerprint cholesteric texture. Interestingly the nanoparticles create a novel helical structure with larger helical pitch and the pitch of the liquid crystal acts as a simple control parameter to tune the structuring of nanoparticles.
Experimental results have shown that low concentrations of ferroelectric nanoparticles greatly enhance the physical properties of nematic LCs.2 In particular, Sn2P2S6 or BaTiO3 nanoparticles at low concentrations increase the orientational order parameter of the host LC, increase the isotropic–nematic transition temperature, and decrease the switching voltage for the Fredericksz transition. These findings are important for fundamental nanoscience because they show that nanoparticles can couple to the orientational order of macroscopic medium. They are also important for technological applications, because they provide a new opportunity to tune the properties of LCs without additional chemical synthesis. The introduction of ferroelectric nanoparticles into a chiral nematic matrix has resulted in a strong change in the mesogenic, optical and electro-optic properties of the cholesteric, keeping the optical quality and director field in the cell undisturbed.39 The most impressive impact of the nanoparticles is the decrease in the driving voltage in a cell to about half that of the pure host. It has been postulated that ferroelectric nanoparticles produce local electric fields, which polarize the liquid-crystal molecules and thereby increase the intermolecular interaction. Recently another theoretical treatment considers the orientational distribution of the nanoparticles themselves since the electric dipoles of the ferroelectric particles are likely to interact with each other forming particle chains and so on.40
The hybrid system consisting of liquid crystals and magnetic nanoparticles is known as ferronematic or ferrosmectic, depending on the phase of the liquid crystal. The idea of these ferrofluids composed from LCs doped with magnetic particles was suggested in the early 1970s by de Gennes and co-workers in order to enhance the magnetic susceptibility of liquid crystals and hence the coupling between liquid-crystal order and external magnetic fields.4 Following these theoretical predictions, some ferrofluids were successfully composed and investigated in both thermotropic and lyotropic LCs, with the size and concentration effects of the particles being the main enquiry. Very recently the impact of surface coating, and shape and size of magnetic nanoparticles on the phase behavior of classical nematic LCs has been studied.41,42 It has been observed that the shape and size of magnetic nanoparticles has significant influence on the magnetic Fredericksz transition owing to the parallel alignment of the magnetic moments of the nanoparticles with the director field of liquid crystals.
Kobayashi and co-workers have reported in a series of works on the remarkable impact of different types of inorganic nanoparticles such as Pd, Ag, MgO, SiO2 and CdS, etc. on various LCD devices.10,43 They have described frequency modulation electro-optic response with sub-millisecond response time in twisted nematic LCDs, as well as lower operating voltages and shorter response times, in different liquid-crystal display modes using these inorganic nanoparticles. The above studies clearly suggest that nanoparticles offer enormous advantages to improve upon current LCD technologies by lowering operating voltages, with higher switching speeds and better contrast ratios.
2.3 Synthesis of nanoparticles in liquid crystals
Lyotropic liquid crystals (LLCs) are long known for their role in food, cosmetic and drug industries.4 During the last two decades lyotropic liquid-crystal materials with functional properties have been attracting considerable amount of attention owing to the advantage of yielding mesoporous structures with predictable geometry and pore size. This is typically accomplished by starting from a LLC phase, and then the liquid continuous phase is simply solidified by some chemical or electrochemical reaction. The function of the LLC phase is to direct or template the organization of another material. After solidification of the secondary material, the LLC component is typically removed to allow access to the interior of these mesoporous materials. Nanostuctured films of these materials are proposed to have relevance in catalysis, batteries, fuel cells, capacitors and sensors, etc.4
The size- and shape-controlled synthesis of nanoparticles is important in present day advanced materials as almost every property within the nanometre regime are size and shape dependent. Common methods for size control employ capping agents, such as surfactants, ligands and polymers to confine the growth in the nanometre regime. Controlling shape of nanoparticles has been most successfully achieved using a template. Templates provide a constrained environment during the nanoparticles growth and thus shapes are tuned according to the templates. However procedures for shape-controlled preparation of nearly monodisperse nanoparticles are limited. Methodologies for the bulk synthesis of nanoparticles generally utilize four basic strategies: condensation from a very dilute solution or gas, solution-based surface passivation to arrest the growth of particles, the use of physical confinement on a nanometre scale to constrain growth, or nanometre scale chemical confinement to limit the amount of material available for reaction. In this context, LLCs can be utilized for the controlled synthesis of zero-, one- and two-dimensional nanoparticles.10LLCs seem to make use of the latter two strategies for the preparation of nanoparticles. The hydrophobic and hydrophilic domains of lyotropic liquid crystals have dimensions on the order of nanometres, so chemistries designed to operate exclusively in one domain of the liquid crystal are effectively constrained inside these ‘nanoreactors’.44 By controlling the liquid-crystalline phase, it is possible to control the size, shape, connectivity and dimensionality of these ‘nanoreactors’, and thereby control the size and shape of nanoparticles grown inside them. For example, a micellar cubic phase results in spherical nanoparticles, lamellar phase results in plate-like particles, inverted columnar hexagonal phase results in rod-like particles, etc.45 Moreover, these nanoreactors are easily reproducible, and can be generated in large volume for scale-up.
In a typical metal nanoparticles synthesis, a solution of the metal salt is mixed with an appropriate amount of the liquid-crystalline host such that the desired lyotropic phase is formed. Precipitation of the nanoparticles is induced chemically, electrochemically, etc., which aggregate into clusters that subsequently form a single nanostructure. Following dispersion of the lyotropic phase, the resulting nanostructures are then collected through centrifugation or filtration.10
Thermotropic LCs have also been used for the preparation and stabilization of nanoparticles analogously to LLC mediums. The use of thermotropic liquid crystals is very promising since the size- and shape-selective synthesis and self-assembly of nanoparticles can be achieved in one-pot. Ionic liquid-crystal precursors has been utilized for the synthesis of CuCl nanoplatelets though in the isotropic state, followed by reports on the preparation of leaf-like Au nanostructures and Ag nanoplatelets by electrodeposition in the lamellar phase of ionic liquid crystals.10 Recently the synthesis of gold nanoparticles has been achieved in a LC phase derived from vitamin C (ascorbic acid) based amphiphiles.46 This method exploits the advantages of ascorbic acid which is known to reduce Au(III) to Au(0) to form gold nanoparticles. Similarly in situ shape-selective synthesis and spontaneous assembly of GNPs in glass-forming LC materials have been achieved and it was observed that the LC phases were thermally stabilized as well.47 A side-chain LC network has also been employed for the size-selective synthesis of gold nanoparticles in the mesophase.48 Very recently, a sputter doping method has been introduced for preparation and stabilization of NPs in liquid crystals. It also involves a single step without having to prepare the NPs chemically.49 This technique is in principle applicable to any kind of material and LC phase, and provides a versatile route to prepare stable NPs dispersed in LCs. The electro-optic properties of a twisted nematic cell with such NPs show remarkable improvement. The above discussed reports are excellent examples of in situ synthesis and assembly of GNPs in thermotropic liquid crystals. Furthermore, the systems are free of external impurities that would have been used for the reduction and stabilization of gold nanoparticles.
3. Liquid crystals and nanorods and discs
Nanorods (NRs) are 1D solid nanostructures and are very intriguing materials for both academic interest and industrial applications. These anisotropic materials possess unique thermal, electrical, optical and mechanical properties. However, unlike the dispersion of quasi-spherical nanoparticles in liquid crystal media, there are very few reports on the dispersion of 1D nanorods in liquid-crystal mediums.10 Chen et al. have fabricated a novel nanocomposite device by embedding semiconducting CdS nanorods in liquid crystals.50 Electrically assisted magnetic anisotropy rotation has been demonstrated in another LC device doped with ferromagnetic Ni nanorods.51 Recently a newly designed colour-tunable light-emitting device based on CdSe nanorods and quantum dots dispersed in LC cells has been developed.52 It is observed that the polarization of the emission from semiconductor NRs can be controlled by an external bias and the polarization anisotropy is of large magnitude. Such devices are interesting from the viewpoint of various smart optoelectronic applications including optical switches and electric field-controlled magnetic data storage. The self-assembly of gold NRs has been studied in a lyotropic chomonic liquid-crystal material.53 These NRs display both side-by-side and end-to-end self-assembly depending on their surface charge and these assembled superstructures are stable within an extended temperature range. Interestingly, the degree of NRs aggregation can be controlled by a number of factors influencing the self-assembly of the host chromonic material, such as the concentrations and pH of the solution. Very recently, spontaneous ordering of aggregation-free plasmonic gold NRs dispersed in surfactant-based lyotropic LCs has been demonstrated.54 It is noticed that the anisotropic fluids in both columnar hexagonal and nematic LC phases impose nematic-like long-range orientational ordering of NRs with no correlation of their centers of mass. Furthermore, realignment of the NRs is attained by an external magnetic field or shearing flow effects on the host LC matrices.
Similar to organic rod-like molecules, nanorods can in principle form liquid-crystalline phases. However, since these materials are devoid of necessary flexible parts and in most of the cases their phase transition temperatures lie above their melting/decomposition temperature, they do not seem to exhibit thermotropic LC phases in the neat state. Nevertheless if these anisotropic objects are functionalized with suitable organic moieties, which provide sufficient mobility while retaining their orientational order, these organic–inorganic hybrids tend to exhibit thermotropic liquid-crystalline phases. Kanie et al. have designed and synthesized thermotropic LCs, by surface coating of needle-shaped TiO2 as well as α-Fe2O3 particles with mesomorphic rod-like molecules, which furnish nematic and cubic phases.55,56 While the surface of TiO2 has been passivated by amino-functionalized mesogens, that of α-Fe2O3 is by phosphate-functionalized mesogens.
Since nanorods possess the necessary geometric anisotropy, they can form lyotropic liquid-crystalline phases in suitable solvents, which imparts them with mobility both in their pure form as well as upon surface-functionalization with organic molecules. Historically, lyotropic liquid-crystalline phases have been observed and well understood for various anisotropic rigid-rod objects, such as, tobacco mosaic virus, colloidal rods and DNA, etc.4 The basis for this phenomenon is the classical Onsager argument for liquid-crystal phase formation in concentrated suspensions of rigid rods. If the aspect ratio of the rods and their concentration are both large enough, the free energy of the system is reduced by forming LC phases. Based on this principle various inorganic NRs have been found to exhibit lyotropic LC phases, the so-called inorganic liquid crystals. Lyotropic LC phases of CdSe semiconductor nanorods have been obtained by Alivisato and co-workers in concentrated solutions.57 Liquid-crystalline phases of high aspect ratio gold NRs in concentrated solutions have been studied by Jana and others.58 The LC phases are stable up to very high temperature and they exhibit concentration-dependent orientational order. Recently, liquid-crystalline phases from polymer-functionalized semiconducting nanorods such as TiO2, SnO2, ZnO and CdTe have been systematically studied by Zentel and co-workers.59 These hairy-rod organic–inorganic hybrids display LC phases at suitable concentrations in organic solvents as well as in oligomeric and polymeric matrices and undergo field-induced reorientation. The combination of liquid-crystalline nanorod ordering with electric field induced reorientation could be a promising approach for their optoelectronic applications.
Another anisotropic shape exhibiting liquid crystallinity is the nanodisk. Like the liquid-crystalline ordering of collection of rod-shaped nanocrystals in concentrated dispersions, collection of disk-shaped nanocrystals should also exhibit orientational order with sufficient concentration and narrow size and shape distributions. Examples of disc-like objects showing mesophase behavior include films of sub-micrometer size colloidal discs of Al(OH)3 (gibbsite) and Ni(OH)2, forming nematic, smectic and columnar type mesophases. Recently, sterically stabilized colloidal CuS nanodiscs have been shown to form self-assembled columnar structures with hexagonal close packing.60 Moreover several examples have appeared in the literature where electron microscopy images of evaporated films of nanodiscs have displayed long-range orientational ordering.10
4.1 CNTs in thermotropic liquid crystals
Carbon nanotubes are the only other nanomaterials after GNPs which have been studied in greater detail with liquid crystals owing to their elongated molecular structure and their catalogue of unique properties.61–63 CNTs, the fourth allotrope of carbon, after diamond, graphite and fullerene, are one-dimensional, well ordered all-carbon hollow cylinders of graphite with a high aspect ratio. The combination of superlative mechanical, thermal and electronic properties displayed by CNTs make them ideal for a wide range of applications, such as conductive and high-strength composites, energy-storage and energy-conversion devices, field emitters, transistors, sensors, gas storage media, and molecular wires. Despite the extraordinary promise of CNTs, their realistic application as 1D semiconductors has been limited because of difficulties in aligning them in the required directions. Well-arrayed CNTs are highly desirable since the alignment of CNTs play a critical role in the properties of nanotube-based devices and materials. However, processing materials with well-controlled CNT alignment remains a challenge. Recently, there has been growing interest in the field of dispersion of CNTs in both thermotropic and lyotropic LC phases, besides the mesophase behavior of CNTs themselves, and preparation of CNTs from discotic liquid crystals.61–63Mixing CNTs into thermotropic LC hosts has become one of the attempted approaches to modify the physical properties of LCs as well as the homogeneous alignment of CNTs in different LC matrices for CNT-based device applications.62 Thus various physical parameters important for LCD applications such as the elastic constants of deformation, dielectric and optical anisotropies, conductivity and rotational viscosity have been evaluated in LC cells doped with CNTs. It is encouraging that LC cells with various display modes exhibit improved electrooptical characteristics such as low driving voltage, faster refresh rates, high contrast ratios, etc. On the other hand, well-dispersed CNTs in LC matrices will in general align with their long axes along the LC director (Fig. 5a) to minimize distortions of the LC director field, and thus the free energy of the system.63 Dispersion of CNTs in LCs can provide a cheap, simple, versatile and effective means of controlling nanotube orientation on the macroscopic scale with no restrictions on nanotube type. The advantages of thermotropic LCs in this context are twofold: one, using existing alignment techniques, well developed for LCD industry, the ground state of the LC and hence of the dispersed CNTs can easily be determined on macroscopic scale; second, the easy and rapid changing the director field by means of electric or magnetic fields can lead to switches based on reorientation of CNTs. Dierking et al. have studied the alignment and reorientation of doped carbon nanotubes in LC cells, under both electric and magnetic fields, along with the change in the conductivity of the LC cells upon reorientation of the CNTs with the liquid-crystal director field.64 Moreover CNTs have been aligned in polymerizable LC monomers and subsequently polymerized to fix the orientations of the mesogens and CNTs to achieve composites with enhanced as well as novel functionalities.61 The above discussions seem to support the idea of both the goals of CNT alignment and liquid-crystal property enhancement by doping CNTs in calamitic nematic liquid crystals.
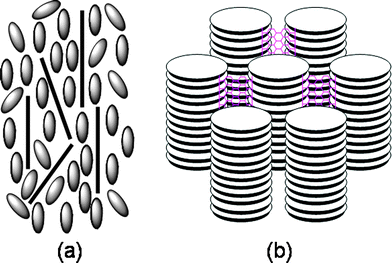 |
| Fig. 5 Alignment of CNTs in calamitic nematic liquid crystals (a) and in discotic hexagonal columnar phase (b). | |
The insertion (dispersion) of CNTs in the supramolecular order of discotic liquid-crystalline monomers and polymers may lead to novel semiconducting materials useful for device applications. Since the ordered columnar phases, in contrast to isotropic and nematic phases, can offer wider opportunity for processing materials with oriented nanotubes, a critical factor considering the strongly anisotropic properties of CNTs. Furthermore, there are many similarities between CNTs and discotic liquid crystals; first, both CNTs and DLCs are anisotropic materials. Second, both the materials posses self-assembling properties and often form hexagonal aggregates. Third, and more important, is the 1D conductivity properties of both CNTs and DLCs. Conductivity along the columnar axis of DLCs is several orders of magnitude greater than perpendicular to it, an anisotropy also found in CNTs, i.e. the conductivity along the tube axis is much higher than across it. Therefore, both the materials can be considered as “molecular wires”. With this in mind, we have dispersed functionalized CNTs into the columnar matrix of liquid-crystalline discotic monomers and polymers.65,66 Initially, we attempted to disperse acid-purified single walled nanotubes (SWNTs) into the columnar matrix of hexabutyloxy triphenylene (H4TP) in minute quantities but we noticed that the purified CNTs formed aggregates in the columnar phase which can be clearly observed under POM. Then we turned towards chemical functionalization of CNTs to make them more compatible with the discotic liquid-crystal matrix. Thus acid-purified carboxylic acid-bearing CNTs were chemically functionalized at the ends and sidewalls by a hydroxyl functionalized H4TP derivative through an ester functionality. These discotic functionalized nanotubes (f-SWNTs) were highly soluble in common organic solvents. The formation of f-SWNTs was confirmed by spectral, microscopic and thermogravimetric analysis (TGA). However, the triphenylene capped SWNTs were found to be non-liquid-crystalline in nature. Subsequently composites of f-SWNTs and hexabutyloxy triphenylene were prepared and their LC properties were investigated by POM, DSC and XRD studies. The composites display the classical texture of columnar phases on cooling from the isotropic liquid state and no aggregations of CNTs are observed. The insertion of CNTs decreases the mesophase to isotropic transition temperatures significantly but crystal-to-mesophase transitions are little affected. The X-ray diffraction pattern of pure H4TP was compared with the 10 wt% composite. A significant shift in the first-order reflection toward larger d-spacing clearly indicates the insertion of CNTs in the supramolecular order of the columnar phase. It seems the functionalized CNTs occupy the space between the disc columns and are oriented parallel to the columnar axis (Fig. 5b).65 We have also studied the dispersion of commercial octadecylamine (ODA) functionalized CNTs in the columnar phases of triphenylene and rufigallol based discotic monomers and polymers, which were found to exhibit mesomorphism starting from room temperature.66 Unlike discotic functionalized CNTs, here up to a maximum of 2 wt% of CNTs can be homogeneously dispersed in the LC phases. Beyond this percentage, small aggregates of CNTs can be observed under a microscope. This study reflects the importance of chemical compatibility of functionalized CNTs with the host columnar phases. Therefore chemical functionalization of CNTs with mesogens should yield stable dispersions with high concentration of CNTs, of course with some compromise on the electronic properties of the CNTs. The mesophase behaviors of the above dispersions reveal that there is no significant change in the d-spacings of the composites compared to the pristine materials. However, the high birefringence of the composites compared to the host materials is indicative of CNT alignment along the columnar axis in the liquid-crystalline matrix. These room-temperature liquid-crystalline nanocomposites, with broad mesophase ranges and complimentary electronic properties, may be important for many device applications since the aligned CNTs can be reoriented in the desired direction using established DLC alignment techniques.
Recent years are witnessing novel approaches of CNT functionalization with mesomorphic oligomers and polymers and their dispersion into smectic and polymeric liquid crystals. Smectic liquid-crystalline conjugated oligomers are found to form stable supramolecular complexes with the CNTs imparting excellent solubility in organic solvents.67 The addition of excess of the LC oligomers into dispersed solutions induces the formation of precipitates and the precipitates exhibit thermotropic LC phases. Self-assembled plate-like structures of CNTs have been prepared by noncovalent hybridization with a smectic liquid crystal.68 This facile approach to the processing of CNTs is significant for nanotube-based composite materials. Nematic elastomers with aligned CNTs exhibit reversible infrared and electromechanical actuation.61 Very recently it has been shown that pyrene end-capped flexible main-chain LC polymers can effectively assist the dispersion of CNTs in organic solvents. The enhanced solubility of the thus obtained conjugates in liquid-crystalline elastomer hosts has led to multi-stimuli actuation behavior in the LC elastomers.69 It seems the combination of the fourth form of carbon in the fourth state of matter would provide some more fascinating results in the near future.
4.2 CNTs in lyotropic liquid crystals
There are relatively less examples of inclusion of CNTs into lyotropic LCs as compared to their thermotropic counterparts.61–63 However, as lyotropic LCs are water based and common in biological and living systems, it could be very interesting to use CNT–lyotropic LC mixtures for biotechnological applications. Unfortunately, recent toxicological studies of CNTs indicate that CNTs could be as harmful as asbestos and, therefore, extensive studies are required before their in vivo biotechnological application. In contrast to thermotropic LC-CNT composites, the lyotropic LC-CNT composites can be prepared in two different ways. One can start by preparing an isotropic low-surfactant-concentration suspension of CNTs and then that can be made liquid crystalline by adding more surfactant, or CNTs can be added to an already prepared LLC sample. The insertion of CNTs into LLC phases made from non-ionic and ionic-liquid surfactants was observed to increase both the d-spacing and viscosity of the hexagonal columnar phase with increase of carbon nanotube concentrations.61 Lagerwall et al. have aligned carbon nanotubes in the lyotropic nematic phases formed by both rod-shaped and disc-shaped micelles as shown in Fig. 6.70 Later they have combined cationic and anionic surfactants to form a LC colloidal suspension of CNTs in the columnar hexagonal phase from which filaments with highly aligned CNTs can be drawn and deposited in selected directions on surfaces.62 Because of the high viscosity of the LLC phases, the incorporation of CNTs is usually difficult, and requires rigorous sonication combined with a heating process. Therefore approaches to create spontaneous phase transition from isotropic CNTs dispersions to lyotropic phases are highly desired. Hence CNT/LLC hybrids have been fabricated by a phase separation method induced by different polyelectrolytes.71 Several factors that can influence the properties of these novel composites were also examined including concentration of the surfactants and polyelectrolytes and temperature. Recently, polymeric CNT–LC composites were prepared from lyotropic LCs using CNTs and a polymerizable surfactant.72 The nanotubes were dispersed in the LLC phase of the surfactant and the dispersions were subsequently photopolymerized to give liquid-crystalline polymeric composites with oriented CNTs. Until recently there were no reports of dispersions of CNTs in the lyotropic lamellar phase.73 Hydrophilically functionalized CNTs are now reported to be selectively distributed in the polar domains of a lyotropic lamellar phase prepared from an aqueous ternary mixture of a block copolymer in p-xylene.
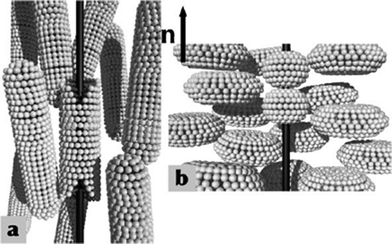 |
| Fig. 6 Schematic illustrations of the mechanism of CNT alignment in a lyotropic nematic LC host, for the case of rod- (a) and disc-micelle-type (b) adsorption, respectively, of surfactant molecules on the CNT surface. The CNT is drawn as a vertical black rod in the centre of each picture, only partially covered by surfactant molecules for clarity. In reality, it is completely encapsulated. Reproduced with permission from ref. 70. | |
4.3 Liquid-crystal phases formed by CNTs
Carbon nanotubes can be viewed as highly anisometric rigid-rod-like particles. Like other anisotropic and 1D molecules, CNTs exhibit lyotropic mesomorphism upon suitable functionalization.74,75 Several experimental contributions have been reported, all of them describing different ways of making liquid-crystalline phases of carbon nanotubes in aqueous solutions. These systems form nematic phases via acid functionalization, acid protonation, gradual evaporation of films or absorption of amphiphilic molecules and polymers on CNTs surfaces. Protonation of CNTs sidewalls in super-acids eliminates van der Waals interactions and promote the dispersion process, and at higher concentrations CNTs form nematic phases. A promising approach reported to obtain LC phases from unmodified CNTs, is by non-covalent wrapping of biopolymers such as denatured DNA and hyaluronic acid to CNTs, which exhibit the nematic mesophase but preserves the intrinsic properties of CNTs.63 The mesophase behavior of CNTs in aqueous solutions has been exploited to separate CNTs based on their length and so facilitate fabrication of highly orientated CNT arrays for thin film transistors etc.75 However, recent works have focused on the formation of LLC phases of CNTs in organic solvents by noncovalent functionalization with synthetic polymers. Consequently pyrene-functionalized poly(methyl methacrylate) (PMMA) chains were found to be highly efficient to solubilize and disentangle multi-walled CNTs that self-organizes as an LC phase in an ethylene glycol oligomer matrix.61 Similarly conjugated polymer-functionalized CNTs have been observed to exhibit liquid-crystalline phase in o-dichlorobenzene organic solvent.76 Interestingly, CNTs can be easily aligned in large domains on glass surfaces by simple shearing of a drop of the LC polymer/CNT dispersion. Furthermore, uniform alignment of a nematic liquid-crystal material was observed on the above shear aligned films. This result indicates that such films could potentially act as conducting alignment layers for liquid crystals in contrast to the very commonly used insulating rubbed polyimide layers on conducting electrodes. While nematic phases have been experimentally reported, no evidence of smectic phases of CNTs has ever been observed. The observation of a smectic phase of rods necessitates a polydispersity as narrow as possible; however, the actual polydispersity of carbon nanotubes does not seem to allow the formation of lamellar phases. However, the LC phase behavior of CNTs seems to validate and further strengthens the basic understanding of LC phase formation in 1D rod-like objects.
4.4 Preparation of CNTs from discotic liquid crystals
Preparation of carbon nanotubes typically requires high temperature or high pressure. It is well known that in these cases the reactions are chemically complicated and difficult to control. Another serious problem is the separation of the catalysts from the nanotube product as significant amount of the catalyst particles are encapsulated into the nanotubes. Moreover, the orientation of the graphene layers in the nanotubes is important from their application point of view. To form ordered graphite nanostructures without metal catalysts and with desired graphene layer orientations, one possible approach is carbonization within a discotic columnar mesophase. From this point of view, the columnar super-structures of discotic liquid crystals are possible precursors as the molecules possess a nanographene subunit as the aromatic core. Upon carbonization under a controlled heating process the preorganized ordered columnar superstructures can be converted into nanotubes. As a ‘proof-of-the-principle’ CNTs have successfully been produced from both thermotropic as well as lyotropic discotic liquid crystals by pyrolysis in the mesophase.61 The temperatures required are much lower than the normally used graphitization temperatures. Discotic molecules can also be converted into 1D carbon nanotubes with well-controlled graphene layer orientation under a template directed solid-state pyrolysis process. Recently the template method has been used to fabricate uniform CNTs by pyrolysis of the graphitic molecule hexabenzocoronene (HBC) in porous alumina membranes. Upon processing graphene molecules aligned along the channels and kept ordered under slow heating procedures, uniform CNTs with ordered graphene orientations were obtained in quantitative yield after template removal.77 Interestingly, the orientation of the graphene layers is perpendicular to the tube axis due to the preorganization of the disc-like molecules in the template. This is in sharp contrast to the normal CNTs, in which the graphene layers are parallel to the tube axes. Furthermore, graphitic nanotubes can be assembled in a suitable solvent from amphiphilic discotic molecules by an appropriate choice and balance of the hydrophilic and hydrophobic parts. Hill et al. have demonstrated a unique approach to self-assembled graphitic nanotubes from an amphiphilic hexabenzocoronene.78 In sharp contrast to the above pyrolysis prepared nanotubes, their nanotubes consist of graphitic walls formed from numerous molecular graphene sheets stacked parallel to the longer axis of the tube. The proposed structure of the nanotubes consists of helically rolled-up bilayer tapes composed of π-stacked HBC units, where the inner and outer HBC layers are connected by interdigitation of the hydrophobic alkyl chains, while the hydrophilic ethylene oxide chains are located on both sides of the tubular wall. The π-stacked HBC units provide a charge carrier transport pathway and the nanotubes have been proposed as potential 1D helical conductors. Another remarkable aspect of these nanotubes is the existence of a thermotropic discotic columnar mesophase upon heating these graphitic nanotubes under neat conditions.78
5. Liquid crystals and [60]fullerenes
[60]Fullerene (C60) has attracted considerable attention in the field of supramolecular chemistry. The development of materials that display the outstanding photophysical and electrochemical properties of C60 has driven such studies.79 Thus considerable efforts have been devoted to the development of donor–acceptor dyads for which photoinduced electron and/or energy transfer could be achieved since the results could potentially open the doors for the elaboration of novel artificial photosynthetic systems, molecular electronic devices and photovoltaic cells. Recently, C60 has also been used for dispersion in thermotropic liquid crystals in a hope to improve the physical properties. C60 as such has been dispersed in both calamitic nematic and discotic columnar liquid crystals to study its effect on phase behavior.79 While for the nematic liquid crystal it does not show any discernable change of the transition temperature, dendritic discotic liquid crystals form supramolecular complexes with C60, thereby modifying the liquid-crystal phase structure as well as enhancing the isotropic phase transition temperature substantially. The effect of C60 on the electro-optical properties of liquid-crystal display cells has also been investigated in a couple of reports. However it has not been very extensively studied as a dopant in liquid crystals probably owing to its isotropic geometry and small size.79 Interestingly liquid-crystalline derivatives of this isotropic species has been designed and studied to simultaneously benefit from the photophysical and electrochemical properties of C60 and the self-assembling and processible properties of liquid crystals. These self-organized structures are expected to open up new optoelectronic applications and the study of the physicochemical properties within the liquid-crystalline medium is worthwhile.79 There are basically two approaches pursued for the covalent functionalization of C60 to yield liquid-crystalline materials; the first approach uses potential mesogenic units while in the second approach non-mesogens have been used. Various calamitic, discotic and dendritic mesogen units have been covalently grafted to the fullerene units which exhibit different mesomorphism. Alkoxycyanobiphenyl-substituted fullerenes exhibit interesting lamellar mesophase structures wherein the fullerene units are phase segregated in a sublayer separated from the interdigitated cyanobiphenyl sublayer.79 The use of optically active mesomorphic groups leads to the formation of chiral nematic phase. An optically active liquid-crystalline hexa-adduct of C60 has been designed and synthesized which displays supramolecular helical organization.80 While considering the selection process for the formation of helical organization of these molecules, it turns out that these molecules may be considered as chiral nanoparticles having poles at the top and bottom of the structure, i.e. a molecular boojom as shown in Fig. 7. This would arise from spiralling of the local director of the chiral mesogens around the C60 core. These chiral supermolecular nanoparticles would transmit their local organization through amplification to adjacent supermolecules resulting in the formation of a helical supramolecular structure. Discotic mesogens such as triphenylene and phthalocyanines have also been attached to the fullerene units, however these conjugates fail to exhibit any mesomorphism.21 Nevertheless, the discotic functionalized fullerenes have been mixed with structurally related discotic liquid-crystal hosts forming columnar phase and has provided some stimulating results. A phthalocyanine functionalized fullerene derivative when mixed with a columnar liquid-crystalline phthalocyanine did not disturb the phase behavior of the host as observed by optical microscopy and calorimetric studies, however, on the basis of X-ray diffraction studies of the blends, an alternating stacking of the host molecules and the dyads in the columns has been proposed.81 Bushby et al. have observed a two-dimensional hexagonal superlattice (Fig. 8) from the ordering of fullerenes within the hexagonal columnar liquid-crystal matrix formed by a fullerene derivative and a hexaphenyl hexaazatriphenylene discotic.82 It is believed that to maximize fullerene–fullerene contact, the fullerenes form chains which wrap around the central column in every group of seven columns. This observation points to the self-assembly of nanoparticles in the liquid-crystalline phase when they are suitably functionalized and proper hosts are chosen judiciously.
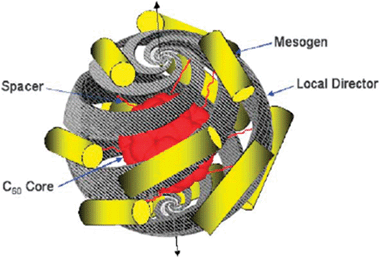 |
| Fig. 7 Proposed helical structure of supermolecular nanoparticles. Reproduced with permission from ref. 80. | |
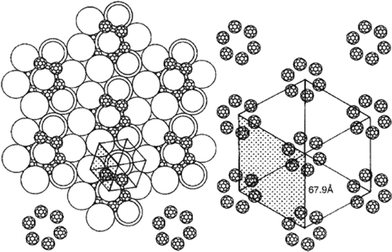 |
| Fig. 8 Schematic drawing of the proposed structure of self-assembled fullerenes in a hexagonal columnar phase forming a superlattice. Reproduced with permission from ref. 82. | |
Recently columnar liquid crystals with polar columns have been obtained by attaching five aromatic groups with aliphatic chains to one pentagon of a C60 fullerene molecule (Fig. 9). The attachment of five aromatic groups to one pentagon of C60 yields conical molecules.83 These ‘nano-shuttlecock’ molecules with conical cavities stack into columnar assemblies and exhibit thermotropic hexagonal columnar phase. Interestingly, lyotropic nematic columnar and hexagonal columnar phases are also observed at lower and higher concentrations, when dodecane is used as the solvent. Subsequently, to make a larger cavity that has a greater loading capacity, a cup-shaped cavity is created on C60 with the aid of a silicon connection.84 The larger cavity of these molecules can comfortably accommodate a second fullerene molecule. Thus it leads to the induction of a thermally more stable columnar phase which is reflected in the columnar–isotropic phase transition temperature. Furthermore, decaaryl-functionalized bow-tie-shaped fullerenes (Fig. 10) have been recently designed and synthesized and observed to exhibit a liquid-crystalline smectic phase.85 Phase behavior comparison of the pentaaryl- and decaaryl-substituted fullerenes clearly suggests the importance of molecular design to engineer their supramolecular organizations and it is possible to obtain liquid crystals from isotropic nano-objects by suitable chemical functionalization.
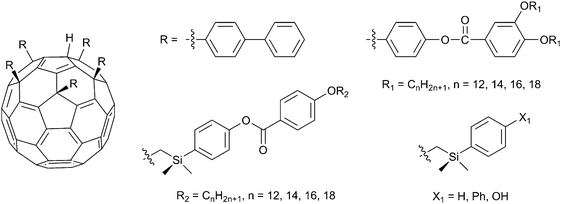 |
| Fig. 9 Penta-aryl substituted C60 fullerenes exhibiting liquid-crystalline phases. | |
![Structure of bow-tie-shaped decaaryl[60]fullerene mesogens and the proposed smectic organization of 1 based on XRD data. Reproduced with permission from ref. 85.](/image/article/2011/CS/b901793n/b901793n-f10.gif) |
| Fig. 10 Structure of bow-tie-shaped decaaryl[60]fullerene mesogens and the proposed smectic organization of 1 based on XRD data. Reproduced with permission from ref. 85. | |
6. Liquid-crystal nanocolloids
Along with the ‘nano-revolution’ the past few years have seen a steady increase in the use of colloidal particles in liquid crystals particularly in the nematic phase. The so-called liquid-crystal nanocolloids attract special attention due to the fact that large particles, of the order of microns, disturb the order of the liquid-crystal phase, introducing strain and defects.10 Dispersed colloidal particles disrupt the nematic order and minimization of the elastic energy leads to the formation of anisotropic colloidal structures. Sufficiently large particles, depending on the strength and direction of nematic anchoring on the particle surface can form various types of topological defects in nematic colloids such as Saturn rings, hyperbolic hedgehog and boojums, in agreement with theoretical considerations. Thus the formation of defects and the formation of linear particle aggregates as a result thereof have been most intensively studied in nematic liquid crystals. However, nanoscale particles (10–100 nm) when dispersed in LC medium cause little or no deformation owing to their comparable size relative to the liquid-crystal molecules. Towards this end, liquid-crystal nanoscience seems to draw inspiration from liquid-crystal nanocolloids since nematic colloids not only self-assemble in 1D but also tend to form 2D-ordered structures, which is an indication that large-area crystalline structures could be self-assembled using appropriate techniques.
The introduction of anisotropic interactions is necessary to increase the complexity and diversity of self-assembled colloidal architectures. It has been observed that colloidal droplets self-organize into highly ordered arrays of monodisperse chains.86 The size and spatial organization of the droplets are controlled by the orientational elasticity of the LC phase and the defects caused by droplets exceeding a critical size. Similarly two-dimensional nematic colloidal crystals self-assembled by topological defects has also been observed in micrometre sized silica spheres in nematic liquid crystals.87 The two-dimensional colloidal crystal structure is a consequence of side-by-side organization of chain-like particle aggregates. In addition to topological defect induced self-assembly of spherical microparticles, recently particle-shape controlled colloidal interactions has been noticed by dispersing anisotropic polygonal platelets in nematic liquid crystals.88 It has been demonstrated that the colloidal interactions and self-assembly in anisotropic nematic fluids can be effectively tailored via control over particles shape. It appears that the real goal in the study of nematic LC colloids, is to fabricate 3D colloidal crystals and more complex structures for applications in photonics and other areas, which is one of the main objectives of liquid-crystal nanoscience as well.
7. Conclusions and outlook
Liquid-crystal nanoscience is a young emerging field of liquid-crystal research and has received increasing attention over recent years. The presence of zero-, one- and two-dimensional nanostructures dispersed into thermotropic liquid crystals have resulted in striking differences in the optical and electrooptical behaviors. Liquid-crystalline nanomaterials have been successfully designed and investigated, in addition to synthesis and self-assembly of nanoparticles in the liquid-crystalline medium itself. Liquid-crystal nanoscience seems to broadens the horizon of liquid-crystal science by the use of topical sophisticated characterization techniques, provokes the development of new theories and stimulates novel scientific endeavors, etc. It is understood that nanoscience is hunting for simple methods to organize nanomaterials by means of ‘bottom-up’ processes. Self-assembly processes are the method of choice to achieve this goal, focusing on the tailoring of supramolecular and interfacial interactions, and liquid crystals seem to be the promising supportive medium. ‘Active’ nanoparticles assemblies in liquid-crystal anisotropic mediums could provide the next generation of advanced functional and/or engineered materials and may find applications in nanocomposite-based devices. The recent observation of spontaneous liquid-crystalline phase formation in concentrated graphene solutions would definitely add an extra impetus to the LC nanoscience field.89 Some nanomaterials such as GNPs and CNTs have enjoyed the golden opportunity in LC nanoscience so far but better opportunities may be in store for other nanoparticles which can only be realized as the field moves forward and makes more significant advances. Nonetheless, many interesting effects reported in the literature are not yet completely understood. Given the century-old history of liquid crystals, liquid crystal nanoscience is a new-born field and is envisaged to emerge as an interesting field at its own right.
References
- T. Kato, N. Mizoshita and K. Kishimoto, Angew. Chem., Int. Ed., 2006, 45, 38–68 CrossRef CAS
.
- J. W. Goodby, I. M. Saez, S. J. Cowling, V. Gortz, M. Draper, A. W. Hall, S. Sia, G. Cosquer, S.-E. Lee and E. P. Raynes, Angew. Chem., Int. Ed., 2008, 47, 2754–2787 CrossRef CAS
.
- C. Tschierske, Chem. Soc. Rev., 2007, 36, 1930–1970 RSC
.
-
Handbook of Liquid Crystals, ed. D. Demus, J. Goodby, G. W. Gray, H.-W. Spiess and V. Vill, Wiley-VCH, Weinheim, 1998, vol. 1–3 Search PubMed
.
- D. Tanner, J. A. Fitzgerald and B. R. Philips, Angew. Chem., Int. Ed. Engl., 1989, 28, 649–654 CrossRef
.
- I. W. Hamley, Soft Matter, 2010, 6, 1863–1871 RSC
, and references therein.
- M. Nakata, G. Zanchetta, B. D. Chapman, C. D. Jones, J. O. Cross, R. Pindak, T. Bellini and N. A. Clark, Science, 2007, 318, 1276–1279 CrossRef CAS
, and references therein.
- S. J. Woltman, G. D. Jay and G. P. Crawford, Nat. Mater., 2007, 6, 929–938 CrossRef CAS
.
- S. Kumar, Synth. React. Inorg., Met.-Org., Nano-Met. Chem., 2007, 37, 327–331 Search PubMed
.
- T. Hegmann, H. Qi and V. M. Marx, J. Inorg. Organomet. Polym. Mater., 2007, 17, 483–508 CrossRef
.
- L. Cseh and G. H. Mehl, J. Mater. Chem., 2007, 17, 311–315 RSC
.
- H. Qi and T. Hegmann, J. Mater. Chem., 2008, 18, 3288–3294 RSC
.
- M. Brust, M. Walker, D. Bethell, D. J. Schiffrin and R. Whyman, J. Chem. Soc., Chem. Commun., 1994, 801–802 RSC
.
- N. Kanayama, O. Tsutsumi, A. Kanazawa and T. Ikeda, Chem. Commun., 2001, 2640–2641 RSC
.
- I. In, Y.-W. Un, Y. J. Kim and S. Y. Kim, Chem. Commun., 2005, 800–801 RSC
.
- L. Cseh and G. H. Mehl, J. Am. Chem. Soc., 2006, 128, 13376–13377 CrossRef CAS
.
- X. Zeng, F. Liu, A. G. Fowler, G. Ungar, L. Cseh, G. H. Mehl and J. E. Macdonald, Adv. Mater., 2009, 21, 1746–1750 CrossRef CAS
.
- B. Donnio, P. Garcia-Vazquez, J.-L. Gallani, D. Guillon and E. Terazzi, Adv. Mater., 2007, 19, 3534–3539 CrossRef CAS
.
- M. Wojcik, W. Lewandowski, J. Matraszek, J. Mieczkowski, J. Borysiuk, D. Pociecha and E. Gorecka, Angew. Chem., Int. Ed., 2009, 48, 5167–5169 CrossRef CAS
.
- V. M. Marx, H. Girgis, P. A. Heiney and T. Hegmann, J. Mater. Chem., 2008, 18, 2983–2994 RSC
.
- S. Kumar, Chem. Soc. Rev., 2006, 35, 83–109 RSC
.
-
S. Kumar, Chemistry of Discotic Liquid Crystals: From Monomers to Polymers, CRC Press, Boca Raton, FL, 2010 Search PubMed
.
- H. K. Bisoyi and S. Kumar, Chem. Soc. Rev., 2010, 39, 264–285 RSC
.
- S. Kumar and V. Lakshminarayanan, Chem. Commun., 2004, 1600–1601 RSC
.
- D. Vijayaraghavan and S. Kumar, Mol. Cryst. Liq. Cryst., 2009, 508, 101–114
.
- S. Kumar, S. K. Pal, P. S. Kumar and V. Lakshminarayanan, Soft Matter, 2007, 3, 896–900 RSC
.
- Z. Shen, M. Yamada and M. Miyake, J. Am. Chem. Soc., 2007, 129, 14271–14280 CrossRef CAS
.
- P. S. Kumar, S. K. Pal, S. Kumar and V. Lakshminarayanan, Langmuir, 2007, 23, 3445–3449 CrossRef CAS
.
- H. Qi and T. Hegmann, J. Mater. Chem., 2006, 16, 4197–4205 RSC
.
- H. Qi, A. Lepp, P. A. Heiney and T. Hegmann, J. Mater. Chem., 2007, 17, 2139–2144 RSC
.
- H. Qi, B. Kinkead, V. M. Marx, H. Zhang and T. Hegmann, ChemPhysChem, 2009, 10, 1211–1218 CrossRef CAS
.
- H. Qi, B. Kinkead and T. Hegmann, Adv. Funct. Mater., 2008, 18, 212–221 CrossRef CAS
.
- J. Prakash, A. Choudhary, A. Kumar, D. S. Mehta and A. M. Biradar, Appl. Phys. Lett., 2008, 93, 112904 CrossRef
.
- A. Kumar, J. Prakash, D. S. Mehta, A. M. Biradar and W. Haase, Appl. Phys. Lett., 2009, 95, 02317
.
- S. Khatua, P. Manna, W.-S. Chang, A. Tcherniak, E. Friedlander, E. R. Zubarev and S. Link, J. Phys. Chem. C, 2010, 114, 7251–7257 CrossRef CAS
.
- G. M. Koenig Jr, M.-V. Meli, J.-S. Park, J. J. de Pablo and N. L. Abbott, Chem. Mater., 2007, 19, 1053–1061 CrossRef CAS
.
- G. M. Koenig Jr, J. J. de Palbo and N. L. Abbott, Langmuir, 2009, 25, 13318–13321 CrossRef CAS
.
- M. Mitov, C. Portet, C. Bourgerette, E. Snoeck and M. Verelst, Nat. Mater., 2002, 1, 229–231 CrossRef CAS
.
- O. Kurochkin, O. Buchnev, A. Iijin, S. K. Park, S. B. Kwon, O. Grabar and Y. Reznikov, J. Opt. A: Pure Appl. Opt., 2009, 11, 024003 CrossRef
.
- L. M. Lopatina and J. V. Selinger, Phys. Rev. Lett., 2009, 102, 197802 CrossRef
.
- G. Cordoyiannis, L. K. Kurihara, L. J. Martnez-Miranda, C. Glorieux and J. Thoen, Phys. Rev. E: Stat., Nonlinear, Soft Matter Phys., 2009, 79, 011702 CrossRef
.
- P. Kopcansky, N. Tomasovicova, M. Koneracka, V. Zavisova, M. Timko, A. Dzarova, A. Sprincova, N. Eber, K. Fodor-Csorba, T. Toth-Katona, A. Vajda and J. Jadzyn, Phys. Rev. E: Stat., Nonlinear, Soft Matter Phys., 2008, 78, 011702 CrossRef
.
- F. Haraguchi, K. Inoue, N. Toshima, S. Kobayashi and K. Takatoh, Jpn. J. Appl. Phys., 2007, 46, L796–L797 CrossRef CAS
, and references therein.
- T. M. Dellinger and P. V. Braun, Chem. Mater., 2004, 16, 2201–2207 CrossRef CAS
.
- G. N. Karanikolos, P. Alexanderidis, R. Mallory, A. Petrou and T. J. Mountziaris, Nanotechnology, 2005, 16, 2372–2380 CrossRef CAS
.
- P. K. Vemula, U. Aslam, V. A. Mallia and G. John, Chem. Mater., 2007, 19, 138–140 CrossRef CAS
.
- V. A. Mallia, P. K. Vemula, G. John, A. Kumar and P. M. Ajayan, Angew. Chem., Int. Ed., 2007, 46, 3269–3274 CrossRef CAS
.
- I. Gascon, J.-D. Marty, T. Gharsa and C. Mingotaud, Chem. Mater., 2005, 17, 5228–5230 CrossRef CAS
.
- H. Yoshida, K. Kawamoto, H. Kubo, T. Tsuda, A. Fujii, S. Kuwabata and M. Ozaki, Adv. Mater., 2010, 22, 622–626 CrossRef CAS
.
- K.-J. Wu, K.-C. Chu, C.-Y. Chao, Y.-F. Chen, C.-W. Lai, C.-C. Kang, C.-Y. Chen and P.-T. Chou, Nano Lett., 2007, 7, 1908–1913 CrossRef CAS
.
- T.-J. Lin, C.-C. Chen, W. Lee, S. Cheng and Y.-F. Chen, Appl. Phys. Lett., 2008, 93, 013108 CrossRef
.
- H.-S. Chen, C.-W. Chen, C.-H. Wang, F.-C. Chu, C.-Y. Chao, C.-C. Kang, P.-T. Chou and Y.-F. Chen, J. Phys. Chem. C, 2010, 114, 7995–7998 CrossRef CAS
.
- H.-S. Park, A. Agarawal, N. A. Kotov and O. D. Lavrentovich, Langmuir, 2008, 24, 13833–13837 CrossRef CAS
.
- Q. Liu, Y. Cui, D. Gardner, X. Li, S. He and I. I. Smalyukh, Nano Lett., 2010, 10, 1347–1353 CrossRef CAS
.
- K. Kanie and T. Sugimoto, J. Am. Chem. Soc., 2003, 125, 10518–10519 CrossRef CAS
.
- K. Kanie and A. Muramatsu, J. Am. Chem. Soc., 2005, 127, 11578–11579 CrossRef CAS
.
- L. Li, J. Walda, L. Manna and A. P. Alivisatos, Nano Lett., 2002, 2, 557–560 CrossRef CAS
.
- N. R. Jana, L. A. Gearheart, S. O. Obare, C. J. Johnson, K. J. Edler, S. Mann and C. J. Murphy, J. Mater. Chem., 2002, 12, 2909–2912 RSC
.
- M. Zorn, S. Meuer, M. N. Tahir, Y. Khalavka, C. Sonnichsen, W. Tremel and R. Zentel, J. Mater. Chem., 2008, 18, 3050–3058 RSC
.
- A. E. Saunders, A. Ghezelbash, D. Smilgies, M. B. Sigmann Jr and B. A. Korgel, Nano Lett., 2006, 6, 2959–2963 CrossRef CAS
.
- H. K. Bisoyi and S. Kumar, J. Indian Inst. Sci., 2009, 89, 101–112 Search PubMed
, and references therein.
- J. P. F. Lagerwall and G. Scalia, J. Mater. Chem., 2008, 18, 2890–2898 RSC
, and references therein.
- C. Zakri, Liq. Cryst. Today, 2007, 16, 1–11 CrossRef CAS
.
- I. Dierking, G. Scalia and P. Morales, J. Appl. Phys., 2005, 97, 044309 CrossRef
, and references therein.
- S. Kumar and H. K. Bisoyi, Angew. Chem., Int. Ed., 2007, 46, 1501–1503 CrossRef CAS
.
- H. K. Bisoyi and S. Kumar, J. Mater. Chem., 2008, 18, 3032–3039 RSC
.
- M. Kimura, N. Miki, N. Adachi, Y. Tatewaki, K. Ohta and H. Shirai, J. Mater. Chem., 2009, 19, 1086–1092 RSC
.
- H. S. Jeong, Y. K. Ko, Y. H. Kim, D. K. Yoon and H.-T. Jung, Carbon, 2010, 48, 774–780 CrossRef CAS
.
- Y. Ji, Y. Y. Huang, R. Rungsawang and E. M. Terentjev, Adv. Mater., 2010, 22, 3436 CrossRef CAS
.
- J. Lagerwall, G. Scalia, M. Haluska, U. Dettlaff-Weglikowska, S. Roth and F. Giesslmann, Adv. Mater., 2007, 19, 359–364 CrossRef CAS
.
- X. Xin, H. Li, E. Kalwarczyk, A. Kelm, M. Fialkowski, E. Gorecka, D. Pociecha and R. Holyst, Langmuir, 2010, 26, 8821–8828 CrossRef CAS
.
- Y. S. Kwon, B. M. Jung, H. Lee and J. Y. Chang, Macromolecules, 2010, 43, 5376–5381 CrossRef CAS
.
- C. Doe, H.-S. Jang, S. R. Kline and S.-M. Choi, Macromolecules, 2010, 43, 5411–5416 CrossRef CAS
.
- W. Song, I. A. Kinloch and A. H. Windle, Science, 2003, 302, 1363–1363 CrossRef CAS
.
- S. Zhang and S. Kumar, Small, 2008, 4, 1270–1283 CrossRef CAS
, and references therein.
- H. W. Lee, W. You, S. Barman, S. Hellstrom, M. C. LeMieux, J. H. Oh, S. Liu, T. Fujiwara, W. M. Wang, B. Chen, Y. W. Jin, J. M. Kim and Z. Bao, Small, 2009, 5, 1019–1024 CrossRef CAS
.
- L. Zhi, J. Wu, J. Li, U. Kolb and K. Mullen, Angew. Chem., Int. Ed., 2005, 44, 2120–2123 CrossRef CAS
.
- J. P. Hill, W. Jin, A. Kosaka, T. Fukushima, H. Ichihara, T. Shimomura, K. Ito, T. Hashizume, N. Ishii and T. Aida, Science, 2004, 304, 1481–1483 CrossRef CAS
.
- R. Deschenaux, B. Donnio and D. Guillon, New J. Chem., 2007, 31, 1064–1073 RSC
.
- S. Campidelli, T. Brandmuller, A. Hirsch, I. M. Saez, J. W. Goodby and R. Deschenaux, Chem. Commun., 2006, 4282–4284 RSC
.
- A. de la Escosura, M. V. Martinez-Diaz, J. Barbera and T. Torres, J. Org. Chem., 2008, 73, 1475–1480 CrossRef CAS
.
- R. J. Bushby, I. W. Hamley, Q. Liu, O. R. Lozman and J. E. Lydon, J. Mater. Chem., 2005, 15, 4429–4434 RSC
.
- M. Sawamura, K. Kawai, Y. Matsuo, K. Kanie, T. Kato and E. Nakamura, Nature, 2002, 419, 702–705 CrossRef CAS
.
- Y. Matsuo, A. Muramatsu, R. Hamasaki, N. Mizoshita, T. Kato and E. Nakamura, J. Am. Chem. Soc., 2004, 126, 432–433 CrossRef CAS
.
- C.-Z. Li, Y. Matsuo and E. Nakamura, J. Am. Chem. Soc., 2009, 131, 17058–17059 CrossRef CAS
.
- J.-C. Loudet, P. Barois and P. Poulin, Nature, 2000, 407, 611–613 CrossRef CAS
.
- I. Musevic, M. Skarabot, U. Tkalec, M. Ravnik and S. Zumer, Science, 2006, 313, 954–958 CrossRef CAS
.
- C. P. Lapointe, T. G. Mason and I. I. Smalyukh, Science, 2009, 326, 1083–1086 CrossRef CAS
.
- N. Behabtu, J. R. Lomeda, M. J. Green, A. L. Higginbotham, A. Sinitskii, D. V. Kosynkin, D. Tsentalovich, A. Nicholas, G. Parra-Vasquez, J. Schmidt, E. Kesselmann, Y. Cohen, Y. Talmon, J. M. Tour and M. Pasquali, Nat. Nanotechnol., 2010, 5, 406–411 CrossRef CAS
.
|
This journal is © The Royal Society of Chemistry 2011 |