DOI:
10.1039/C0SC00396D
(Edge Article)
Chem. Sci., 2010,
1, 716-722
A benzocrown-6-calix[4]arene methacrylate copolymer: Selective extraction of caesium ions from a multi-component system†
Received
29th July 2010
, Accepted 20th September 2010
First published on
19th October 2010
Abstract
The synthesis and extraction properties of a copolymer comprised of a 10:1 molar ratio of methyl methacrylate (MMA) and a methacrylate functionalized benzocrown-6-calix[4]arene are reported. Under aqueous-dichloromethane liquid–liquid extraction conditions, the copolymer displayed an enhanced selectivity for caesium over sodium and potassium (in the form of their picrate salts) as compared to an appropriate small molecule analogue. Further, the copolymer was capable of removing caesium nitrate from aqueous solution in the presence of various other anionic (e.g., F−, Cl−, and SO4−) and cationic species (e.g., K+ and Na+). The ability of this polymeric system to extract inorganic caesium salts into dichloromethane from aqueous media scaled with the relative hydration energies (ΔhydG°) of each anion studied (i.e., NO3− > Br− ≫ Cl− or F−).
Introduction
Calixarenes are versatile molecular receptors that have greatly impacted the field of host–guest chemistry.1 Their macrocyclic structure combined with their inherent flexibility affords binding pockets capable of recognizing specifically alkali metal ions and other guest molecules. There has been extensive research efforts aimed at incorporating these dynamic receptors into materials such as solid supports,2 side chain,3 and main chain polymers.4 Moreover, the ability of the resulting materials to complex cationic species has been applied to a diverse range of applications involving purification5 and sensing,6 and the development of ion selective electrodes.7
Separately, advances in calixarene chemistry have led to the development of calixarenes with crown ether rings appended to their framework, known as calixcrowns.8 Over the last decade these specialized supramolecular receptors have received significant attention for their role in the selective extraction of alkali metal cations.9 In particular, crown-6-calix[4]arenes, such as benzocrown-6-calix[4]arene 1,10 have been recognized for their ability to bind and extract Cs+ salts from aqueous media.11 Moreover, systems analogous to 1 can be designed to extract Cs+ with selectivity over other cations, including Na+.8f,11b,12 and K+.11b,12b,13 This selectivity is of great importance when removing Cs+ from contaminated ground water and neutralized reprocessing waste. For example, related efforts have led to the development of receptors, such as calix[4]arene-bis(t-ocylbenzo-crown-6),14 which are highly efficient in the removal of Cs+ from nuclear waste.13c,15 However, given this success it is interesting to note that only a few reports have addressed the effect of incorporating these selective receptors into polymeric materials.16 To date most of these studies have involved embedding16f,17 calixcrowns in, or attaching them to, preformed polymer supports.16a Further, in most cases, the associated studies have not involved calixcrown receptors that were designed to bind caesium ions selectively.16b,c,d,g We were thus keen to explore whether an appropriately designed polymeric system could be synthesized that incorporates a caesium selective crown-6-calix[4]arene receptor and, if successful, to investigate how the polymer backbone influences the inherent selectivity and extraction properties.
Recent work from our laboratories described a general strategy for preparing polymethyl methacrylate (PMMA) polymers with neutral molecular receptors as side-chains.18 In these initial studies, copolymers containing calix[4]pyrroles (4) were found to be useful for the extraction of certain salts from aqueous media. However, these copolymers were not designed to recognize Cs+. Accordingly, we have now prepared a new copolymer (3) comprised of a benzocrown-6-calix[4]arene containing monomer (2) and methyl methacrylate (MMA) (Fig. 1). As detailed below, this system is capable of extracting the Cs+ cation from aqueous media with a selectivity over K+ and Na+ that exceeds that displayed by the constituent (monomeric) calixcrown receptor (i.e., 1). It was further found that precipitation of the salt-bound form of polymer 3, followed by polymer “digestion” under oxidizing acidic conditions, could be used to isolate the extracted caesium species. Finally, the anion dependence of the Cs+ cation recognition process under biphasic aqueous-dichloromethane conditions was studied in the case of 3. In accord with what would be expected based on the Hofmeister bias,19 this selectivity was found to scale with the relative hydration energies (ΔhydG°) of the anions investigated (i.e., NO3− > Br− ≫ Cl− or F−).
Results and discussion
Synthesis
Monomer 2 was selected because it can be accessed readily, a characteristic we considered predicative to a comparative study of the type involved in this report. The strategy used to prepare monomer 2 is outlined in Scheme 1. Briefly, dialkylation of known20 calix[4]arene 5 with ethyl bromoacetate under basic conditions afforded the desired diester 6 in 86% isolated yield.21 This first reaction in the synthetic sequence proceeded with high regioselectivity, a finding that is believed to stem from deactivation of the neighboring phenol moieties relative to the first site of alkylation. This feature facilitated access to formylated benzocrown-6-calix[4]arene 7 in 64% yield via deprotonation of 6 with CsCO3 followed by substitution of the known22 benzaldehyde polyether 8.11a,23 Finally, reduction of the aforementioned product using NaBH4 followed by condensation with methacryloyl chloride afforded 2 in 90% yield (over two steps).
![Synthesis of benzocrown-6-calix[4]arene monomer 2 and coploymer 3.](/image/article/2010/SC/c0sc00396d/c0sc00396d-s1.gif) |
| Scheme 1 Synthesis of benzocrown-6-calix[4]arene monomer 2 and coploymer 3. | |
With 2 in hand, efforts were next directed toward the synthesis of copolymer 3. A tetrahydrofuran solution of 2 and MMA in a 1
:
10 molar ratio, respectively, was treated with azoisobutyronitrile (AIBN, 1 mol%) and then heated at 70 °C for 18 h. The resulting solution was then cooled to ambient temperature and was added drop-wise into excess cold methanol. The desired copolymer precipitated from solution, and was isolated in 75% yield via gravity filtration. Proton NMR spectroscopic analysis of 3 confirmed that 2 and MMA were successfully copolymerized in a random fashion and in a 1: 10 molar ratio (i.e., x = 1 and y = 10; Scheme 1). The formation of a random copolymer was further supported by gel permeation chromatography, which revealed that 3 exhibited a monomodal chromatogram that corresponded to a polystyrene equivalent weight average molecular weight (Mw) of 28 kDa and a polydispersity index (PDI) of 2.0; these results are typical of free radical polymerizations and provide support for the proposed structure (Fig. 2A).24 The thermal stability of 3 was similar to that exhibited by PMMA, with an initial onset of thermal decomposition being observed at 260 °C (Fig. 2B).
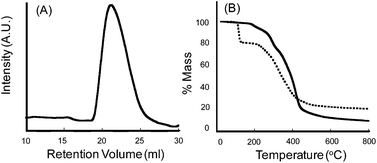 |
| Fig. 2 (A) Gel permeation chromatogram of copolymer 3; conditions: DMF as the eluent, 40 °C, 10 mL min−1. Mw = 28 kDa, PDI = 2.0 (relative to polystyrene standards). (B) Thermogravigrams of copolymer 3 (solid line) and copolymer 3 after exposure to CsNO3 (dotted line) (see text for additional details); conditions: nitrogen atmosphere, temperature ramp rate = 10 °C min−1. | |
In a preliminary experiment designed to assess qualitatively the extraction properties of 3, an aqueous solution of Cs+ picrate ([Cs+ picrate]0 = 1 mM) was vigorously mixed for 30 min with a CH2Cl2 solution of the aforementioned copolymer (the effective initial concentration of the receptor subunit, [calix[4]arene]0, was 6 mM). Upon subsequent separation via centrifugation, the organic phase displayed the distinctive yellow color characteristic of the picrate anion (Fig. 3; vial A). This result was taken as an indication that caesium picrate had been successfully extracted from the aqueous layer. Further support for this contention came from a series of control experiments. As a first positive control experiment, the benzocrown-6-calixarene 1 ([1]0 = 6 mM) was tested as an extractant. Under extraction conditions identical to those used in the case of 3, the yellow color characteristic of picrate was observed with this receptor (vial B). As negative controls, the extraction capabilities of a copolymer 4 ([calix[4]pyrrole]0 = 6 mM; vial C), PMMA homopolymer ([monomer]0 = 60 mM; vial D), and dichloromethane (vial E) were also tested under similar conditions. As shown in Fig. 3, no color was observed in the organic phase in the case of vials D or E, and only a faint yellow color was seen in the case of vial C.25 These results are consistent with a system that necessitates a calix[4]arene (e.g., 1 or 3) to facilitate efficient extraction of caesium picrate.
The extraction efficiency of copolymer 3 was quantified using UV-Vis spectroscopy. Extraction experiments similar to those described above were performed by exposing 3 mL of aqueous caesium picrate ([caesium picrate]0 = 0.02 mM) to 1 mL of solutions of 1, 3, 4, and PMMA (these solutions were prepared at 6 mM initial concentrations based on the calix[4]arene or calix[4]pyrrole repeat units, respectively, in the aforementioned polymers except for PMMA which was prepared at 60 mM based on its repeat unit) in CH2Cl2. After phase separation, the aqueous layers were isolated and analyzed. The percent extraction efficiency, expressed as %E, was calculated using eqn (1), where [M+ Pic−]0 and [M+ Pic−] are the concentrations of caesium picrate before and after extraction, respectively. As summarized in Table 1 and in accord with our initial qualitative observations (vide supra), no evidence of salt extraction was seen in the case of the control systems lacking a calix[4]arene subunit. However, copolymer 3 and its small molecule analogue14b1 both proved capable of facilitating the extraction of caesium picrate from aqueous media.
| 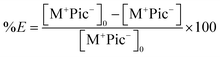 | (1) |
Table 1 Summary of liquid–liquid extraction data obtained after exposing aqueous solutions of metal picrate salts to dichloromethane solutions of 1, 3, 4, and PMMA.a
Extractantb |
Cs+ picrate (%E) |
Na+ picrate (%E) |
K+ picrate (%E) |
αCs/Nac |
αCs/K |
[Metal picrate]0 = 0.020 mM in the case of Cs+ picrate and [Metal picrate]0 = 0.019 mM in the case of Na+ and K+ picrate. The extraction efficiencies (%E) were determined according to eqn (1). The error values given represent the deviations seen in replicate (at least duplicate) measurements.
The concentrations in CH2Cl2 are listed in parenthesis and refer to the initial concentration of calix[4]arene moieties (1 and 3), the initial concentration of the calix[4]pyrrole moieties (4), or the initial concentration of the repeat units in PMMA.
The selectivity factor αCs/M refers to the ratio of the respective %E values, where M = Na or K.
|
1 (6 mM) |
23.65 ± 2.40 |
2.10 ± 1.00 |
22.60 ± 2.30 |
11 |
1.0 |
3 (6 mM) |
23.65 ± 2.30 |
0.10 ± 0.05 |
6.80 ± 0.70 |
236 |
3.5 |
4 (6 mM) |
3.40 ± 1.00 |
— |
— |
— |
— |
PMMA (60 mM) |
0.00 ± 1.00 |
— |
— |
— |
— |
The cation selectivity of copolymer 3 and its small molecule analogue 1 was examined by testing their abilities to extract other metal picrate salts, specifically sodium picrate and potassium picrate. Under conditions analogous to those described above, small molecule analogue 1 was found to effectively extract potassium picrate from the aqueous phase (%E = 22.6), but not sodium picrate (%E = 2.10). In contrast, the extraction efficiency calculated for copolymer 3 was significantly lower and appeared to be restricted to potassium picrate (%E = 6.80) (cf. Table 1). Based on these figures, copolymer 3 displayed a selectivity factor (α) of 236 for Cs+versus Na+, whereas the corresponding selectivity factor of its small molecule analogue (1) was 11 (cf. Table 1). The selectivity values found here are less than other optimized systems reported in the literature.11b,12b,13c However, our prototype demonstrates that in this instance covalent attachment of receptor 1 to PMMA increases the selectivity characteristics of this particular test receptor. We speculate that the ester linkages on the MMA backbone limit access to the calixarene thus reducing non-specific binding modes, such as “outside binding” to the crown subunit, that would otherwise be expected to decrease the specificity.
A series of competition studies provided further evidence for the selective nature of copolymer 3 (Fig. 4). In these experiments, CH2Cl2 solutions of both extractants (i.e., 3 and 1; [calix[4]arene]0 = 6 mM) were first exposed to an aqueous solution of sodium picrate (0.6 mM). Upon separation, the organic layers were found to be only slightly yellow in color (Fig. 5A and C), leading us to suggest that very little phase transfer of the picrate anion (and its sodium counter cation) had occurred. Caesium chloride (2 equiv. relative to sodium picrate) was then added to each of the aforementioned aqueous phases. In the case of the extraction experiment performed using copolymer 3, the addition of CsCl resulted in the complete transfer of the yellow color attributed to the picrate anion to the organic phase (Fig. 5, Vial B).26 In comparison, a faint yellow color was observed in the aqueous layer of the experiment involving 1 (Fig. 5, Vial D). Such results are consistent with small molecule 1 being a less selective Cs+ extractant than copolymer 3.
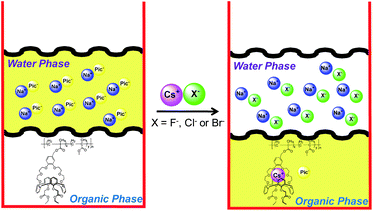 |
| Fig. 4 Schematic representation of a competition experiment designed to test the selectivity of copolymer 3. See text for further details. | |
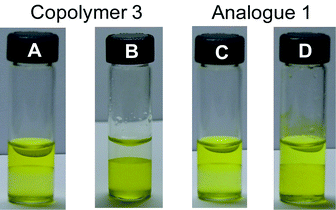 |
| Fig. 5 Photographs of vials showing the results of competition experiments. In these studies, copolymer 3 and analogue 1 (vials C and D) in CH2Cl2 (bottom layer) were first exposed to an aqueous solution (top layer) of sodium picrate (0.6 mM) and then caesium chloride (2 equiv. relative to sodium picrate) was directly added to the mixture. This resulted in complete or partial transfer of the color ascribed to the picrate anion to the bottom layer (vials B and D). | |
To explore further the ability of copolymer 3 to effect the extraction of the caesium cation in the presence of various inorganic salts, a series of 1H NMR extraction studies were carried out. Here, 1H NMR spectroscopic techniques were used to monitor the spectral changes of copolymer 3 in CD2Cl2 ([calix[4]arene]0 = 6 mM) upon exposure to aqueous solutions of inorganic caesium salts (viz., CsF, CsCl, CsBr, CsNO3, CsSO4, KNO3 and NaNO3) at initial concentrations that ranged from 0–200 mM.27 The greatest spectral response (i.e., the largest change in chemical shifts) was observed upon exposure of 3 to aqueous CsNO3. For example, inspection of Fig. 6 revealed that the signals attributed to the calix[4]arene (i.e., δ = 6.6–7.5 ppm) and the crown ether bridge (i.e., δ = 3.4–4.2 ppm) were shifted to lower fields as the aqueous CsNO3 concentration increased and were in agreement with previously reports that explored Cs+ complexation with other calix[4]arene-crowns.28 In contrast, less pronounced downfield changes in chemical shifts were observed when CsBr was added under identical biphasic conditions and no significant response was observed upon exposure of 3 to aqueous solutions of CsF, CsCl, or CsSO4.† In a separate set of extraction studies, conducted under analogous conditions, but using KNO3 and NaNO3 instead of CsNO3, no appreciable extraction was observed, as inferred from the lack of spectral shifts.† Collectively, these results provide support for the contention that copolymer 3 is capable of removing caesium nitrate from the aqueous layer, as inferred in part from the observation that little or no spectral response is observed upon extraction of caesium salts containing other anions (e.g., F−, Cl−, Br−, and SO4−), or in the case of other metal nitrate salts (e.g., sodium and potassium).
![Partial 1H NMR spectra (7.6–3.4 ppm) of 3 dissolved in CD2Cl2 ([calix[4]arene]0 = 6 mM) after exposure to (A) deionized water and various solutions of CsNO3 (B–E; concentrations noted in the respective spectra).](/image/article/2010/SC/c0sc00396d/c0sc00396d-f6.gif) |
| Fig. 6 Partial 1H NMR spectra (7.6–3.4 ppm) of 3 dissolved in CD2Cl2 ([calix[4]arene]0 = 6 mM) after exposure to (A) deionized water and various solutions of CsNO3 (B–E; concentrations noted in the respective spectra). | |
To test whether copolymer 3 could be used to extract caesium nitrate from aqueous environments in the presence of other anionic and cationic species (see Fig. 7 for schematic depiction), a CD2Cl2 solution of the copolymer 3 ([calix[4]arene]0 = 6 mM) was exposed to equal volume of an aqueous solution containing both NaNO3 and KNO3 (100 mM in each) in analogy to the experiments described above. In accord with what would be expected for a caesium cation-selective system, no evidence of salt extraction was observed by 1H NMR spectroscopy under these conditions.† However, mixing CD2Cl2 solutions of 3 ([calix[4]arene]0 = 6 mM) with an aqueous mixture containing CsNO3 (100 mM), NaNO3 (100 mM), and KNO3 (100 mM) resulted in spectral changes consistent with the coordination of CsNO3, as described above. Similarly, exposure of CD2Cl2 solutions of 3 ([calix[4]arene]0 = 6 mM) to an equal volume of an aqueous mixture of CsF, CsCl, and CsSO4 (the initial concentration of each salt was 100 mM) resulted in no significant spectral response. However, when 3 was exposed to an aqueous solution containing CsF, CsCl, CsSO4, CsBr, and CsNO3, a spectral response consistent with CsNO3 extraction was observed. Additional control experiments revealed that CsBr was also extracted by copolymer 3, albeit less effectively than CsNO3 (see ICP-MS results below). Collectively, these results are consistent with the Hofmeister effect,19,29 which implies that hydrophobic anions are extracted more readily than their hydrophilic counterparts (ΔhydG° (kJ mol−1): NO3− = −300; Br− = −315; Cl− = −340; F− = −465).30
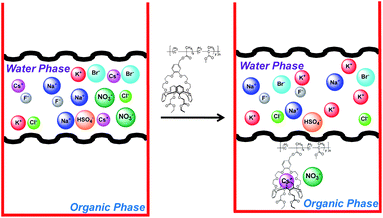 |
| Fig. 7 Schematic representation of the extraction properties of copolymer 3 highlighting the ability of this system to extract caesium nitrate from aqueous environments containing several other ionic species. | |
Another NMR spectral study was performed in an effort to establish further the selectivity of copolymer 3. In this experiment, a CD2Cl2 solution of 3 ([calix[4]arene]0 = 6 mM) was first exposed to an equal volume of an aqueous solution containing a complex salt mixture of a variety of inorganic salts (i.e., CsF, CsCl, CsSO4, KF, and NaCl; 100 mM in each). Under these conditions, no spectral response was observed (Fig. 8A) until CsNO3 (100 mM) was added (Fig. 8B). Moreover, the similar spectral response was observed when either KNO3 or NaNO3 (both 100 mM in D2O) were added to the initial salt mixture (Fig. 8C and 8D). To the best of our knowledge, this is the first direct demonstration of caesium extraction from a multi-component aqueous ionic mixture by a polymer-supported synthetic receptor system.
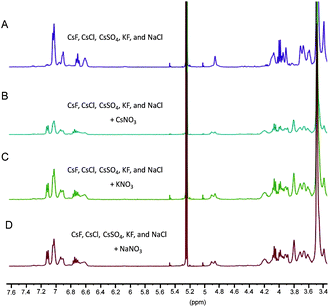 |
| Fig. 8 Partial 1H NMR spectra (7.6–3.4 ppm) of copolymer 3 in CD2Cl2 recorded after exposure to H2O solutions (all salts 100 mM) of A) CsF, CsCl, CsSO4, KF, and NaCl, B) CsF, CsCl, CsSO4, KF, NaCl + CsNO3, C) CsF, CsCl, CsSO4, KF, NaCl + KNO3 and D) CsF, CsCl, CsSO4, KF, NaCl + NaNO3. Mixtures were agitated for 30 min, separated via centrifugation, and the organic layer was isolated prior to analysis. | |
ICP-MS quantification studies
To support the colorimetric and 1H NMR spectroscopic analyses described above, inductively coupled mass spectrometry (ICP-MS) was used to quantify the caesium (as well as sodium and potassium) concentrations extracted in the aforementioned studies. These experiments were performed by exposing an aqueous solution of the analyte salt (e.g., CsBr, KNO3, or CsNO3; 200 mM) to an equal volume of a CH2Cl2 solution of copolymer 3 ([calix[4]arene]0 = 6 mM) in accord with the protocols described above. Upon phase separation, the organic layer was removed, evaporated, and then “digested” using “piranha” acid (30% v/v hydrogen peroxide in sulfuric acid). The resulting solution was then evaporated to dryness and the residual material was dissolved in a known volume of aqueous 2% nitric acid, filtered, and then analyzed by ICP-MS. After appropriate normalization, the number of receptor sites within the polymer containing caesium was calculated (reported as a percentage of total receptor sites bound; cf. Table 2).31 It was found that exposure of 3 to an aqueous solution of CsNO3 resulted in complexation of approximately 60% of the total receptor sites; for comparison, 10% of the total receptor sites were complexed upon exposure of 3 to an aqueous solution of CsBr. No detectable binding was observed for any of the other caesium salts that were subject to analysis. Analogous experiments performed with the nitrate salts of sodium and potassium revealed little evidence of either K+ or Na+ extraction (see ESI†). These later studies were consistent with the colorimetric and 1H NMR spectroscopic analyses described above; as such, they provide additional support for the suggestion that copolymer 3 is effective for caesium cation extraction.
Table 2 Summary of ICP-MS results
Extractant |
Caesium Salt |
133Csa (ppb) |
Receptor Sites Boundb (%) |
The concentration reported is after dilution with a known amount of aqueous 2% nitric acid.
Efficiencies are reported as the molar percentage of extractant populated with caesium after exposure to an aqueous solution of CsNO3. The error values given represent the deviations seen in replicate (at least duplicate) measurements.
|
3 (6 mM) |
CsF |
100 |
0 ± 1 |
3 (6 mM) |
CsCl |
90 |
0 ± 1 |
3 (6 mM) |
CsBr |
530 |
10 ± 1.4 |
3 (6 mM) |
CsSO4 |
5 |
0 ± 0.9 |
3 (6 mM) |
CsNO3 |
2,300 |
60 ± 1.1 |
Isolation of caesium nitrate-bound copolymer
Having established that copolymer 3 is an effective extractant for caesium nitrate, efforts shifted toward exploring the ability of this material to facilitate the isolation of this salt. A CH2Cl2 solution of 3 ([calix[4]arene]0 = 6 mM) was vigorously mixed with an equal volume of an aqueous solution of CsNO3 ([CsNO3]0 = 200 mM). Upon phase separation, the organic layer was then removed and added drop-wise to an excess of cold hexanes. Subsequent analysis of the resulting precipitate by 1H NMR spectroscopy revealed that the CsNO3 remained bound to 3 (approximately 60% of the total receptor sites on a molar basis were occupied), as determined by 1H NMR spectroscopy and ICP-MS analysis.† This result was corroborated by thermogravimetric analysis which revealed that the residual mass of 3 isolated post-exposure to aqueous CsNO3 was 10% higher than that in a pre-exposed sample (see Fig. 2B). This value correlates well with the increase in mass based on the ICP-MS data (calculated value = 5%).32 In contrast, an analogous extraction experiment performed using 1 resulted in the formation of a slightly cloudy solution upon its addition to cold hexanes from which no appreciable amount of precipitate could be isolated. As demonstrated previously in the course of the ICP-MS studies discussed above, the caesium cation can be recovered from the polymer by “digestion” under oxidizing acidic conditions, followed by filtering and drying.
Conclusions
In summary, a copolymer of MMA and a methacrylate derivative of benzocrown-6-calix[4]arene, copolymer 3, was synthesized. In the case of the picrate salts, greater selectivity for Cs+ over K+ and Na+ was observed for 3 as compared to an appropriate small molecule analogue (1). Copolymer 3 was also found to be effective in extracting caesium nitrate from aqueous media in the presence of various other inorganic ions. We conclude that appending a benzocrown-6-calix[4]arene to a PMMA backbone creates a system with significant advantages relative to the monomeric receptor from which it is derived. In particular, if desired, copolymer 3 may be used to recover caesium from aqueous environments via a straightforward extraction and reprecipitation procedure. This process, which cannot be easily duplicated using a low molecular weight analogue (i.e., 1) leads us to suggest that polymers, such as 3, may have a role to play in the generalized arena of ion extraction chemistry. More broadly, the present study serves to demonstrate that incorporation of appropriately chosen receptors into polymers can give rise to systems whose function is not impaired and, in favorable situations, can actually be enhanced relative to the constituent monomers.
Acknowledgements
This work was supported by the U.S. Department of Energy (grant DE-FG02-01ER-15186 to J.L.S.), the ONR (grant N00014-08-1-0729 to C.W.B.) and the Robert A. Welch Foundation (grants F-1018 and 1621 to J.L.S. and C.W.B., respectively). Support under the WCU (World Class University) program (R32-2008-000-10217-0) and the Creative Research Initiative program (grant No. 2010-0000728 to J.S.K.) is also acknowledged.
Notes and references
-
(a)
C. D. Gutsche, in Calixarenes Revisited, Monographs in Supramolecular Chemistry, ed. J. F. Stoddart, The Royal Society of Chemistry, Cambridge, 1998 Search PubMed;
(b)
G. D. Andreetti and F. Ugozzoli, in Calixarenes, A Versatile Class of Macrocyclic Compounds, ed. J. Vicens, V. Böhmer, Kluwer, Dordrecht, 1991 Search PubMed;
(c)
E. B. Brouwer, G. D. Enright, C. I. Ratcliffe, J. A. Ripmeester and K. A. Udanchin, in Calixarenes 2001, ed. Z. Asfari, V. Böhmer, J. Harrowfield, J. Vicens, Kluwer, Dordrecht, 2001 Search PubMed.
-
(a) S. Memon, M. Tabakci, D. M. Roundhill and M. Yilmaz, React. Funct. Polym., 2006, 66, 1342–1349 CrossRef CAS;
(b) S. D. Alexandratos and S. Natesan, Macromolecules, 2001, 34, 206–210 CrossRef CAS;
(c) Y. Kang and D. M. Rudkevich, Tetrahedron, 2004, 60, 11219–11225 CAS;
(d) G. Arena, A. Casnati, A. Contino, L. Mirone, D. Sciotto and R. Ungaro, Chem. Commun., 1996, 2277–2278 RSC.
-
(a) G. U. Akkuş and C. J. Cebeci, J. Inclusion Phenom. Macrocyclic Chem., 2008, 62, 303–309 CrossRef;
(b) A. I. Costa, L. F. Ferreira and J. V. Prata, J. Polym. Sci., Part A: Polym. Chem., 2008, 46, 6477–6488 CrossRef CAS;
(c) J. H. Wosnick and T. M. Swager, Chem. Commun., 2004, 2744–2745 RSC;
(d) J. E. Guillet and D. M. Gravett, Macromolecules, 1996, 29, 617–624 CrossRef CAS;
(e) M. T. Blanda and E. Adou, Polymer, 1998, 39, 3821–3826 CrossRef CAS.
-
(a) E. Akceylan, M. Bahadir and M. Yilmaz, J. Hazard. Mater., 2009, 162, 960–966 CrossRef CAS;
(b) Y. Yang and T. M. Swager, Macromolecules, 2007, 40, 7437–7440 CrossRef CAS;
(c) Y. Yang and T. M. Swager, Macromolecules, 2006, 39, 2013–2015 CrossRef CAS;
(d) M. T. Blanda and E. Adou, Chem. Commun., 1998, 139–140 RSC.
-
(a) L. O. Healy, M. M. McEnery, D. G. McCarthy, S. J. Harris and J. D. Glennon, Anal. Lett., 1998, 31, 1543–1551 CAS;
(b) S. Hutchinson, G. A. Kearney and S. J. Harris, Anal. Chim. Acta, 1994, 291, 269–275 CrossRef CAS.
-
(a) T. M. Swager, Acc. Chem. Res., 1998, 31, 201–207 CrossRef CAS;
(b) T. D. McQuade, A. E. Pullen and T. M. Swager, Chem. Rev., 2000, 100, 2537–2574 CrossRef CAS.
-
(a) D. W. M. Arrigan, G. Svehla, S. J. Harris and M. A. McKervey, Electroanalysis, 1994, 6, 97–106 CAS;
(b) K. M. O'Connor, M. Cherry, G. Svehla, S. J. Harris and M. A. McKervey, Talanta, 1994, 41, 1207–1217 CrossRef CAS.
-
(a) J. S. Kim and J. Vicens, J. Inclusion Phenom. Macrocyclic Chem., 2009, 63, 189–193 CrossRef CAS;
(b) K. Salorinne and M. Nissinen, J. Inclusion Phenom. Macrocyclic Chem., 2008, 61, 11–27 CrossRef CAS;
(c) S. K. Kim, J. K. Lee, S. H. Lee, M. S. Lim, S. W. Lee, W. Sim and J. S. Kim, J. Org. Chem., 2004, 69, 2877–2880 CAS;
(d) S. K. Kim, J. Vicens, K.-M. Park, S. S. Lee and J. S. Kim, Tetrahedron Lett., 2003, 44, 993–997 CrossRef CAS;
(e) S. K. Kim, W. Sim, J. Vicens and J. S. Kim, Tetrahedron Lett., 2003, 44, 805–809 CrossRef CAS;
(f) R. Ungaro, A. Casnati, F. Ugozzoli, A. Pochini, J.-F. Dozol, C. Hill and H. Rouquette, Angew. Chem., 1994, 106, 1551–1553 CAS.
-
(a) X. Liu, K. Surowiec and R. A. Bartsch, Tetrahedron, 2009, 65, 5893–5898 CrossRef CAS;
(b) M. T. Blanda, D. B. Farmer, J. S. Broadbelt and B. J. Goolsby, J. Am. Chem. Soc., 2000, 122, 1486–1491 CrossRef CAS;
(c) S. H. Lee, J. Y. Kim, J. Ko, J. Y. Lee and J. S. Kim, J. Org. Chem., 2004, 69, 2902–2905 CrossRef CAS;
(d) J. S. Kim, W. K. Lee, W. Sim, J. W. Ko, M. H. Cho, D. Y. Ra and J. W. Kim, J. Inclusion Phenom. Macrocyclic Chem., 2000, 37, 189–193.
- Compound 1 was synthesized in a manner analogous to 2; see ESI† for synthesis and characterization.
-
(a) A. Casnati, A. Pochini, R. Ungaro, F. Ugozzoli, F. Arnaud, S. Fanni, M.-J. Schwing, R. J. M. Egberink, F.d. Jong and D. N. Reinhoudt, J. Am. Chem. Soc., 1995, 117, 2767–2777 CrossRef CAS;
(b) R. A. Sachleben, J. C. Bryan, N. L. Engle, T. J. Haverlock, B. P. Hay, A. Urvoas and B. A. Moyer, Eur. J. Org. Chem., 2003, 4862–4869 CrossRef CAS;
(c) K. Kavallieratos and B. A. Moyer, Chem. Commun., 2001, 1620–1621 RSC;
(d) R. A. Sachleben, A. Urvoas, J. C. Bryan, T. J. Haverlock, B. P. Hay and B. A. Moyer, Chem. Commun., 1999, 1751–1752 RSC;
(e) R. A. Sahleben, P. V. Bonnesen, T. Descazeaud, T. J. Haverlock, A. Urvoas and B. A. Moyer, Solvent Extr. Ion Exch., 1999, 17, 1445–1459 CrossRef CAS.
-
(a) H. Yamamoto and S. Shinkai, Chem. Lett., 1994, 1115–1118 CAS;
(b) Z. Asfari, C. Bressot, J. Vicens, C. Hill, J.-F. Dozol, H. Rouquette, S. Eymard, V. Lamare and B. Tounois, Anal. Chem., 1995, 67, 3133–3119 CrossRef CAS;
(c) V. Lamare, J.-F. Dozol, F. Ugozzoli, A. Casnati and R. Ungaro, Eur. J. Org. Chem., 1998, 8, 1559–1568 CrossRef.
-
(a) T. J. Haverlock, P. V. Bonnesen, R. A. Sachleben and B. A. Moyer, Radiochim. Acta, 1997, 76, 103–108 CAS;
(b) H.-F. Ji, R. Dabestani, G. M. Brown and R. A. Sachleben, Chem. Commun., 2000, 833–834 RSC;
(c) C. Hill, J.-F. Dozol, V. Lamare, H. Rouquette, S. Eymard, B. Tournois, J. Vicens, Z. Asfari, C. Bressot, R. Ungaro and A. Casnati, J. Inclusion Phenom. Mol. Recognit. Chem., 1994, 19, 399–408 CrossRef CAS.
-
(a) T. J. Haverlock, P. V. Bonnesen, R. A. Schleben and B. A. Moyer, J. Inclusion Phenom. Macrocyclic
Chem., 2000, 36, 21–37 CrossRef CAS;
(b) M. G. Gorbunova, P. V. Bonnesen, N. L. Engle, E. Bazelaire, L. H. Delmau and B. A. Moyer, Tetrahedron Lett., 2003, 44, 5397–5401 CrossRef CAS.
-
(a)
J.-F. Dozol, Z. Asfari, C. Hill, J. Vicens, FR 2698362, 1994;
(b)
J.-F. Dozol, H. Rouquette, R. Ungaro, A. Casnati, WO 9424138, 1994;
(c)
B. A. Moyer, R. A. Sachelben, P. V. Bonnesen, D. J. Pesley, WO 9912878, 1999;
D. R. Peterman, D. H. Meikrantz, J. D. Law, C. L. Riddle, T. A. Todd, M. R. Geenhalgh, R. D. Tillotson, R. A. Bartsch, B. A. Moyer, L. H. Delmau, P. V. Bonnesen, US. Pat. Appl. 2010116749, 2010.
-
(a) G. U. Akkuş, S. Memon, D. E. Gürkaş, S. Aslan and M. Yilmaz, React. Funct. Polym., 2008, 68, 125–132 CrossRef;
(b) F. Yang, H. Guo, X. Cai and X. Chen, React. Funct. Polym., 2005, 64, 163–168 CrossRef CAS;
(c) H. Li and Y. Chen, React. Funct. Polym., 2003, 55, 171–178 CrossRef CAS;
(d) S. Memon, G. Uysal and M. Yilmaz, J. Macromol. Sci. Pure Appl. Chem., 2001, A38, 173–184 CrossRef CAS;
(e) S. Memon, G. Uysal and M. Yilmaz, React. Funct. Polym., 2001, 47, 165–174 CrossRef CAS;
(f) J. S. Kim, S. K. Kim, J. W. Ko, E. T. Kim, S. Yu, M. H. Cho, S. G. Kwon and E. H. Lee, Talanta, 2000, 52, 1143–1148 CrossRef CAS;
(g) Z.-L. Zhong, C.-P. Tang, C.-Y. Wu and Y.-Y. Chen, J. Chem. Soc., Chem. Commun., 1995, 1737–1738 RSC;
(h) H.-h. Yu, A. E. Pullen, M. G. Büschel and T. M. Swager, Angew. Chem., Int. Ed., 2004, 43, 3700–3703 CrossRef CAS.
- M. Ulewicz, U. Lesinska, M. Bochenska and W. Walkowaik, Sep. Purif. Technol., 2007, 54, 299–305 CrossRef CAS.
-
(a) A. Aydogan, D. J. Coady, V. M. Lynch, A. Akar, M. Marquez, C. W. Bielawski and J. L. Sessler, Chem. Commun., 2008, 1455–1457 RSC;
(b) A. Aydogan, D. J. Coady, S. K. Kim, A. Akar, C. W. Bielawski, M. Marquez and J. L. Sessler, Angew. Chem., Int. Ed., 2008, 47, 9648–9652 CrossRef CAS.
- R. Custelcean and B. A. Moyer, Eur. J. Inorg. Chem., 2007, 1321–1340 CrossRef CAS.
- D. C. Gutsche and L.-G. Lin, Tetrahedron, 1986, 42, 1633–1640 CrossRef CAS.
- E. M. Collins, M. A. McKervey, E. Madigan, M. B. Moran, M. Owens, G. Ferguson and S. J. Harris, J. Chem. Soc., Perkin Trans. 1, 1991, 3137–3142 RSC.
- J.-H. Bu, Q.-Y. Zheng, C.-F. Chen and Z.-T. Huang, Tetrahedron, 2005, 61, 897–902 CrossRef CAS.
- S. K. Kim, S. H. Lee, J. Y. Lee, J. Y. Lee, R. A. Bartsch and J. S. Kim, J. Am. Chem. Soc., 2004, 126, 16499–16506 CrossRef CAS.
-
G. Odian, in Principles of Polymerization, John Wiley and Sons Inc, Hoboken, New Jersey, 4th edn, 2004, ch. 3 Search PubMed.
-
(a) The slight yellow color observed in the organic layer of Vial C (i.e., copolymer 4) is attributed to a modest anion extraction effect induced by the calix[4]pyrrole moiety attached to this particular copolymer;
(b) S. K. Kim, J. L. Sessler, D. L. Gross, C.-H. Lee, J. S. Kim, V. M. Lynch, L. H. Delmau and B. P. Hay, J. Am. Chem. Soc., 2010, 132, 5827–5836 CrossRef CAS;
(c) R. Custelcean, B. A. Moyer, J. L. Sessler, W.-S. Cho, D. Gross, G. W. Bates, S. J. Brooks, M. E. Light and P. A. Gale, Angew. Chem., Int. Ed., 2005, 44, 2537–2542 CrossRef CAS.
- In the case of both analogue 1 and copolymer 3, enhanced removal of caesium picrate was observed in the competition studies (Fig. 5) as compared to the direct extraction studies reported in Table 1. This is thought to reflect an increase in overall salt concentration in the aqueous layer, resulting in a “salting out” effect.
- Proton NMR spectroscopic methods were chosen rather than UV-vis analyses due to the lack of a useful chromophore in this set of caesium salts.
- J. L. Sessler, S. K. Kim, D. E. Gross, C.-H. Lee, J. S. Kim and V. M. Lynch, J. Am. Chem. Soc., 2008, 130, 13162–13166 CrossRef CAS.
- F. Hofmeister, Naunyn-Schmiedebergs Arch. Pharmacol., 1888, 24, 247–269.
-
(a) Y. J. Marcus, J. Chem. Soc., Faraday Trans., 1991, 87, 2995–2999 RSC;
(b)
B. A. Moyer, in Molecular Recognition: Receptors for Cationic Guests, ed. G. W. Gokel, Comprehensive Supramolecular Chemistry, ed. J. L. Atwood, J. E. D. Davies, D. D. MacNicol, F. Vögtle, and J.-M. Lehn, Pergamon, Oxford, 1996, vol. 1, pp. 377–416 Search PubMed;
(c)
Y. Marcus, in Ion Properties, Marcel Dekker, New York, 1997 Search PubMed.
- The percentage of receptor sites containing caesium was determined by normalizing the amount of caesium present in the analysis sample to the total number of possible receptor sites present in the copolymer used for extraction.
- The mass loss observed at 100 °C was attributed to water evaporation. This result was observed in spite of repeated attempts at drying the post-aqueous CsNO3 exposed copolymer and is thought to reflect hydration of the bound caesium salt.
Footnote |
† Electronic supplementary information (ESI) available: Additional synthetic details and spectroscopic (NMR, UV-vis, ICP-MS) data. See DOI: 10.1039/c0sc00396d |
|
This journal is © The Royal Society of Chemistry 2010 |