DOI:
10.1039/C0AY00608D
(Minireview)
Anal. Methods, 2011,
3, 33-42
Electrochemiluminescence based on quantum dots and their analytical application
Received
11th October 2010
, Accepted 8th November 2010
First published on 20th December 2010
Abstract
This review presents a general description of the electrochemiluminescence (ECL) related to quantum dots (QDs) and their analytical application. It briefly overviews the synthetic route of quantum dots. The basic mechanisms are given for QDs ECL behavior. Finally, new developments and improvements of its application in inorganic substance analysis, organics analysis, immunoassay and aptasensing assay are discussed.
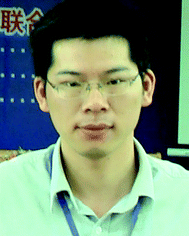 Haiping Huang | Dr Haiping Huang received his B. Sc. in 2003 from Southwest University and his M. Sc. in 2006 from Changchun Institute of Applied Chemistry, Chinese Academy of Sciences. In 2009 he obtained his Ph. D. from Nanjing University under the supervision of Prof. Jun-Jie Zhu. Currently he is an instructor at Jiangxi University of Science and Technology. General research interests include the synthesis, characterization and bio-related applications of novel nanomaterials. |
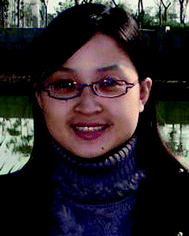 Jingjing Li | Jingjing Li obtained her B. Sc. in 2005 and M. Sc. in 2008 from Suzhou University. Currently she is pursuing her Ph. D. degree under the supervision of Prof. Jun-Jie Zhu and Jianrong Zhang at Nanjing University. The research interest of her Ph. D. study is mainly focused on the synthesis, characterization of novel nanomaterials and their conjugation with affinity ligand to construct biosensors. |
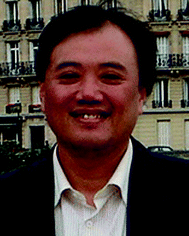 Jun-Jie Zhu | Jun-Jie Zhu is a Professor of Chemistry at Nanjing University, China. He received his B.Sc. (1984) and Ph.D. (1993) degrees from the Department of Chemistry, Nanjing University. Then, he began his academic career at Nanjing University. From 1998 to 1999, he entered Bar-Ilan University, Israel as a postdoctoral researcher. Since 2001, he has been a full professor at the School of Chemistry and Chemical Engineering, Nanjing University. His research interests are focused on developing electrochemical biosensors, functional nanomaterials and microfluidic chips. |
1. Introduction
As newly developed inorganic materials, semiconductor nanocrystals (NCs), or quantum dots (QDs), have been extensively studied because they possess unique optical properties including high quantum yield, low photobleaching, high photochemical stability, size-tunable emission and simultaneous excitation of multiple fluorescence colors.1–3 They have been widely used in cell imaging, bacteria detection, and immunoassay.4–6 In particular, Bard's group found that QDs could generate light emission during potential cycling or pulsing, which was called electrogenerated chemiluminescence (also known as electrochemiluminescence and abbreviated ECL).7,8 ECL technique involves the generation of species at electrode surface that undergo electron-transfer reactions to form excited states, and light is produced when the excited molecule decays to the ground state. It is the combination of chemiluminescence (CL) and electrochemistry. It not only holds the advantages of sensitivity and wide dynamic range inherent to conventional CL, but exhibits several advantages of the electrochemical method such as the simplicity, stability, facility, etc. Since the first detailed ECL investigations described by Hercules and Bard et al. in 1960s,9–11 ECL has now become a powerful analytical technique and been widely used in the areas of immunoassay,12–16 food and water testing17,18 biowarfare agent detection,19,20 and DNA and aptamer biosensors.21–24 ECL has also been successfully combined with other analytical techniques like flow injection analysis (FIA),25,26 high-performance liquid chromatography (HPLC),27,28 capillary electrophoresis,29 and micro total analysis (μTAS),30,31 and so on. After more than 40 years' development after the 1960s, ECL has been studied deeply and there are many reviews available in the literature,32–43 ranging from the fundamental theory, principle to the related application.
In this review, we mainly summarize most of the published achievement in QDs ECL. The QDs synthetic route, basic ECL mechanisms and its application in inorganic substance analysis, organics analysis, immunoassay and aptasensor assay are discussed in detail.
2. Synthesis of QDs
Multicolor QDs are usually obtained by accurately controlling time, temperature and ligand molecules in their synthesis.44 The two existent general strategies of high quality QDs preparation are organometallic synthesis and aqueous synthesis.45 Since the first report of formation of metal–chalcogen bonds by the reaction of metal alkyls with silyl chalcogenides by Steigerwald's group in 1989,46 QDs preparation is mainly based on an organometallic synthesis using high temperature solution reaction from organometallic precursors and QDs are capped with hydrophobic organic ligands which are made of a mixture of trioctylphosphine/trioctylphosphine oxide (TOP/TOPO) and alkyl amines.47,48 Dimethyl cadmium (Cd(CH3)2) was usually used as cadmium precursor in the synthesis of high-quality cadmium chalcogenides nanocrystals. Given that Cd(CH3)2 was extremely toxic, pyrophoric, expensive, unstable at room temperature and explosive at elevated temperatures by releasing large amounts of gas, Peng's group chose CdO to take the place of Cd(CH3)2 as the cadmium precursor and developed a much green-chemistry approach for synthesizing high-quality quantum rods and dots compared to the original organometallic synthetic route.49 However, the hydrophobic properties of QDs prepared in the organic phase limited their applications in biological domains. So further surface modification is necessary to make them water-soluble and biocompatible.50 Various strategies have been reported over the past decades to transfer QDs from the organic phase to the aqueous phase. The basic protocols used for such modification employed two main solubilization approaches: one depends on the ligand exchange with hydrophilic ligands such as thiol-containing molecules,51 dendrons52 and peptides,53 the other depends on the encapsulation by a layer of amphiphilic diblock,54 triblock copolymers55 or silica coating,56,57 phospholipid micelles.58 Nie and coworkers developed a new class of QD conjugate containing an amphiphilic triblock copolymer for in vivo protection, targeting-ligands for tumor antigen recognition and multiple PEG molecules for improved biocompatibility and circulation. Encapsulated QDs have a high quantum yield and stability, but these additional organic shells increase the nanocrystals hydrodynamic diameter which results in a barrier to the wider application of QDs for in vitro and in vivo biological imaging.59
In another aspect, the aqueous synthetic method has also been developed because of its simplicity, high reproducibility and lower toxicity. In general, 3-mercaptopropionic acid (MPA), 2-mercaptoethylamine acid (MA), thioglycolic acid (TGA) and L-cysteine are applied as the stabilizers in such aqueous synthetic routes.60,61 Taking CdTe QDs prepared by Gaponik as an example,45 there were two stages to synthesize thiol-capped CdTe QDs in the aqueous medium. The first stage was the formation of CdTe precursors by introducing H2Te gas which was generated by the reaction of Al2Te3 lumps with H2SO4 under N2 atmosphere. And the second stage was the formation and growth of CdTe nanocrystals promoted by reflux. Different sizes and colors of CdTe QDs were taken from the refluxing reaction mixture at different intervals of time. Up to now, series of QDs such as CdTe@CdSe,62 CdTe@CdS,63 Mn:ZnS,64 Mn:CdTe65 and CdSeTe alloyed quantum dots61 have been successfully synthesized in an aqueous medium. Furthermore, some QDs such as CdTe/CdS,66 CdTe/CdS/ZnS CSS QDs67 were synthesized in the aqueous phase assisted by microwave irradiation which could improve the photostability and quantum yield. However, nanocrystals synthesized by the aqueous approach do not possess the degree of crystallinity of the organometallically prepared QDs.45 Besides these two general approaches, the reverse microemulsion method which could yield more uniform spheres in the size range of 30–150 nm was employed to synthesize CdTe@SiO2 core-shell-structured fluorescent spheres.68
3. ECL of QDs
ECL involves the emission of light by species that can undergo highly energetic electron-transfer reactions which is controlled by changing an electrode potential (Fig. 1).
In generally, there are three main types of ECL: annihilation ECL, coreactant ECL and cathodic luminescence. Annihilation ECL is the first fully studied ECL which involves electron transfer reactions between an oxidized and a reduced species. After the material is electrochemically oxidized and reduced, the newly formed radical cation and anion are annihilated to form the excited state species that emits light.9,11 This approach is typically called “annihilation”, and a general mechanism is outlined below:43
| R − e− → R˙+ (oxidation at electrode) | (1) |
| R + e− → R˙− (reduction at electrode) | (2) |
| R˙+ + R˙− → R + R* (excited state formation) | (3) |
| R* → R + hν (light emission) | (4) |
Cathodic luminescence results from the injection of hot electrons into the aqueous electrolyte solution with the possible formation of hydrated electrons. For example, emission from Dy(III), Sm(III), and Tb(III) has been observed at oxide-covered aluminium electrodes during the reduction of hydrogen peroxide, persulfate, or oxylate in aqueous solution.70–72
Till now, coreactant ECL has been the most widely studied and used ECL technique and most of the commercially available ECL analytical instruments are based on coreactant ECL. Unlike annihilation ECL, coreactant ECL is usually generated with the reaction between the luminophore species and an additionally added assistant reagent which is called the coreactant in a single potential step or a directional potential scanning. Depending on the polarity of the applied potential, the corresponding ECL reactions could be classified as “oxidative reduction” ECL and “reductive oxidation” ECL, respectively. Compared with annihilation ECL, the use of a coreactant can enhance the ECL efficiency. The oxalate ion (C2O42−) was the first discovered coreactant73 and the ECL mechanism has been fully studied by Bard's group. 74
Other common coreactants include peroxydisulfate (persulfate, S2O82−), tri-n-propylamine (TPrA) and other amine-related derivatives, hydrogen peroxide (H2O2), etc. The main organic luminants contain luminol, tris(2,2′-bipyridine) ruthenium(II) (Ru(bpy)32+) and their derivatives.
Since Bard's group first reported the ECL property of silicon NCs in 2002,7 research about ECL of nanoparticles (NPs) has also received considerable interest in the last few years. The Si NCs have the ability to store charge in N,N′-dimethylformamide and acetonitrile, which can subsequently lead to light emission upon electron and/or hole transfer. This quality provides electrochemically sensitive optoelectronic properties. In 2006, they observed the ECL emission from silica NPs in aqueous solution.75 By using S2O82− as the coreactant, octadecyl-protected silica NPs deposited on indium tin oxide (ITO) showed ECL in both anodic and cathodic sweep potentials. In the negative potential scans, the Si NP film could produce a large ECL signal when the potential is beyond −0.95 V, the principle was described as follows:
| S2O82− + e− → SO42− + SO4− | (5) |
Another type of nanoparticle, QDs, has received considerable interest due to its excellent luminescent properties, fundamental and application research.76–79 Many elemental and compound semiconductors like Ge NCs8, CdTe NPs,80 PbS QDs,81 CdSe NCs82, 83 ZnS84 can generate efficient ECL. The ECL mechanism of semiconductor NCs mainly depends on the annihilation or coreactant ECL reaction. For example, PbS QDs can form oxidized (R˙+) and reduced (R˙−) QDs during potential cycling. Two oppositely charged QDs can collide to yield an excited QD in the annihilation process, and the same as CdSe and Ge QDs. An intense ECL signal of CdTe QDs was observed in electron transfer reaction between reduced NPs and reductively oxidized species of CH2Cl2 at negative potential region.
It is believed that the ECL emission is not sensitive to NP size and capping agent used but depends more sensitively on surface chemistry and the presence of surface states.85 So effective methods are being developed in order to chemically functionalize the surfaces of QDs with suitable ligands or receptors. Anodic ECL of mercaptopropionic acid (MCA) modified water-soluble CdTe QDs in aqueous systems has been reported. Stable and intensive anodic ECL emission with a peak value at +1.17 V (vs Ag/AgCl) in pH 9.3 phosphate buffered saline (PBS) at an ITO electrode was observed. The ECL emission involved the collision between the superoxide ion produced at the ITO surface and the oxidation products of QDs. The whole process of anodic ECL emission is described in the following equations86:
| In/SnOx + CdTe/CdSR → CdTe (h+)/CdSR + In/SnOx (e−) | (9) |
| O2 + In/SnOx (e−) → O2− + In/SnOx | (10) |
| CdTe/CdSR + O2− → CdTe (e−)/CdSR + O2 | (11) |
| CdTe (h+)/CdSR + CdTe (e−)/CdSR → CdTe/CdSR* | (12) |
| CdTe/CdSR* → CdTe/CdSR + hν | (13) |
Thioglycolic acid (TGA)-protected CdSe QDs for cathodic ECL research has also been studied.87 Chen's group prepared the CdS-polyamidoamine (CdS–PAMAM) nano-composite membranes88 and CdS NCs/CNTs composites.89Via the in situ electrochemical reduction approach, CdS–PAMAM nanocomposite membranes were prepared on electrode surfaces, and this resulting film showed 55-fold enhanced red ECL compared with that of CdS nano-film without PAMAM. After combination with CNTs, the CdS NCs/CNTs composites not only enhanced their electrochemiluminescent intensity but also decreased their ECL starting potential.
In our group, a series of nanoparticles were synthesized and the corresponding ECL property was investigated. In 2005, we first reported the ECL property of CdS spherical nanoparticles. Four ECL peaks were observed in both nonaqueous systems and aqueous systems.90 According to the electrochemical behavior of the as-prepared CdS assemblies and the two-equivalent adsorbed surface state theory, we proposed the mechanism for ECL as annihilation mechanism. Next, we studied the ECL behavior of CdS nanotubes in aqueous solution. 91,92 By entrapping the CdS nanotubes in a carbon paste electrode, two respective ECL peaks at the potential −0.9 V (vs Ag/AgCl sat. KCl, sic passim, ECL-1) and −1.2 V (ECL-2) were observed when the potential was cycled between 0 and −1.6V. The ECL-1 is generated via an annihilation process between reduced and oxidized species. The ECL-2 is attributed to the electron-transfer reaction between electrochemically reduced CdS nanocrystal species (CdS˙−) and coreactants (S2O82− or H2O2). The corresponding ECL processes are as follows: 92
S2O82− as coreactant:
| CdS NCs + ne− → nCdS˙− | (14) |
| S2O82− + e− → SO42− + SO4˙− | (15) |
| CdS˙− + SO4˙− → CdS* + SO42− | (16) |
H2O2 as coreactant:
| O2 + H2O + 2e− → OOH− + OH− | (18) |
| 2CdS˙− + OOH− + H2O → 3OH− + 2CdS* | (19) |
| 2CdS˙− + H2O2 → 2OH− + 2CdS* | (20) |
Next, we studied the enhancement ECL effect of 3-aminopropyl-triethoxysilane (APS) on the CdSe NCs in aqueous solution by conjugating APS to the CdSe NCs/CNT-CHIT composite film.16 In the presence of reactive amine groups, APS can facilitate the radical generation and electron-transfer processes during the ECL reaction. After the addition of K2S2O8 as coreactant, APS can catalyze the reaction of CdSe NCs with K2S2O8 based on the following mechanism:
| CdSe NCs + ne− → nCdSe˙− | (22) |
| S2O82− + RC3H7–NH2 → SO42− + SO4˙− + RC3H7–NH2+˙ | (23) |
| CdSe˙− + SO4˙− → CdSe * + SO42− | (24) |
4. QDs ECL for inorganic analysis
The combination of sensitive ECL with selective biological interactions such as enzymatic reactions, immunoassays, DNA/aptamer-probe assays has gained more and more interest over the past years. ECL allows the detection of analytes at low concentrations over a wide linear range. What is more, ECL can combine with many other analytical methods such as high performance liquid chromatography (HPLC), liquid chromatography (LC), capillary electrophoresis (CE) and flow injection analysis (FIA), etc. Listed in Table 1 are the ECL applications of QDs.
Table 1 ECL application of QDs in biosensors
Most of the ECL biosensors are based on the quenching, inhibition, or enhancement of the ECL intensities via the well-studied coreactant ECL systems which took S2O82−, H2O2, SO32− as the coreactants, etc. In 2004, Ju's group reported a QD-based ECL sensor in aqueous system based on the electron-transfer reaction.101 By using CdSe QDs as the luminophor and H2O2 as the coreactant, two ECL peaks were observed which resulted from the reaction between coreactants and individual reduced nanocrystal species (ECL-1) and the reaction between coreactants and assembly of CdSe reduced nanocrystal species (ECL-2). The electrochemical and ECL process can be expressed as follows:
| O2 + H2O + 2e → OOH− + OH− | (27) |
| 2R˙− + OOH− + H2O → 3OH− + 2R* | (28) |
Or
| 2R˙− + H2O2 → 2OH− + 2R* | (29) |
Based on the fact that the ECL-1 was more sensitive to the dissolved oxygen and H2O2, ECL-1 was chosen to detect H2O2. A linear relationship between the ECL-1 intensity and H2O2 concentration was obtained from 2.5 × 10−7 to 6 × 10−5 M with a correlation coefficient of 0.9992. The detection limit was 0.1 μM at a signal-to-noise ratio of 3. Other QD ECL biosensors for H2O2 detection were also reported with different QDs using luminophors such as CdS,92,100 CdTe,96 ZnSe98 and composites like Au/CdS,97 Mn2+-Doped ZnS,99etc.
Han et al. prepared the thiol-capped CdTe QDs and studied its ECL property.96 In the presence of H2O2, the CdTe QDs could be excited to give rise to light emission. Based on the enhancement of ECL of CdTe QDs by H2O2 in the negative electrode potential, a novel method for the determination of H2O2 was developed. The light intensity was linearly proportional to the concentration of H2O2 between 2.0 × 10−7 and 1.0 × 10−5 M with a correlation coefficient of 0.9994, and the limit of detection was 6.0 × 10−8 M at a signal-to-noise ratio of 3. Most recently, they synthesized non-cadmium and low-toxicity ZnSe QDs and observed the blue emitting ECL QDs in aqueous system.98 The ECL intensity was also greatly enhanced in the presence of H2O2 or dissolved oxygen. Under the optimal conditions, the dynamic range is from 6.10 × 10−7 to 3.10 × 10−4 M H2O2, and the detection limit (at S/N= 3) is 2.00 × 10−7 M.
Notably, Chen's group prepared a novel bi-functional sensor via the layer-by-layer (LbL) assembly. Multilayer films of CdS NCs and Hb, designated as {Hb/CdS}n, were adsorbed onto the glassy carbon electrode (GCE).100 In this multilayer composite films, Hb can enhance the stability of electrogenerated species of CdS NCs, and CdS NCs can also promote the direct electron transfer between hemoglobin and the GCE. With the help of this synergistic effect, the multilayer films modified GCE is suitable to be used as a bi-functional sensor, ECL sensor and electrochemical sensor, to determine H2O2 in obviously different concentration (Fig. 2).
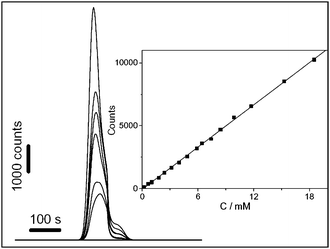 |
| Fig. 2 Effect of H2O2 concentration on ECL intensity of {Hb/CdS}5-chitosan-GCE in 0.1M PBS (pH 9.6) containing 0, 4.0, 4.8, 8.4, 9.9, 12, and 18 mM H2O2 (from lower to upper). Inset: calibration curve for H2O2 detection. Reprinted with permission from ref. 100. Copyright 2007 Elsevier. | |
In our group, the ECL from CdS nanotubes was observed, and its application for H2O2 detection was also studied by entrapping the CdS nanotubes in a carbon paste electrode.92 The hydrophobicity of the used paraffin resulted in the formation of a clear O/W interface between immobilized CdS nanotubes and aqueous solution. The method of using carbon paste to immobilize CdS nanocrystals provided an easy renewable modified electrode surface. All these provided a more effective way with better stability and higher sensitivity for H2O2 detection.
Besides H2O2, other inorganic substances like Cu2+ and nitrite could also be detected via the ECL technique. Based on the ECL quenching of QDs in aqueous solution, Dong et al.93 and Wang et al.94 reported the ECL method to detect Cu2+ with high sensitivity and selectivity. In the presence of Cu2+, surfactant was removed from the surface of the QDs and preferentially bound with Cu2+. The displacement of surfactant capping layer created imperfections on the QDs surface, and this eventually led to the ECL quenching. The ECL quenching effect was used for another inorganic substance in the detection of nitrite.95
5. QDs ECL for organics analysis
Unlike H2O2 that could be directly involved in the ECL chemical reaction as coreactant, the organics detections are mostly based on their quenching effect of QDs ECL. The quenching mechanism relied on the energy transfer process between the excited molecule and the byproduct of the analyte. After reacting with the analyte, the excited QDs or coreactant decreased, and this directly resulted in a decrease of QDs ECL intensity. According to the Stern–Volmer equation, this quenching principle could be described as follows:115where I0 is the initial ECL intensity, I is the ECL intensity at a given concentration of quencher [Q], and Ksv is the quenching constant.
Following this quenching principle, the anodic ECL of CdTe QDs in aqueous system was reported.86 With catechol derivatives as model analytes, the ECL energy transfer was observed from the excited QDs to quencher, which resulted in the decrease of anodic ECL emission of QDs. Based on this, a method for ECL detection of quencher-related analytes was proposed, which was also used for ECL analysis of L-adrenalin, tyrosine15 and glutathione106. Subsequently, they developed another biosensor for dopamine detection based on the energy transfer from the excited QDs to the electro-oxidized product of dopamine.105
In order to amplify the QDs ECL emission, Li's group recently developed an ECL pathway of CdTe QDs with the participation of graphene oxide.104 Graphene oxide could facilitate the CdTe QDs˙+ production and triggered O2˙− generation. A higher yield of CdTe QDs* could be formed after the combination of CdTe QDs˙+ and O2˙−, leading to an ∼5-fold ECL amplification. Furthermore, they used this proposed platform for glutathione-selective detection from thiol-containing compounds with a wide linear relationship. This platform was also used for the direct, sensitive, and continuous detection of the glutathione-containing drug isethion.
Other QDs ECL quenching principles were also employed for organic analysis. In the air-saturated solution, the dissolved oxygen was an important coreactant for producing ECL in the QDs solution. some thiol compounds could react with the dissolved oxygen or their intermediate species produced in the processes of QD ECL, which resulted in decreasing the ECL intensity. The possible quenching effect could be concluded using the following reaction:
|  | (32) |
Based on this, the QDs ECL detection of gossypol,102L-cysteine103 and methimazole107 was developed by Han et al. as for the cysteine detection, the ECL intensity decreased linearly with the concentrations of L-cysteine in the range from 1.3 × 10−6 to 3.5 × 10−5 M with a correlation coefficient of 0.996. The detection limit (S/N = 3) was found to be 8.7 × 10−7 M.
According to the above discussion, we know that both oxygen and hydrogen peroxide can act as the ECL coreactants, however, the efficiencies of the two coreactants are different. Since O2 can capture more electrons from electrochemically reduced QDs than H2O2 and their reaction rates with the reduced QDs also differ. Based on this, a simple strategy for the fabrication of a glucose biosensor based on the intrinsic ECL of QDs coupled with an enzymatic reaction is proposed with glucose oxidase as the model.87 In a typical GOD catalytic cycle, the dissolved oxygen is consumed to produce equimolar hydrogen peroxide, so the ECL response of GOD–QDs film should decrease upon addition of glucose. They concluded that the concise ECL pathways in the presence of glucose can be expressed as follows:
| CdSe/Cd(TGA) QDs + ne → nR˙− | (33) |
| Catalyzed by GOD: Glucose + O2 → Gluconate + H2O2 | (34) |
| For oxygen: O2 + 2R˙− + 2H+ → 2R* + H2O2 | (35) |
| H2O2 + 2R˙− → 2R* + 2OH− | (36) |
| For peroxide: H2O2 + 2R˙− → 2R* + 2OH− | (37) |
By employing this strategy, the linear range for glucose was from 25.0 μM to 3.0 mM (R = 0.995) with a detection limit of 4.0 μM at an S/N of 3.
6. QDs ECL for immunoassay
The sensitive measurement of proteins is critical to many aspects of biochemical and biomedical research. The ECL immunosensor combines the high sensitivity of the ECL detection with the specificity of the immunoreaction. Different from the above discussed ECL process, in the QDs ECL immunoassay, the detected analytes are not involved in the ECL chemical reactions, either directly or indirectly; it just effects the amount of QDs that are integrated into the immunosystem. In 2007, our group explored the ECL properties of water-soluble CdS NCs and developed a novel NCs ECL biosensor for low-density lipoprotein (LDL) detection via self-assembly and nanoparticle amplification techniques (Fig. 3).111
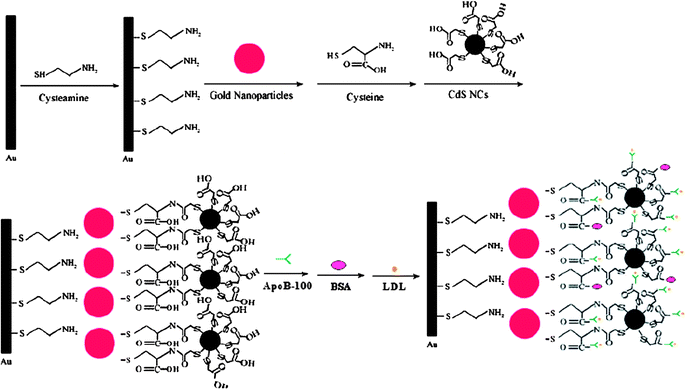 |
| Fig. 3 Schematic diagram for the biosensor fabrication and LDL binding. Reprinted with permission from ref. 111. Copyright 2007 American Chemical Society. | |
In this system, an Au nanoparticle layer was first conjugated to the Au electrode surface. In the next, CdS NCs, apoB-100 and LDL were conjugated to the Au layer-electrode in sequence. The specific binding of apoB-100 with LDL greatly inhibited the ECL reaction of CdS NCs on the electrode, and thus decreased the ECL intensity. In the range from 0.025 to 16 ng mL−1, the ECL intensity decreased linearly with the increasing concentrations of LDL. This method is rapid, cost-effective, specific, and ultrasensitive for bioassays.
Another label-free ECL immunosensor for the sensitive detection of human IgG (HIgG) was fabricated by combining CdSe nanocrystals (NCs), carbon nanotube-chitosan (CNT-CHIT), and 3-aminopropyl-triethoxysilane (APS).16 In the presence of reactive amine groups, APS can be used as an efficient cross-linker for the conjugation of biomolecules. After the formation of an immunocomplex between the antibody and HIgG, the ECL intensity decreased linear with the HIgG concentration from 0.02 to 200 ng mL−1 with a detection limit of 0.001 ng mL−1. In order to improve the detection performance of the ECL biosensor, gold NPs were used for the ECL signal amplification. After gold NPs were assembled onto the QDs modified electrode, the linear range was enlarged to 0.002–500 ng L−1108 and 0.006–150 ng mL−1109 respectively. Based on the similar inhibition process, a recent investigation about the use of ECL CdSe QDs for detection of human prealbumin (PAB, antigen) has been reported.110 In this case, the immunocomplex increased the steric hindrance and inhibited the transfer of K2S2O8 to the surface of the CdSe QD-electrode, thus inhibiting the ECL reaction between CdSe QDs and the coreactant K2S2O8, and eventually resulting in the decrease of ECL intensity. This allowed ng mL−1 concentration of PAB to be detected and the detection limit was as low as 1.0 × 10−11 g mL−1
7. QDs ECL application for aptasensor assay
Aptamers are a new class of single-stranded DNA/RNA molecules, which are selected from synthetic nucleic acid libraries via the selection procedure called SELEX (systematic evolution of ligands by exponential enrichment). Since its first discovery in the 1990s,116,117 many aptamers have been selected for corresponding targets combination ranging from metal ions, organic molecules, biomolecules, to entire organism and even whole cells. Aptamers offer remarkable convenience in the design and modification of their structures, which has motivated them to generate a great variety of aptamer sensors (aptasensors) that exhibit high sensitivity as well as specificity. In our group, by using the QDs ECL as the signal transduction, a series of aptasensors were developed for the detection of thrombin, lysozyme and adenosine 50-triphosphate (ATP), etc. Through the aptamer–target specific affinity and the rules of Watson–Crick base pairing, aptasensors for lysozyme112 and ATP114 detection were fabricated respectively (Fig. 4). Take the ATP detection as an example, after the thiol modified anti-ATP probes were immobilized onto the pretreated Au electrode, ATP solution was dropped for the formation of aptamer–ATP bioaffinity complexes. The biotin modified complementary DNA (biotin-cDNA, in terms to the probe) oligonucleotides were hybridized with the remnant free probes. At last, the avidin-modified QDs were bound to the aptasensor through the biotin–avidin system in the presence of biotin-cDNA. The ECL intensity, as the readout signal for the aptasensor, was responsive to the amount of QDs bonded to the cDNA oligonucleotides, which was indirectly inversely proportional to the combined target analyte.
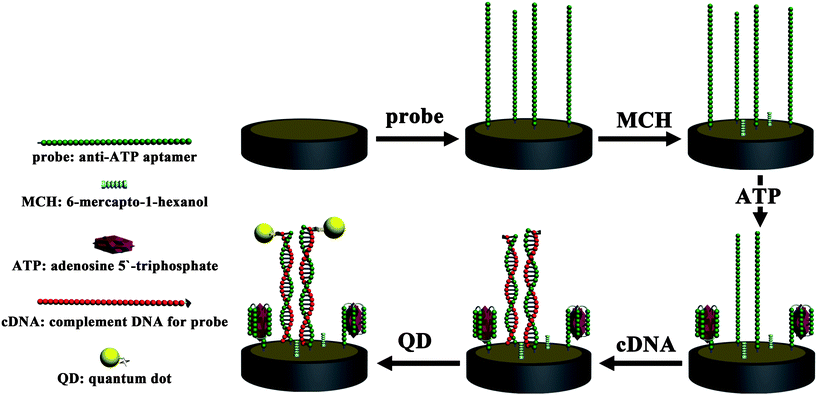 |
| Fig. 4 Schematic diagram for the biosensor fabrication for ATP detection. Reprinted with permission from ref. 114. Copyright 2010 Royal Society of Chemistry. | |
In the case where thrombin owns two different aptamers, we ingeniously graft the concept of sandwich immunoreaction onto the aptamer field and constructed a sandwich aptasensor by using QDs ECL technique for thrombin detection.113 The thiol-terminated aptamer with 15 nucleotides (probe I) was first immobilized on Au electrode, then thrombin and another 5`-biotin modified aptamer (29 nucleotides, probe II) was incubated to the above electrode respectively, in order to form the sandwich structure of probe I/thrombin/probe II. Streptavidin modified QDs (avidin–QDs) were bound to probe II via the biotin–avidin-system. Thrombin was detected by measuring the ECL intensity of the bound QDs. It provided an alternative convenient, low-cost and specific method for protein detection. What is more, the application of QDs ECL in aptasensors may intrigue researchers into gaining a new interest in the investigation of the QDs ECL and promote the exploitation in the bioapplications.
8. Conclusions
Since the first detailed ECL studies of Si QDs, both fundamental and applicational research of QDs ECL has rapidly developed over the past several years. Large numbers of papers have been published ranging from the synthesis, the study of its ECL mechanism to the application. Via the type of coreactant ECL, QDs could generate efficient and stable ECL via the coreaction with different coreactant such as sulfite (SO32−), O2, H2O2 and persulfate (S2O82−), etc. Through surface functional modification by coating with protecting and stabilizing shell, QDs could be used safely for subsequent bioconjugation with proteins, peptides and even the whole cell. CNTs, Au NPs and other nanomaterials could be composited with QDs in order to amplify the ECL signal. Based on these, QDs ECL sensors could be fabricated for detection ranging from inorganic analysis, organic analysis to immunoassays and aptasensor assays. Together with QDs' efficient and stable luminescent properties, surface functional modification, and ECL's high sensitivity and selectivity, QDs ECL will continue to be an useful tool in developing novel biosensing applications and understanding the fundamental questions involved in biological and chemical processes.
Acknowledgements
We greatly appreciate the support of the National Natural Science Foundation of China (20635020, 50972058, 21005034). This work is also supported by National Basic Research Program of China (2011CB933502).
Notes and references
- Y. D. Yin and A. P. Alivisatos, Nature, 2005, 437, 664 CrossRef CAS.
- A. P. Alivisatos, Science, 1996, 271, 933 CrossRef CAS.
- C. Burda, X. B. Chen, R. Narayanan and M. A. El-Sayed, Chem. Rev., 2005, 105, 1025 CrossRef CAS.
- R. Edgar, M. McKinstry, J. Hwang, A. B. Oppenheim, R. A. Fekete, G. Giulian, C. Merril, K. Nagashima and S. Adhya, Proc. Natl. Acad. Sci. U. S. A., 2006, 103, 4841 CrossRef CAS.
- R. Bakalova, Z. Zhelev, H. Ohba and Y. Baba, J. Am. Chem. Soc., 2005, 127, 9328 CrossRef CAS.
- X. Y. Wu, H. J. Liu, J. Q. Liu, K. N. Haley, J. A. Treadway, J. P. Larson, N. F. Ge, F. Peale and M. P. Bruchez, Nat. Biotechnol., 2003, 21, 41 CrossRef CAS.
- Z. Ding, B. M. Quinn, S. K. Haram, L. E. Pell, B. A. Korgel and A. J. Bard, Science, 2002, 296, 1293 CrossRef CAS.
- N. Myung, X. Lu, K. P. Johnston and A. J. Bard, Nano Lett., 2004, 4, 183 CrossRef CAS.
- D. M. Hercules, Science, 1964, 143, 308.
- R. E. Visco and E. A. Chandross, J. Am. Chem. Soc., 1964, 86, 5350 CrossRef CAS.
- K. S. Santhanam and A. J. Bard, J. Am. Chem. Soc., 1965, 87, 139 CrossRef CAS.
- C. Bertolino, M. MacSweeney, J. Tobin, B. O'Neill, M. M. Sheehan, S. Coluccia and H. Berney, Biosens. Bioelectron., 2005, 21, 565 CrossRef CAS.
- W. Zhan and A. J. Bard, Anal. Chem., 2007, 79, 459 CrossRef CAS.
- K. Ryoji, A. Kumi, N. Kohei, K. Dai and N. Osamu, Anal. Chem., 2010, 82, 1692 CrossRef CAS.
- X. Liu and H. X. Ju, Anal. Chem., 2008, 80, 5377 CrossRef CAS.
- G. F. Jie, J. J. Zhang, D. C. Wang, C. Cheng, H. Y. Chen and J. J. Zhu, Anal. Chem., 2008, 80, 4033 CrossRef CAS.
- V. R. Rivera, F. J. Gamez, W. K. Keener and M. A. Poli, Anal. Biochem., 2006, 353, 248 CrossRef CAS.
- L. R. Luo, Z. J. Zhang, L. J. Chen and L. F. Ma, Food Chem., 2006, 97, 355 CrossRef CAS.
- J. G. Bruno and J. L. Kiel, Biosens. Bioelectron., 1999, 14, 457 CrossRef CAS.
- A. Wolter, R. Niessner and M. Seidel, Anal. Chem., 2008, 80, 5854 CrossRef CAS.
- J. Zhang, H. L. Qi, Y. Li, J. Yang, Q. Gao and C. X. Zhang, Anal. Chem., 2008, 80, 2888 CrossRef CAS.
- X. Y. Wang, W. Yun, P. Dong, J. M. Zhou, P. G. He and Y. Z. Fang, Langmuir, 2008, 24, 2200 CrossRef CAS.
- L. Z. Hu, Z. Bian, H. J. Li, S. Han, Y. L. Yuan, L. X. Gao and G. B. Xu, Anal. Chem., 2009, 81, 9807 CrossRef CAS.
- X. B. Yin, Y. Y. Xin and Y. Zhao, Anal. Chem., 2009, 81, 9299 CrossRef CAS.
- Y. Chi, J. Duan, S. Lin and G. Chen, Anal. Chem., 2006, 78, 1568 CrossRef CAS.
- Y. Chi, Y. Dong and G. Chen, Electrochem. Commun., 2007, 9, 577 CrossRef CAS.
- Y. Sun, Z. Zhang and Z. Xi, Anal. Chim. Acta, 2008, 623, 96 CrossRef CAS.
- H. N. Choi, S. H. Cho, Y. J. Park, D. W. Lee and W. Y. Lee, Anal. Chim. Acta, 2005, 541, 47 CrossRef.
- J. Wang, Z. Yang, X. Wang and N. Yang, Talanta, 2008, 76, 85 CrossRef CAS.
- X. B. Jin, J. Kang, L. Fang, X. R. Yang and E. K. Wang, J. Chromatogr., A, 2004, 1055, 223 CrossRef CAS.
- H. Qiu, J. Yan, X. P. Sun, J. Liu, W. Cao, X. R. Yang and E. K. Wang, Anal. Chem., 2003, 75, 5435 CrossRef CAS.
-
A. J. Bard, Electrogenerated Chemiluminescence; Ed.; Dekker: New York, 2004 Search PubMed.
- M. M. Richter, Chem. Rev., 2004, 104, 3003 CrossRef CAS.
- Y. Du and E. Wang, J. Sep. Sci., 2007, 30, 875 CrossRef CAS.
- K. A. Fähnrich, M. Pravda and G. G. Guilbault, Talanta, 2001, 54, 531 CrossRef CAS.
- X. B. Yin and E. K. Wang, Anal. Chim. Acta, 2005, 533, 113 CrossRef CAS.
- W. Y. Lee, Microchim. Acta, 1997, 127, 19 CrossRef CAS.
- A. W. Knight, TrAC, Trends Anal. Chem., 1999, 18, 47 CrossRef CAS.
- C. A. Marquette and L. J. Blum, Anal. Bioanal. Chem., 2008, 390, 155 CrossRef CAS.
- R. D. Gerardi, N. W. Barnett and S. W. Lewis, Anal. Chim. Acta, 1999, 378, 1 CrossRef CAS.
- B. A. Gorman, P. S. Francis and N. W. Barnett, Analyst, 2006, 131, 616 RSC.
-
(a) B. Paolo and J. F. Robert, Biosens. Bioelectron., 2009, 24, 3191 CrossRef CAS;
(b) H. L. Qi, Y. G. Peng, Q. Gao and C. X. Zhang, Sensors, 2009, 9(1), 674 CrossRef CAS.
- W. J. Miao, Chem. Rev., 2008, 108, 2506 CrossRef CAS.
- S. M. Nie, Y. Xing, G. J. Kim and J. W. Simons, Annu. Rev. Biomed. Eng., 2007, 9, 257 CrossRef CAS.
- N. Gaponik, D. V. Talapin, A. L. Rogach, K. Hoppe, E. V. Shevchenko, A. Kornowski, A. Eychmu1ller and H. Weller, J. Phys. Chem. B, 2002, 106, 7177 CrossRef CAS.
- S. M. Stuczynski, J. G. Brennan and M. L. Steigerwald, Inorg. Chem., 1989, 28, 4431 CrossRef CAS.
- K. Susumu, T. Uyeda, I. L. Medintz, T. Pons, J. B. Delehanty and H. Mattoussi, J. Am. Chem. Soc., 2007, 129, 13987 CrossRef CAS.
- A. R. Clapp, E. R. Goldman and H. Mattoussi, Nat. Protoc., 2006, 1, 1258 CrossRef CAS.
- Z. A. Peng and X. G. Peng, J. Am. Chem. Soc., 2001, 123, 183 CrossRef CAS.
- X. Michalet, F. F. Pinaud, L. A. Bentolila, J. M. Tsay, S. Doose, J. J. Li, G. Sundaresan, A. M. Wu, S. S. Gambhir and S. Weiss, Science, 2005, 307, 538 CrossRef CAS.
- W. C. W. Chan and S. M. Nie, Science, 1998, 281, 2016 CrossRef CAS.
- W. Z. Guo, J. J. Li, Y. A. Wang and X. G. Peng, Chem. Mater., 2003, 15, 3125 CrossRef CAS.
- F. Pinaud, D. King, H. P. Moore and S. Weiss, J. Am. Chem. Soc., 2004, 126, 6115 CrossRef CAS.
- X. Y. Wu, H. J. Liu, J. Q. Liu, K. N. Haley, J. A. Treadway, J. P. Larson, N. F. Ge, F. Peale and M. P. Bruchez, Nat. Biotechnol., 2003, 21, 41 CrossRef CAS.
- X. H. Gao, Y. Y. Cui, R. M Levenson, L. W. K. Chung and S. M. Nie, Nat. Biotechnol., 2004, 22, 969 CrossRef CAS.
- M. J. Bruchez, M. Moronne, P. Gin, S. Weiss and A. P. Alivisatos, Science, 1998, 281, 2013 CrossRef CAS.
- D. Gerion, F. Pinaud, S. C. Williams, W. J. Parak, D. Zanchet, S. Weiss and A. P. Alivisatos, J. Phys. Chem. B, 2001, 105, 8861 CrossRef CAS.
- B. Dubertret, P. Skourides, D. J. Norris, V. Noireaux, A. H. Brivanlou and A. Libchaber, Science, 2002, 298, 1759 CrossRef CAS.
- W. Mahmoud, A. Sukhanova, V. Oleinikov, Y. P. Rakovich, J. F. Donegan, M. Pluot, J. H. M. Cohen, Y. R. Volkov and I. Nabiev, Proteomics, 2010, 10, 700 CrossRef CAS.
- H. Z. Sun, H. Zhang, J. Ju, J. H. Zhang, G. Qian, C. L. Wang, B. Yang and Z. Y. Wang, Chem. Mater., 2008, 20, 6764 CrossRef CAS.
- G. X. Liang, M. M. Gu, J. R. Zhang and J. J. Zhu, Nanotechnology, 2009, 20, 415103 CrossRef.
- R. S. Zeng, T. T. Zhang, J. C. Liu, S. Hu, Q. Wan, X. M. Liu, Z. W. Peng and B. S. Zou, Nanotechnology, 2009, 20, 095102 CrossRef.
- Q. H. Zeng, X. G. Kong, Y. J. Sun, Y. L. Zhang, L. P. Tu, J. L. Zhao and H. Zhang, J. Phys. Chem. C, 2008, 112, 8587 CrossRef CAS.
- K. Sooklal, B. S. Cullum, S. M. Angel and C. J. Murphy, J. Phys. Chem., 1996, 100, 4551 CrossRef.
- G. X. Liang, H. C. Pan, Y. Li, L. P. Jiang, J. R. Zhang and J. J. Zhu, Biosens. Bioelectron., 2009, 24, 3693 CrossRef CAS.
- Y. He, H. T. Lu, L. M. Sai, W. Y. Lai, Q. L. Fan, L. H. Wang and W. Huang, J. Phys. Chem. B, 2006, 110, 13370 CrossRef.
- Y. He, H. T. Lu, L. M. Sai, Y. Y. Su, M. Hu, C. H. Fan, W. Huang and L. H. Wang, Adv. Mater., 2008, 20, 3416 CrossRef CAS.
- Y. H. Yang and M. Y. Gao, Adv. Mater., 2005, 17, 2354 CrossRef CAS.
-
W. Miao, in Handbook of Electrochemistry; Zoski, C. G., Ed.; Elsevier: Amsterdam, The Netherlands, 2007; p543 Search PubMed.
- S. Kulmala, T. Ala-Kleme, L. Vare, M. Helin and T. Lehtinen, Anal. Chim. Acta, 1999, 398, 41 CrossRef CAS.
- J. Kankare, K. Falden, S. Kulmala and K. Haapakka, Anal. Chim. Acta, 1992, 256, 17 CrossRef CAS.
- J. Kankare, K. Haapakka, S. Kulmala, V. Nanto, J. Eskola and H. Takalo, Anal. Chim. Acta, 1992, 266, 205 CrossRef CAS.
- M. M. Chang, T. Saji and A. J. Bard, J. Am. Chem. Soc., 1977, 99, 5399 CrossRef CAS.
- I. Rubinstein and A. J. Bard, J. Am. Chem. Soc., 1981, 103, 512 CrossRef CAS.
- Y. Bae, D. C. Lee, E. V. Rhogojina, D. C. Jurbergs, B. A. Korgel and A. J. Bard, Nanotechnology, 2006, 17, 3791 CrossRef CAS.
- M. Bruchez, M. Moronne, P. Gin, S. Weiss and P. A. Alivisatos, Science, 1998, 281, 2013 CrossRef CAS.
- W. Chan and S. Nie, Science, 1998, 281, 2016 CrossRef CAS.
- S. Chen, L. A. Truax and J. M. Sommers, Chem. Mater., 2000, 12, 3864 CrossRef CAS.
- X. Michalet, F. F. Pinaud, L. A. Bentolila, J. M. Tsai, S. Doose, J. J. Li, G. Sundaresan, A. M. Wu, S. S. Gambhir and S. Weiss, Science, 2005, 307, 538 CrossRef CAS.
- Y. Bae, N. Myung and A. J. Bard, Nano Lett., 2004, 4, 1153 CrossRef CAS.
- L. F. Sun, L. Bao, B. R. Hyun, A. C. Bartnik, Y. W. Zhong, J. C. Reed, D. W. Pang, H. D. Abruña, G. G. Malliaras and F. W. Wise, Nano Lett., 2009, 9, 789 CrossRef CAS.
- N. Myung, Z. Ding and A. J. Bard, Nano Lett., 2002, 2, 1315 CrossRef CAS.
- N. Myung, Y. Bae and A. J. Bard, Nano Lett., 2003, 3, 1053 CrossRef CAS.
- L. Shen, X. Cui, H. Qi and C. Zhang, J. Phys. Chem. C, 2007, 111, 8172 CrossRef CAS.
- A. J. Bard, Z. Ding and N. Myung, Struct. Bonding (Berlin, Germany), 2005, 118, 1 Search PubMed.
- X. Liu, H. Jiang, J. P. Lei and H. X. Ju, Anal. Chem., 2007, 79, 8055 CrossRef CAS.
- H. Jiang and H. X. Ju, Chem. Commun., 2007, 404 RSC.
- C. Lu, X. F. Wang, J. J. Xu and H. Y. Chen, Electrochem. Commun., 2008, 10, 1530 CrossRef CAS.
- S. N. Ding, J. J. Xu and H. Y. Chen, Chem. Commun., 2006, 3631–3633 RSC.
- T. Ren, J. Z. Xu, Y. F. Tu, S. Xu and J. J. Zhu, Electrochem. Commun., 2005, 7, 5 CrossRef CAS.
- J. J. Miao, T. Ren, D. Lin, J. J. Zhu and H. Y. Chen, Small, 2005, 1, 802 CrossRef CAS.
- G. F. Jie, B. Liu, J. J. Miao and J. J. Zhu, Talanta, 2007, 71, 1476 CrossRef CAS.
- L. H. Zhang, L. Shang and S. J. Dong, Electrochem. Commun., 2008, 10, 1452 CrossRef CAS.
- Y. L. Mei, H. S. Wang, Y. F. Li, Z. Y. Pan and W. L. Jia, Electroanalysis, 2010, 22, 155 CrossRef CAS.
- X. Liu, L. Guo, L. X. Cheng and H. X. Ju, Talanta, 2009, 78, 691 CrossRef CAS.
- H. Y. Han, Z. H. Sheng and J. G. Liang, Anal. Chim. Acta, 2007, 596, 73 CrossRef CAS.
- C. G. Shi, Y. Shan, J. J. Xu and H. Y. Chen, Electrochim. Acta, 2010, 55, 8268 CrossRef CAS.
- X. F. Hu, H. Y. Han, L. J. Hua and Z. H. Sheng, Biosens. Bioelectron., 2010, 25, 1843 CrossRef CAS.
- X. F. Wang, J. J. Xu and H. Y. Chen, J. Phys. Chem. C, 2008, 112, 17581 CrossRef CAS.
- C. G. Shi, J. J. Xu and H. Y. Chen, J. Electroanal. Chem., 2007, 610, 186 CrossRef CAS.
- G. Z. Zou and H. X. Ju, Anal. Chem., 2004, 76, 6871 CrossRef CAS.
- L. J. Hua, J. J. Zhou and H. Y. Han, Electrochim. Acta, 2010, 55, 1265 CrossRef CAS.
- L. J. Hua, H. Y. Han and X. J. Zhang, Talanta, 2009, 77, 1654 CrossRef CAS.
- Y. Wang, J. Lu, L. H. Tang, H. X. Chang and J. H. Li, Anal. Chem., 2009, 81, 9710 CrossRef CAS.
- X. Liu, L. X. Cheng, J. P. Lei and H. X. Ju, Analyst, 2008, 133, 1161 RSC.
- H. Jiang and H. X. Ju, Anal. Chem., 2007, 79, 6690 CrossRef CAS.
- L. J. Hua, H. Y. Han and H. B. Chen, Electrochim. Acta, 2009, 54, 1389 CrossRef CAS.
- G. F. Jie, L. L. Li, C. Chen, J. Xuan and J. J. Zhu, Biosens. Bioelectron., 2009, 24, 3352 CrossRef CAS.
- G. F. Jie, P. Liu, L. Wang and S. S. Zhang, Electrochem. Commun., 2010, 12, 22 CrossRef CAS.
- G. F. Jie, H. P. Huang, X. L. Sun and J. J. Zhu, Biosens. Bioelectron., 2008, 23, 1896 CrossRef CAS.
- G. F. Jie, B. Liu, H. C. Pan, J. J. Zhu and H. Y. Chen, Anal. Chem., 2007, 79, 5574 CrossRef CAS.
- H. P. Huang, G. F. Jie, R. J. Cui and J. J. Zhu, Electrochem. Commun., 2009, 11, 816 CrossRef CAS.
- H. P. Huang and J. J. Zhu, Biosens. Bioelectron., 2009, 25, 927 CrossRef CAS.
- H. P. Huang, Y. L. Tan, J. J. Shi, G. X. Liang and J. J. Zhu, Nanoscale, 2010, 2, 606–612 RSC.
- H. Boaz and G. K. Rollefson, J. Am. Chem. Soc., 1950, 72, 3435 CrossRef CAS.
- A. D. Ellington and J. W. Szostak, Nature, 1990, 346, 818 CrossRef CAS.
- C. Tuerk and L. Gold, Science, 1990, 249, 505 CrossRef CAS.
|
This journal is © The Royal Society of Chemistry 2011 |