DOI:
10.1039/C0AY00505C
(Paper)
Anal. Methods, 2011,
3, 110-115
A novel hydrogen peroxide biosensor based on sol–gel poly (vinyl alcohol) (PVA)/(titanium dioxide)TiO2 hybrid material
Received
17th August 2010
, Accepted 11th September 2010
First published on 15th October 2010
Abstract
A novel hydrogen peroxide biosensor has been fabricated based on Hb entrapped poly(vinyl alcohol) (PVA)/Titanium dioxide (TiO2) hybrid material. Multi-walled carbon nanotubes (MWCNTs) were then dispersed into the composite matrix. It was found that such hybrid material could retain the native biocatalytic activity of the entrapped Hb by electrochemical experiments. In addition, MWCNTs enhanced catalytic performance of hydrogen peroxide and promoted electronic transfer. Effects of some experimental variables such as the amount of MWCNTs, concentration of enzyme, amounts of modifier on the current response of the biosensor were investigated. A linear calibration graph was obtained in the concentration range of H2O2 from 0.5 to 2.7 μM (linear regression coefficient = 0.997) with a detection limit of 0.01 μM (S/N = 3). The apparent Michaelis–Menten constant Km was 0.997 μM. The biosensor displayed excellent repeatability, high sensitivity, long-term stability, and good selectivity. The recovery of H2O2 in samples was testified with satisfactory results.
1. Introduction
Detection of
H2O2 is important in chemical, biological, clinical, environmental, food and industrial analysis. Most of the analytical techniques for measuring hydrogen peroxide (H2O2) such as chromatographic, colorimetric and photometric methods are time-consuming and difficult to adapt to automatic detection.1 Among these, electrochemical method is especially promising because of its simplicity, selectivity, high sensitivity and accurate determination of hydrogen peroxide (H2O2).2–7
Heme
proteins have important physiological functions in living cells which contain the porphyrin complex of iron(II)-heme or iron(III)-hemein as a prosthetic group. Recently, direct electron transfer studies of these proteins have attracted considerable attention,8–14 because fundamental studies of the redox behaviors of these proteins may be of great help to better understand the relationship between their structures and biological functions.15 Haemaglobin (Hb) is regarded as an ideal model molecule for the study of electron transfer between redox proteins and the underlying electrode owing to its known structure, commercial availability and modest cost.16 However, either proteins or enzymes all exhibit rather slow electron transfer rates at conventional electrodes, which may result from the deep burying of electroactive prosthetic groups, unfavorable orientation of proteins on the electrode surface, the adsorption of impurities and denatured protein onto an electrode surface.17,18 In order to achieve the direct electron transfer between the proteins or enzymes and the underlying electrodes, many methods were used to fabricate the third-generation biosensor.8,11,19,20
The immobilization of enzymes is a key step for the preparation of the biosensor. Sol–gel and organic polymer hybrid material can effectively eliminate the brittleness of pure inorganic sol–gel and the swelling property of some pure polymer or hydrogel. Sol–gel physical rigidity, high stability and biocompatibility of organic polymer materials was retained, respectively.21 The polyvinyl alcohol (PVA) is a non-toxic and biocompatible synthetic polymer with good thermal stability as well as good film-forming ability.22–24 PVA is indeed well known for its wide range of potential applications in pharmaceutical, medical, and membrane fields.25 PVA is a polymer composed of a carbon chain backbone attached with hydroxyl groups, which can provide a biocompatible microenvironment for proteins or enzyme immobilization and improve the stability of the modified electrode.26
Meanwhile, many nanosized materials were used to fabricate available protein electrodes and enhance electron transfer10,19,27–30 due to good biocompatibility, high surface activity and perfect uniformity. Carbon nanotubes (CNTs) have unusual electronic properties and many potential applications,31,32 there have been a series of investigations to use CNTs for biosensor interface fabrication.33,34 For example, it can be used as a reaction promoter in biosensors for the electrochemical detection of dopamine,35hydroquinone and catechol,36 and the electrocatalysis of oxygen.37 Recent studies demonstrated that CNTs could promote the electron transfer reactions of proteins including cytochrome c,38myoglobin,39catalase,40hemoglobin,41glucose oxidase,42,43 and horseradish peroxidase (HRP).44–49
TiO2 material is a widely used inorganic material, it shows good biocompatibility, stability and environmental safety, it was used for the immobilization of heme protein and biomaterials in investigating spectroelectrochemical properties or bioanalytical application.12,17,50–53 Xiao et al. reported direct electron transfer and electrocatalysis of hemoglobin on chitosan-TiO2 nanorods-Glass carbon electrode.54 Zheng and his co-workers studied direct electrochemistry and electro-catalysis of hemoglobin immobilized in TiO2 nanotube films.55 Zhang et al. fabricated hydrogen peroxide biosensor based on Mb-TiO2/MWCNTs. The resultant Mb-TiO2/MWCNTs modified glassy carbon electrode exhibited fast amperometric response to hydrogen peroxide reduction, long term life and excellent stability.56
In this study, hybrid material (PVA/TiO2) was prepared by sol–gel process. Furthermore, Hb immobilized in the matrix of multi-walled and PVA/TiO2 film still kept its electrocatalytic reduction to H2O2. The PVA/TiO2 film provided a favorable microenvironment for Hb to exchange electron with the electrode. The experiment results showed that carbon nanotubes enhanced catalytic performance of hydrogen peroxide and promoted electronic transfer. The electrochemical and analytical characteristics of the biosensor were studied. The results showed that the biosensor exhibited a variety of good characteristics including high sensitivity, repeatability, good selectivity, and long-term stability.
2. Experimental
2.1. Apparatus
Electrochemical measurements were performed with CHI660A and CHI440A Electrochemical Station (Shanghai Chenhua Instrument Co., China). The three electrode system consisted of a platinum wire counter electrode, a saturated calomel reference electrode, and the modified glassy carbon electrode (2.0 mm diameter) as working electrode. All electrochemical experiments were carried out in a conventional electrochemical cell holding 6.0 mL of PBS at room temperature. All the electrochemical processes were in aerated solutions.
2.2. Reagents
Hb was obtained from Fluka. MWCNTs (>95% purity) was bought from Cheng du Organic Chemicals Co. Ltd. Chinese Academy of Sciences. Poly (vinyl alcohol) (MW 89000∼98000) were from Sigma-Aldrich. 30% H2O2 was purchased from Guangzhou Chemical (Guangzhou, China). Butyltitanate (TBT) was obtained from TianJin Kermel Chemical Reagents Development Centre. Other reagents were of analytical reagent grade. All solutions were prepared with doubly distilled water. The exact concentration of H2O2 was determined by titration against a standard potassium permanganate solution.
2.3. Preparation of PVA/TiO2 hybrid materials by the sol–gel process
TBT and normal butylalcohol (v/v = 1
:
4) were mixed together in a cuvette. Then the pH of the above solution was adjusted to 2.0 below by adding HCl solution. Uniform and transparent titanium dioxide sol was obtained by stirring for three hours at room temperature. A stock mixture sol–gel titanium dioxide and PVA solution was prepared by mixing 990 μL (PVA aqueous solution of 6%), 10 μL titanium dioxide sol, and in a 2 mL PVC tube. The mixture was sonicated for 30 min until a clear and homogeneous solution resulted. This stock solution was freshly prepared daily just before the fabrication of biosensors.
2.4. Fabrication of PVA/TiO2-MWCNTs-Hb/GCE
A glassy carbon electrode (GCE, 2 mm in diameter) was polished with 1.0, 0.3, and 0.05 mm Al2O3 slurry successively followed by rinsing thoroughly with doubly deionised water until a mirror-like surface was obtained. Then it was sonicated in 1
:
1 nitric acid, absolute ethanol and doubly distilled water, each for 5 min, and allowed to dry at room temperature. A sample of Hb (15 mg) was dissolved in 1 mL of 0.12 M PBS (pH = 6.2). The Hb stock solution was stored at 4 °C in a refrigerator when not in use. A 2.5 mg MWCNTs dispersion was prepared by dissolving MWCNTs in 1 mL doubly distilled water with the help of 30 min ultrasonic agitation. A 70 μL PVA/TiO2 hybrid materials, 30 μL MWCNTs dispersion and Hb (15 mg mL−1) with the volume of 25 μL in a 2.0 mL PVC tube was mixed until a clear and homogeneous solution resulted. A drop of this dispersion with the volume of 4 μL was casted onto the surface of GCE, then it was dried in air for 3 h.
3. Results and discussion
3.1. Electrochemical characteristics of the biosensors
Fig. 1 showed the typical cyclic voltammograms of different electrodes in pH 6.2 PBS at scan rate 50 mV s−1. No electrochemical responses were observed at (a) PVA/GCE (c) PVA/TiO2/GCE, (e) PVA/TiO2- MWCNTs/GCE, which indicated no electroactive substances existed on the electrode surface. While on the PVA/Hb/GCE only an irreversible reduction peak was observed (curve b), which can be explained as only the few Hb molecules closest to the electrode could exchange electrons with the electrode and contribute to the observed redox reaction. For PVA/TiO2-Hb/GCE electrode (curve d), only a pair of small, unsymmetric redox peaks were observed, which indicated that the direct electron transfer of Hb with GCE had taken place with slow electron transfer rate. The peak current of PVA/TiO2-Hb/GCE (curve d) was much higher than that of PVA/Hb/GCE (curve b), that is to say, more electrons of Hb entrapped in PVA/TiO2 film participated in the electrochemical reaction. The PVA/TiO2 film provided a favorable microenvironment for Hb to exchange electron with the electrode. While on the (f) PVA/TiO2-Hb-MWCNTs/GCE, the electrochemical response of Hb was greatly enhanced and a pair of well-defined quasi-reversible cyclic voltammetric peaks were observed (curve f), which revealed that MWCNTs played an important role in facilitating the direct electron transfer of Hb by the synergism with PVA/TiO2 film.
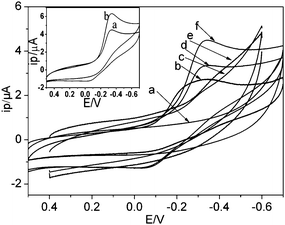 |
| Fig. 1
Cyclic voltammograms of (a) PVA/GCE, (b) PVA/Hb/GCE, (c) PVA/TiO2/GCE, (d) PVA/TiO2-Hb/GCE, (e) PVA/TiO2-MWCNTs/GCE and (f) PVA/TiO2-Hb-MWCNTs/GCE in 0.12 M pH 6.2 PBS scan rate 50 mV s−1. Inset: cyclic voltammogram of (a) PVA/TiO2-Hb-MWCNTs/GCE in 0.12 M pH 6.2 PBS at scan rate 50 mV s−1, (b) PVA/TiO2-Hb-MWCNTs/GCE in 0.12 M pH 6.2 PBS with 1.0 μM H2O2 at scan rate 50 mV s−1. | |
To explore the potential application of PVA/TiO2-Hb-MWCNTs/GCE, the electrocatalytic reduction of H2O2 on the electrode had been studied. The PVA/TiO2-Hb-MWCNTs/GCE showed good electrocatalytic ability to H2O2. As shown in Fig. 1 Inset, with the addition of H2O2 into 0.12 M pH 6.2 PBS an obvious increase of the cathodic peak current with the decrease of the anodic peak current (curves a, b), which indicated the Hb incorporated in the composite film could act as an effective catalyst to the reduction of H2O2.
The CVs at different scan rates can be seen from Fig. 2 both anodic and cathodic peak currents increased linearly with the square root of the scan rates in the range from 10 to 600 mV s−1, which indicated that a diffusion-controlled process occurred at the PVA/TiO2-MWCNTs-Hb/GCE.
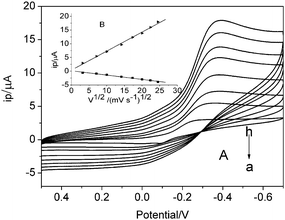 |
| Fig. 2 The cyclic voltammograms of PVA/TiO2-MWCNTs-Hb/GCE with different scan rates: a) 10, b) 50, c) 100, d) 200, e) 300, f) 400, g) 500, h) 600, mV s−1 in 0.12 M pH 6.2 PBS containing 1.0 μM H2O2, (inset B) the linear relation between peak current and v1/2. | |
EIS is a powerful tool for studying the interface properties of surface modified electrodes. The electron transfer resistance (RCT) at the electrode surface is an important parameter. Fig. 3 illustrated the typical results of AC impedance spectra of the (a) bare GCE, (b) PVA/TiO2-Hb/GCE, (c) PVA/TiO2-MWCNTs-Hb/GCE, respectively. Obviously, the whole profile for (b) PVA/TiO2-Hb/GCE exhibited the greatest semicircle, which indicated the PVA/TiO2 hybrid material impeded electron transfer of the electrochemical probe. However, the curve (c) PVA/TiO2-MWCNTs-Hb/GCE exhibited much smaller semicircle compared with (a) bare GCE and (b) PVA/TiO2-Hb/GCE, which showed that the presence of MWCNTs could accelerate the electron transfer.
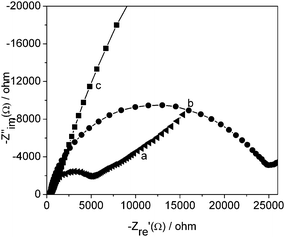 |
| Fig. 3
Electrochemical impedance spectroscopy (a) bare GCE (b) PVA/TiO2-Hb/GCE, (c) PVA/TiO2-MWCNTs-Hb/GCE in the presence of a solution 5.0 × 10−3 M K3Fe(CN)6/K4Fe(CN)6 (1 : 1) containing 0.1 M KCl. | |
3.3. Optimization of experimental conditions
Carbon nanotubes can largely promote the electron transfer of Hb, so the volume of MWCNTs solution is an important factor of the performance of the biosensor. In order to determine the optimal volume of MWCNTs casted on the electrode, a plot of cathodic currents versus amounts of MWCNTs was made as shown in Fig. 4. The cathodic peak current achieved its maximum value when the amount of MWCNTs was 0.5 mg. Because too much amounts of MWCNTs will agglomerate on the electrode surface and decrease the stability of the sensing surface. Moreover, the electron transfer will become difficult if amounts of MWCNTs was 0.5% below. Obviously, the optimal amount of MWCNTs was 0.5 mg. These results indicated that the conductivity of MWCNTs might not be able to extend to the others especially when the nanotubes were packed randomly and disorderly, thus limiting the effect of MWCNTs in improving the protein electron transport.
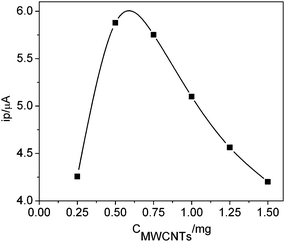 |
| Fig. 4 Effect of MWCNTs amount on the biosensor responses at 50 mV s−1 in 0.12 M PBS (6.2) containing 1.0 μM H2O2. | |
The concentration of enzyme in composite is a vital factor affecting the biosensor response. Fig. 5 displayed the cathodic peak current response of the biosensor with different concentrations of Hb-loading. The cathodic peak current arrived at its peak value, when the Hb concentration on electrode was 15 mg mL−1. So an optimal loading of 15 mg mL−1Hb was used for subsequent experiment.
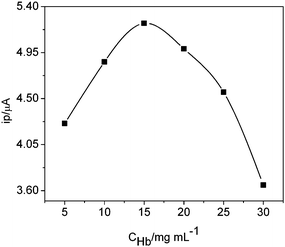 |
| Fig. 5 Effect of Hb concentration on cathodic peak current of the enzyme electrode in 0.12 M PBS (6.2) at 50 mV s−1 containing 1.0 μM H2O2. | |
The effect of amounts of modifier on the catalytic current for H2O2 oxidation was also studied. Fig. 6 displayed the response of the electrode containing different amounts of modifier. The response current increased along with increased amounts of modifier. The maximum response was obtained with 4 μL. The electron transfer will become difficult if modifier was casted on the electrode surface too much. So amounts of modifier of 4 μL was used for the subsequent experiments to obtain the greatest sensitivity.
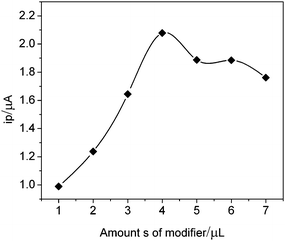 |
| Fig. 6 Effect of amounts of modifier on the electrode response in 0.12 M pH 6.2 PBS containing 1.0 μM H2O2. | |
3.4. The linear range and the detection limit of electrode
Fig. 7 showed the typical response of the biosensor for successive additions of H2O2 under the optimized experimental conditions. The linear range spans the concentration of H2O2 from 0.5 to 2.7 μM with a correlation coefficient of 0.997. The regression equation was i (μA) = 1.47 + 0.18 C (μM). The detection limit of this biosensor was estimated as 0.01 μM at a signal to noise ratio of 3.
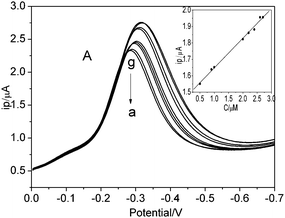 |
| Fig. 7 (A) Differential pulse voltammograms of biosensor (a) in the absence and (b–g) successive addition of 1.0 × 10−4 M H2O2, (inset) calibration curve of biosensor response with H2O2 concentration. | |
The apparent Michaelis–Menten constant (Km) is a reflection of the enzymatic affinity. The apparent Michaelis–Menten constant of immobilized enzyme was obtained according to (Eq. 1)57
where
Iss = steady state current after the addition of substrate,
C = bulk concentration of the substrate,
Imax = maximum current measured under saturated substrate condition. According to this equation,
Km was calculated to be 0.161 μM. This value was lower than the literature
58–61 reported (2.21 μM, 0.68 mM, 0.75 mM, 0.898 mM), which indicated the higher enzymatic activity of immobilized Hb. Thus the above result further showed that the PVA/TiO
2-MWCNTs-Hb modified biosensor possessed a high affinity to H
2O
2. This is because polyvinyl alcohol and titanium dioxide hybrid materials can well maintain the catalytic activity of the enzyme. This film was an excellent carrier for immobilized enzyme (see
Table 1).
Table 1
Peroxidase-like activity of some Hb filmsa
3.5. Biosensor repeatability and stability
The repeatability of response current of the Hb electrode was investigated at H2O2 concentration of 1.0 μM. The variation coefficient (DPV) was 3.68% for eight successive assays. Five Hb electrodes, made independently, showed an acceptable reproducibility with a variation coefficient of 6.92% (n = 5) for the current determination at 1.0 μM H2O2.
The biosensor was stored in a refrigerator at 4 °C when not used. The stability of the biosensor under storage was investigated by measuring the biosensor response with 1.0 μM of H2O2 everyday. The corresponding result was shown in Fig. 8. Δi is the different current of the enzyme electrode after storage. In the figure, only 2% loss of the response current could be observed within 5 days. After 20 days, the biosensor retained about 90% of its original response. The stability of the biosensor is much better than that of the previous biosensor.61 Good stability can be attributed to the following factors: the Hb was physically embedded in the hydrogel; large quantities of hydroxyland amino groups in the PVA, which were favorable in maintaining the activity of the Hb, could form strong interactions between the Hb and the hydrogel; the enzymes were embedded temperately in the sol–gel network, which could more effectively immobilize Hb.
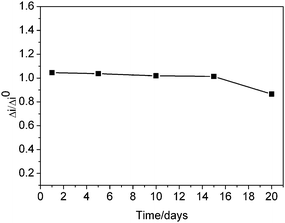 |
| Fig. 8 Stability of enzyme electrode stored at 4 °C in 0.12 M pH 6.2 PBS containing 1.0 μM H2O2. | |
3.6. Interference
Selectivity is an important factor in the performance of sensor. Several substances were measured to examine whether they interfered the determination of H2O2. Table 2 displays minimum concentration of the interferent that provides an error ±5% to the response current of 1.0 μM H2O2. In our experiments, the tested substances did not interfere significantly with the resulting biosensor.
Table 2 Possible interference tested with the biosensor
Interferences |
Minimum concentration/mM of the interferents that provides an error ±5% to the response current of 1.0 μM H2O2 |
Fructose
|
1.14 |
L-Cysteine
|
1.64 |
L-Lysine
|
1.20 |
Tyrosine
|
1.32 |
Cl
− |
1.21 |
NH4+ |
1.21 |
Ca+ |
1.18 |
3.7. Analytical application of the biosensor
The accuracy of the biosensor was evaluated by determining the recoveries of hydrogen peroxide in a disinfectant using a standard addition method with the recovery ranging from 96.75% to 115% (see Table 3).
Table 3 Determination results of recovery using the present biosensor
S.NO. |
Added H2O2/μM |
Found H2O2/μMa |
Recovery (%) |
Average of five measurements.
|
1 |
1.0 |
1.115 |
115 |
2 |
1.5 |
1.591 |
106 |
3 |
2.0 |
1.935 |
96.75 |
4. Conclusions
A Novel PVA/TiO2 organic-inorganic hybrid material has been prepared by the sol–gel process with biocompatibility and network structure. Such a hybrid material could retain the native biocatalytic activity of the entrapped Hb. Carbon nanotubes can promote direct electron transfer of Hb immobilized onto the surface of GCE. We have successfully developed a mediator-free, third-generation H2O2 biosensor, which exhibited a variety of good electrochemical characteristics including reproducibility, high sensitivity, good long-term stability. The method presented here not only can be easily extended to immobilized and obtain direct electrochemistry of other proteins, but also can be used for two-enzyme systems. It showed that the films might be useful for practical application in fabricating biosensors or bioreactors based on the direct electron transfer of protein without using mediators.
Acknowledgements
This work was supported by National Natural Science Foundation of China (No. 21065001), and the Natural Science Foundation of Guangxi Zhuang Autonomous Region (No. 0639025).
References
- C. Guzmán, G. Orozco, Y. Verde, S. Jiménez, Luis A. Godínez, E. Juaristi and E. Bustos, Electrochim. Acta, 2009, 54, 1728 CrossRef CAS.
- N. Q. Jia, Q. Zhou, L. Liu, M. M. Yan and Z. Y. Jiang, J. Electroanal. Chem., 2005, 580, 213 CrossRef CAS.
- J. Li, L. T. Xiao, X. M. Liu, G. M. Zeng, G. H. Huang, G. L. Shen and R. Q. Yu, Anal. Bioanal. Chem., 2003, 376, 902 CAS.
- X. Xu, B. Z. Tian, S. Zhang, J. L. Kong, D. Y. Zhao and B. H. Liu, Anal. Chim. Acta, 2004, 519, 31 CrossRef CAS.
- T. Tangkuaram, C. Ponchio, T. Kangkasomboon, P. Katikawong and W. Veerasai, Biosens. Bioelectron., 2007, 22, 2071 CrossRef CAS.
- T. Tatsuma and T. Watanabe, Anal. Chem., 1991, 63, 1580 CrossRef CAS.
- X. L. Luo, J. J. Xu, Q. Zhang, G. J. Yang and H. Y. Chen, Biosens. Bioelectron., 2005, 21, 190 CrossRef CAS.
- S. F. Ding, W. Wei and G. C. Zhao, Electrochem. Commun., 2007, 9, 2202 CrossRef CAS.
- L. Duan, Q. He, X. H. Yan, Y. Cui, K. W. Wang and J. B. Li, Biochem. Biophys. Res. Commun., 2007, 354, 357 CrossRef CAS.
- G. Y. Shi, Z. Y. Sun, M. C. Liu, L. Zhang, Y. Liu, Y. H. Qu and L. T. Jin, Anal. Chem., 2007, 79, 3581 CrossRef CAS.
- R. Yan, F. Q. Zhao, J. W. Li, F. Mao, S. S. Fan and B. Z. Zeng, Electrochim. Acta, 2007, 52, 7425 CrossRef CAS.
- W. Zheng, Y. F. Zheng, K. W. Jin and N. Wang, Talanta, 2008, 74, 1414 CrossRef CAS.
- S. Q. Liu, Z. H. Dai, H. Y. Chen and H. X. Ju, Biosens. Bioelectron., 2004, 19, 963 CrossRef CAS.
- Y. Liu, J. P. Lei and H. X. Ju, Talanta, 2008, 74, 965 CrossRef CAS.
- F. A. Armstrong, J. Chem. Soc., Dalton Trans., 2002, 661 RSC.
- Q. L. Wang, G. X. Lu and B. J. Yang, Biosens. Bioelectron., 2004, 19, 1269 CrossRef CAS.
- Q. W. Li, G. A. Luo and J. Feng, Electroanalysis, 2001, 13, 359 CrossRef CAS.
- J. M. Xu, W. Li, Q. F. Yin, H. Zhong, Y. L. Zhu and L. T. Jin, J. Colloid Interface Sci., 2007, 315, 170 CrossRef CAS.
- S. J. Guo and E. K. Wang, Anal. Chim. Acta, 2007, 598, 181 CrossRef CAS.
- G. Z. Liu, M. N. Paddon-Row and J. J. Gooding, Electrochem. Commun., 2007, 9, 2218 CrossRef CAS.
- B. Yan, L. Zhao and X. Tan, Chinese Journal of Sensors and Actuators, 2008, 21, 1314 Search PubMed.
- C. Y. Yang, Y. G. Tao, Q. Wang and B. Tang, Journal of Anhui University of Technology and Science, 2008, 23, 7 Search PubMed.
- J. Kumar and S. F. D'Souza, Talanta, 2008, 75, 183 CAS.
- S. Majumdar and B. Adhikari, Sens. Actuators, B, 2006, 114, 747 CrossRef.
- S. Clemenson, L. David and E. Espuche, J. Polym. Sci., Part A: Polym. Chem., 2007, 45, 2657 CrossRef CAS.
- W. Sun, X. Q. Li, Y. Wang, R. J. Zhao and K. Jiao, Electrochim. Acta, 2009, 59, 4142.
- C. X. Cai and J. Chen, Anal. Biochem., 2004, 325, 285 CrossRef CAS.
- J. M. Gong and X. Q. Lin, Chin. J. Chem., 2003, 21, 761 CAS.
- H. L. Qi, C. X. Zhang and X. R. Li, Sens. Actuators, B, 2006, 114, 364 CrossRef.
- Z. W. Zhao, X. J. Chen, B. K. Tay, J. S. Chen, Z. J. Han and K. A. Khor, Biosens. Bioelectron., 2007, 23, 135 CrossRef CAS.
- D. Christopher and M. T. James, J. Am. Chem. Soc., 2003, 125, 1156 CrossRef CAS.
- W. Zhao, C. Song and P. E. Pehrsson, J. Am. Chem. Soc., 2002, 124, 12418 CrossRef CAS.
- J. Wang, M. Musameh and Y. Lin, J. Am. Chem. Soc., 2003, 125, 2408 CrossRef CAS.
- Y. Lin, F. Lu, Y. Tu and Z. F. Ren, Nano Lett., 2004, 4, 191 CrossRef CAS.
- J. J. Davis, R. J. Coles, H. Allen and O. Hill, J. Electroanal Chem., 1997, 440, 279 CrossRef CAS.
- H. Qi and C. Zhang, Electroanalysis, 2005, 17, 832 CrossRef CAS.
- P. J. Britto, K. S. V. Santhanam, A. Rubio, J. A. Alonso and P. M. Ajayan, Adv. Mater., 1999, 11, 154 CrossRef CAS.
- J. X. Wang, M. X. Li, Z. J. Shi, N. Q. Li and Z. N. Gu, Anal. Chem., 2002, 74, 1993 CrossRef CAS.
- G. C. Zhao, L. Zhang, X. W. Wei and Z. S. Yang, Electrochem. Commun., 2003, 5, 825 CrossRef CAS.
- L. Wang, J. X. Wang and F. M. Zhou, Electroanalysis, 2004, 16, 627 CrossRef CAS.
- Y. D. Zhao, Y. H. Bi, W. D. Zhang and Q. M. Luo, Talanta, 2005, 65, 489 CrossRef CAS.
- Y. Q. Dai and K. K. Shiu, Electroanalysis, 2004, 16, 1697 CrossRef CAS.
- C. X. Cai and J. Chen, Anal. Biochem., 2004, 332, 75 CrossRef CAS.
- J. Z. Xu, J. J. Zhu, Q. Wu, Z. Hu and H. Y. Chen, Electroanalysis, 2003, 15, 219 CrossRef.
- X. Yu, D. Chattopadhyay, I. Galeska, F. Papadimitrakop-oulos and J. F. Rusling, Electrochem. Commun., 2003, 5, 408 CrossRef CAS.
- X. L. Luo, A. J. Killard, A. Morrin and M. R. Smyth, Anal. Chim. Acta, 2006, 575, 39 CrossRef CAS.
- V. S. Tripathi, V. B. Kandimalla and H. X. Ju, Biosens. Bioelectron., 2006, 21, 1529 CrossRef CAS.
- Y. D. Zhao, W. D. Zhang, H. Chen, Q. M. Luo and S. F. Y. Li, Sens. Actuators, B, 2002, 87, 168 CrossRef.
- C. X. Cai and J. Chen, Acta. Chimi. Sin., 2004, 62, 335 Search PubMed.
- A. Curulli, A. Cusma, S. Kaciulis, G. Padeletti, L. Pandolfi, F. Valentini and M. Viticoli, Surf. Interface Anal., 2006, 38, 478 CrossRef CAS.
- H. Huang, N. F. Hu, Y. H. Zeng and G. Zhou, Anal. Biochem., 2002, 308, 141 CrossRef CAS.
- A. H. Liu, M. D. Wei, I. Honma and H. S. Zhou, Anal. Chem., 2005, 77, 8068 CrossRef CAS.
- X. Quan, S. G. Yang, X. L. Ruan and H. M. Zhao, Environ. Sci. Technol., 2005, 39, 3770 CrossRef CAS.
- X. L. Xiao, W. Lu and X. Yao, Electroanalysis, 2008, 20, 2247 CrossRef CAS.
- W. Zheng, Y. F. Zheng, K. W. Jin and N. Wang, Talanta, 2008, 74, 1414 CrossRef CAS.
- L. Zhang, D. B. Tian and J. J. Zhu, Bioelectrochemistry, 2008, 74, 157 CrossRef CAS.
- F. R. Shu and G. S. Wilson, Anal. Chem., 1976, 48, 1684.
- Ansari, P. R. Solanki and B. D. Malhotra, J. Biotechnol., 2009, 142, 179 CrossRef CAS.
- H. Y. Xiong, T. Chen, X. H. Zhang and S. F. Wang, Electrochem. Commun., 2007, 9, 2671 CrossRef CAS.
- D. Shan, S. X. Wang, H. G. Xue and S. Cosnier, Electrochem. Commun., 2007, 9, 529 CrossRef CAS.
- Q. L. Wang, G. X. Lu and B. J. Yang, Biosens. Bioelectron., 2004, 19, 1269 CrossRef CAS.
- Y. D. Zhao, Y. H. Bi, W. D. Zhang and Q. M. Luo, Talanta, 2005, 65, 489 CrossRef CAS.
- S. Liu, Z. Dai, H. Chen and H. Ju, Biosens. Bioelectron., 2004, 19, 963 CrossRef CAS.
- Y. Liu, C. Lu, W. Hou and J. Zhu, Anal. Biochem., 2008, 375, 27 CrossRef CAS.
- W. Sun, D. D. Wang, R. F. Gao and K. Jiao, Electrochem. Commun., 2007, 9, 1159 CrossRef.
- W. Sun, X. Q. Li, Y. Wang, R. J. Zhao and K. Jiao, Electrochim. Acta, 2009, 54, 4141 CrossRef CAS.
|
This journal is © The Royal Society of Chemistry 2011 |