DOI:
10.1039/C0SC00279H
(Edge Article)
Chem. Sci., 2010,
1, 383-386
Ligand-assisted nickel-catalysed sp3–sp3 homocoupling of unactivated alkyl bromides and its application to the active template synthesis of rotaxanes†
Received
25th April 2010
, Accepted 28th May 2010
First published on
18th June 2010
Abstract
An efficient, mild and operationally simple Ni-catalysed sp3-carbon-to-sp3-carbon homocoupling of unactivated alkyl bromides has been developed and utilised in the active metal template synthesis of an alkyl chain axle [2]rotaxane. The key to the transformation is the use of tridentate nitrogen-donor-atom (terpy or pybox derived) ligands which inhibit competing β-hydride elimination of alkyl-Ni intermediates.
Introduction
Several of the earliest rotaxanes to be rationally synthesised consisted of rings threaded onto simple alkyl chain axles terminated by bulky ‘stoppering’ groups.1 The subsequent development2 of template approaches dramatically increased the efficiency of rotaxane forming reactions,3 however usually at the cost that permanent recognition elements to direct the interlocking need to be built into both components. Active template methods,4,5 in which transition metal ions act as both the template for the threaded architecture and as the catalyst for the covalent bond forming reaction that captures the interlocked structure, remove the requirement for a recognition motif in the thread. Nevertheless, the active template synthesis of alkyl chain rotaxanes has not previously been demonstrated, in part because sp3–sp3 C–C bond forming reactions are particularly challenging to achieve. Here we describe an active template rotaxane-forming reaction that achieves this difficult construction using a Ni-mediated homocoupling of bromoalkanes. The search for a successful active template system for alkyl chain rotaxane synthesis led to the discovery of this potentially useful catalytic transformation—a novel, mild dehalogenative homocoupling of unactivated alkyl bromides.
Transition metal catalysed sp3-carbon to sp3-carbon bond forming reactions
The paucity of effective, catalytic, transition-metal-mediated sp3–sp3 C–C bond forming reactions is largely due to the slow rates of oxidative addition of metal centres to sp3-carbon–halogen (and other heteroatom) bonds and the propensity of the resulting organometallic intermediates to undergo β-hydride elimination.6,7 Successful sp3-carbon-to-sp3-carbon coupling protocols are generally limited to halides that are activated towards oxidative addition and lack β-hydrogen atoms (for example, allylic and benzylic chlorides).8 Recently, the coupling of unactivated alkyl halides with alkyl organometallics under mild conditions using highly reactive Pd and Ni catalysts has been reported.9–12 The Ni-mediated reactions disclosed11,12 by Fu and co-workers are particularly noteworthy as they allow racemic secondary bromides to be cross-coupled with alkyl nucleophiles with excellent enantioselectively.12
Results and discussion
Active template synthesis of an alkyl chain axle rotaxane
We chose the Negishi system developed by Fu and co-workers as a starting point for our investigations into an active template alkyl-chain-forming reaction as it employs a pyridine-2,6-bisoxazoline (pybox) ligand12 that molecular modeling indicated13 could create a rigid endotopic coordination site when incorporated into an appropriate macrocyclic scaffold. Macrocycle 1 was synthesised in 10 steps from commercially available materials (see ESI†).
We initially focused on the reaction of a bulky alkyl bromide with its corresponding zincate—effectively a homocoupling as the zincate is derived from the bromide—reasoning that the formation of the zincate and the subsequent Ni-mediated coupling reaction might be carried out simultaneously in one pot. Pleasingly, stirring macrocycle 1, 1 equiv. of NiCl2·DME (dimethoxyethane) and 2.2 equiv. of bromide 2 in the presence of 4 equiv. of activated Zn in THF–N-methyl-2-pyrrolidone (NMP) at 80 °C over 18 h led to the formation of [2]rotaxane 3 (Scheme 1), as evidenced by analysis of the crude reaction mixture by 1H NMR spectroscopy and mass spectrometry. Although 1H NMR spectroscopy indicated complete consumption of macrocycle 1, isolation of [2]rotaxane 3 proved difficult as the pybox moiety of the macrocycle was unstable to chromatography on silica gel and also, to some extent, to the conditions of the reaction itself. Purification using reverse phase silica gel gave a modest isolated rotaxane yield of 24%.
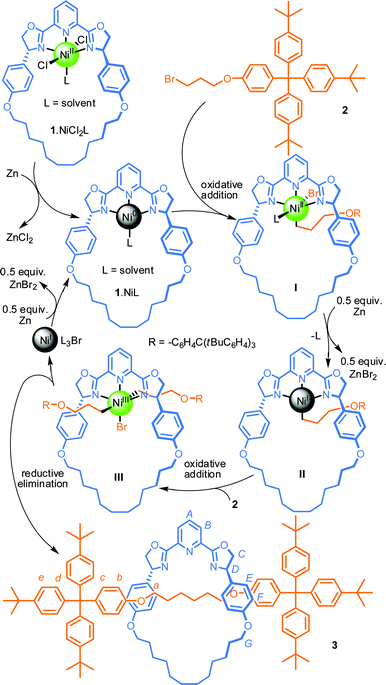 |
| Scheme 1 Rotaxane synthesis via a novel Ni-mediated active template sp3-carbon–sp3-carbon coupling reaction. Reagents and conditions: (i) 2 (2.2 equiv.), NiCl2·DME (1 equiv.), Zn (4 equiv.), NMP–THF (1 : 1), 80 or 25 °C, 18 h, 24% (80 °C) or 46% (25 °C). | |
Somewhat surprisingly, when the reaction protocol was modified to employ the preformed zincate of 2,14 no product was observed. This strongly implied that the homocoupling reaction was not in fact proceeding via a Negishi manifold.7,12 Replacing activated Zn with Mn powder in the original procedure gave a similar yield of rotaxane 3, indicating that the homocoupling reaction does not involve transmetallation of an alkyl organometallic species to Ni, as the formation of RMnX from Mn(0) should not occur to a significant extent under these conditions.15,16 Lowering the reaction temperature to 25 °C increased the yield of [2]rotaxane 3 to 46%, further evidence that formation of RZnX is not required for the homocoupling reaction.17
The results of these experiments are consistent with the mechanistic pathway shown in Scheme 1, in which Ni(II)-alkyl species I—produced by oxidative addition of the alkyl bromide to 1·Ni(0)L—is reduced to the Ni(I)-intermediate II by Zn, which then oxidatively adds to another equivalent of the alkyl bromide. The isolation of the interlocked product indicates that this oxidative addition must proceed through the cavity of the macrocycle to give threaded complex III which then reductively eliminates in a concerted fashion to give [2]rotaxane 3. The resulting Ni(I) complex can then be reduced to Ni(0) by Zn and restart the catalytic cycle.18 This mechanism is similar to that accepted for the Ni-mediated coupling of aryl halides19 and that proposed for the dehalogenative cross-coupling of aryl and alkyl halides.15
The interlocked structure of [2]rotaxane 3 was confirmed by comparison of its 1H NMR spectrum with the spectra of the corresponding non-interlocked macrocycle (1) and thread (Fig. 1). The shielding effects typical of face-on interactions of aromatic rings in interlocked architectures are observed for several of the resonances present in 3. Furthermore the signal corresponding to Ha is more complex in the rotaxane than the thread due to the chiral environment of the macrocycle which renders these protons diastereotopic.
General Ni-catalysed sp3-carbon–sp3-carbon homocoupling of unactivated alkyl bromides
Although metal-promoted sp2-carbon–sp2-carbon homocouplings are well known,20 the metal-catalysed sp3-carbon–sp3-carbon homocoupling of unactivated alkyl halides has not previously been described.18,21 The classic Wurtz coupling22 can bring about this type of transformation, but it is seldom used in a practical context as it requires stoichiometric quantities of highly reactive metals (e.g. Na) and normally gives low yields due to competing elimination and rearrangement processes. The harsh conditions for Wurtz couplings are only compatible with a few functional group types, a problem shared by sp3-carbon–sp3-carbon bond forming reactions between Grignard reagents and alkyl halides or sulfonates. One of the most popular ways to couple molecular fragments via an sp3-carbon–sp3-carbon bond is through ring closing (intramolecular) or cross (intermolecular) olefin metathesis followed by hydrogenation of the resulting internal alkene.23 Accordingly, a mild general method for the dehalogenative homocoupling of unactivated alkyl bromides to form C–C bonds potentially has wide practical utility and so we performed an optimisation study (Scheme 2, Table 1) on the catalytic protocol discovered above and explored the generality of the transformation (Table 2).
Entry |
Ni source (mol%) |
Ligand |
Yield/% |
Time/h |
Reagents and conditions: (i) 5 (1 equiv.), Zn (1 equiv.), NMP–THF (1 : 1), rt.
DMF.
Yield assessed by GCMS analysis.
Isolated yield.
|
1a |
NiCl2·DME (50) |
4
|
>95c |
18 |
2a |
NiCl2·DME (12.5) |
4
|
>95c |
18 |
3a |
NiCl2·DME (5) |
4
|
93d |
18 |
4a |
NiCl2·DME (2.5) |
4
|
50c |
18 |
5a |
NiCl2·DME (5) |
7
|
86d |
18 |
6a |
NiCl2·(H2O)6 (5) |
7
|
88d |
18 |
7b |
NiCl2·(H2O)6 (5) |
7
|
95d |
18 |
8b |
NiCl2·(H2O)6 (5) |
7
|
95d |
1 |
9b |
NiCl2·(H2O)6 (5) |
8
|
<5c |
18 |
10b |
NiCl2·(H2O)6 (5) |
9
|
<5c |
18 |
11b |
NiCl2·(H2O)6 (5) |
— |
0 |
18 |
Table 2 Substrate scope for the 2,2′:6′,2′′-terpyridine (7)-assisted Ni-catalysed homocoupling of alkyl bromidesa
Entry |
Substrate |
Product |
Yield/% |
NiCl2·(H2O)6, 2,2′:6′,2′′-terpyridine 7, DMF, 4 h, rt.
18 h.
1 : 1 mixture of diastereoisomers. p-Tol = 4-CH3-C6H4–.
|
1 |
|
|
97 |
2 |
|
|
96 |
3b |
|
|
78 |
4 |
|
|
>99 |
5 |
|
|
>99 |
6 |
|
|
95 |
7 |
|
|
>99 |
8 |
|
|
80c |
When 1-bromo-3-phenoxypropane 5 was treated with Ni(II) and acyclic pybox ligand 4 (replacing macrocycle 1), under the conditions developed for the active template reaction, 6 was isolated in essentially quantitative yield (>95%, Table 1, entry 1). This indicates that the homocoupling reaction itself is extremely efficient and supports the notion that the yield of rotaxane 3 is limited by the stability of the macrocycle under the reaction conditions. It proved possible to reduce the loading of Ni(II) to 5 mol% (entry 3) without any significant reduction in product yield, but lower catalyst loadings were less effective (entry 4). Replacing chiral ligand 4 with achiral tridentate ligand 2,2′:6′,2′′-terpyridine (7) led to a slight reduction in yield (entry 5) which was improved when cheap, air stable, NiCl2·(H2O)6 was substituted for air sensitive NiCl2·DME (entry 6). Changing the solvent to DMF improved both the reaction yield with the NiCl2·(H2O)6 catalyst (entry 7) and the reaction rate (entry 8). The crucial role played by the tridentate ligand10 (4 or 7) was demonstrated by control reactions with bidentate (8) and monodentate (9) analogues (entries 9 and 10) or the absence of any ligand (entry 11), each of which resulted in virtually no product being formed.
The substrate scope of the reaction was also investigated (Table 2). The reaction tolerates standard oxygen and nitrogen function protecting groups well (entries 1 and 2), including modestly acidic NH groups (entry 1) and esters (entry 3; longer reaction times were required here, possibly due to chelation of the Ni by the ester carbonyl). Alkenyl substrates are also compatible with the reaction (entry 4). The absence of cyclopentane by-products when using this substrate suggests that radical pathways are not in operation10 during the oxidative addition and reductive elimination steps, a finding reinforced by the formation of the threaded product during the active template reaction (Scheme 1). Clear selectivity between C–Br and C–Cl bonds is demonstrated by the chemoselective reaction of 1,3-chlorobromopropane (entry 5). Homocoupling of benzyl bromide proceeds in high yield (entry 6) and coupling of bromocyclohexane is essentially quantitative (entry 7), showing that secondary alkyl bromides are also highly effective substrates for this reaction. Although the dimerisation of a racemic secondary bromide proceeded in good yield (entry 8), no selectivity between the chiral and meso diastereoisomers was observed (d.r. = 1
:
1). Repeating this reaction using (R,R)-Ph-pybox 4 gave no change in the diastereoisomeric ratio, suggesting that the stereoconvergent process demonstrated12 by Fu and co-workers in their Negishi cross-couplings of racemic alkyl halides does not appear to operate in this system.
Conclusions
Through the search for an sp3-carbon–sp3-carbon bond forming active template reaction to produce an alkyl chain axle rotaxane, we have developed a high yielding general method for the homocoupling of primary and secondary alkyl bromides. This ligand-assisted nickel-catalysed procedure is operationally simple, efficient and cheap and may prove to be broadly applicable. Active template rotaxane synthesis not only represents an advanced strategy for the construction of mechanically interlocked molecules but, as exemplified here, can also serve as an effective conduit for reaction discovery.5
Acknowledgements
We thank Drs Hon Lam and Mike Greaney (Edinburgh) for useful discussions, Syngenta for a PhD studentship (to PRM) and the EPSRC National Mass Spectrometry Service Centre (Swansea, UK) for mass spectrometry. DAL is an EPSRC Senior Research Fellow and holds a Royal Society-Wolfson research merit award.
Notes and references
- I. T. Harrison and S. Harrison, J. Am. Chem. Soc., 1967, 89, 5723–5724 CrossRef CAS; G. Schill and H. Zöllenkorpf, Justus Liebigs Ann. Chem., 1969, 721, 53–74 CAS; H. Ogino, J. Am. Chem. Soc., 1981, 103, 1303–1304 CrossRef CAS.
- D. B. Amabilino and J. F. Stoddart, Chem. Rev., 1995, 95, 2725–2828 CrossRef CAS;
Molecular Catenanes, Rotaxanes and Knots: A Journey Through the World of Molecular Topology, ed. J.-P. Sauvage and C. Dietrich-Buchecker, Wiley-VCH, Weinheim, Germany, 1999 Search PubMed; G. A. Breault, C. A. Hunter and P. C. Mayers, Tetrahedron, 1999, 55, 5265–5293 Search PubMed; L. Raehm, D. G. Hamilton and J. K. M. Sanders, Synlett, 2002, 1743–1761 CrossRef; K. Kim, Chem. Soc. Rev., 2002, 31, 96–107 CAS; E. R. Kay and D. A. Leigh, Top. Curr. Chem., 2005, 262, 133–177 RSC; H. Tian and Q. C. Wang, Chem. Soc. Rev., 2006, 35, 361–374 CAS; A. Bogdan, Y. Rudzevich, M. O. Vysotsky and V. Böhmer, Chem. Commun., 2006, 2941–2952 RSC; J. R. Nitschke, Acc. Chem. Res., 2007, 40, 103–112 RSC; S. J. Loeb, Chem. Soc. Rev., 2007, 36, 226–235 CrossRef CAS; J. A. Faiz, V. Heitz and J.-P. Sauvage, Chem. Soc. Rev., 2009, 38, 422–442 RSC; K. M. Mullen and P. D. Beer, Chem. Soc. Rev., 2009, 38, 1701–1713 RSC; J. J. Gassensmith, J. M. Baumes and B. D. Smith, Chem. Commun., 2009, 6329–6338 RSC.
- F. G. Gatti, D. A. Leigh, S. A. Nepogodiev, A. M. Z. Slawin, S. J. Teat and J. K. Y. Wong, J. Am. Chem. Soc., 2001, 123, 5983–5989 CrossRef CAS; J. S. Hannam, T. J. Kidd, D. A. Leigh and A. J. Wilson, Org. Lett., 2003, 5, 1907–1910 CrossRef CAS.
- V. Aucagne, K. D. Hänni, D. A. Leigh, P. J. Lusby and D. B. Walker, J. Am. Chem. Soc., 2006, 128, 2186–2187 CrossRef CAS; S. Saito, E. Takahashi and K. Nakazono, Org. Lett., 2006, 8, 5133–5136 CrossRef CAS; J. D. Crowley, K. D. Hänni, A.-L. Lee and D. A. Leigh, J. Am. Chem. Soc., 2007, 129, 12092–12093 CrossRef CAS; V. Aucagne, J. Berná, J. D. Crowley, S. M. Goldup, K. D. Hänni, D. A. Leigh, P. J. Lusby, V. E. Ronaldson, A. M. Z. Slawin, A. Viterisi and D. B. Walker, J. Am. Chem. Soc., 2007, 129, 11950–11963 CrossRef CAS; J. Berná, J. D. Crowley, S. M. Goldup, K. D. Hänni, A.-L. Lee and D. A. Leigh, Angew. Chem., Int. Ed., 2007, 46, 5709–5713 CrossRef CAS; S. M. Goldup, D. A. Leigh, P. J. Lusby, R. T. McBurney and A. M. Z. Slawin, Angew. Chem., Int. Ed., 2008, 47, 3381–3384 CrossRef CAS; J. Berná, S. M. Goldup, A.-L. Lee, D. A. Leigh, M. D. Symes, G. Teobaldi and F. Zerbetto, Angew. Chem., Int. Ed., 2008, 47, 4392–4396 CrossRef CAS; Y. Sato, R. Yamasaki and S. Saito, Angew. Chem., Int. Ed., 2009, 48, 504–507 CrossRef CAS; J. D. Crowley, S. M. Goldup, A.-L. Lee, D. A. Leigh and R. T. McBurney, Chem. Soc. Rev., 2009, 38, 1530–1541 RSC; S. M. Goldup, D. A. Leigh, T. Long, P. R. McGonigal, M. D. Symes and J. Wu, J. Am. Chem. Soc., 2009, 131, 15924–15929 CrossRef CAS; S. M. Goldup, D. A. Leigh, P. R. McGonigal, V. E. Ronaldson and A. M. Z. Slawin, J. Am. Chem. Soc., 2010, 132, 315–320 CrossRef CAS; J. D. Crowley, K. D. Hänni, D. A. Leigh and A. M. Z. Slawin, J. Am. Chem. Soc., 2010, 132, 5309–5314 CrossRef CAS.
- J. D. Crowley, S. M. Goldup, N. D. Gowans, D. A. Leigh, V. E. Ronaldson and A. M. Z. Slawin, J. Am. Chem. Soc., 2010, 132, 6243–6248 CrossRef CAS.
- D. J. Cárdenas, Angew. Chem., Int. Ed., 1999, 38, 3018–3020 CrossRef CAS; D. J. Cárdenas, Angew. Chem., Int. Ed., 2003, 42, 384–387 CrossRef CAS; F. Glorius, Angew. Chem., Int. Ed., 2008, 47, 8347–8349 CrossRef CAS.
- M. R. Netherton and G. C. Fu, Adv. Synth. Catal., 2004, 346, 1525–1532 CrossRef CAS.
-
Metal-Catalyzed Cross-Coupling Reactions, 2nd edn, ed. A. de Meijere and F. Diederich, Wiley-VCH, Weinheim, 2004 Search PubMed; J. P. Corbet and G. Mignani, Chem. Rev., 2006, 106, 2651–2710 Search PubMed.
- M. R. Netherton, C. Y. Dai, K. Neuschutz and G. C. Fu, J. Am. Chem. Soc., 2001, 123, 10099–10100 CrossRef CAS; J. H. Kirchhoff, C. Y. Dai and G. C. Fu, Angew. Chem., Int. Ed., 2002, 41, 1945–1947 CrossRef CAS; J. Terao, H. Watanabe, A. Ikumi, H. Kuniyasu and N. Kambe, J. Am. Chem. Soc., 2002, 124, 4222–4223 CrossRef CAS; J. Terao, A. Ikumi, H. Kuniyasu and N. Kambe, J. Am. Chem. Soc., 2003, 125, 5646–5647 CrossRef CAS; J. Terao, H. Todo, H. Watanabe, A. Ikumi and N. Kambe, Angew. Chem., Int. Ed., 2004, 43, 6180–6182 CrossRef CAS; T. J. Anderson, G. D. Jones and D. A. Vicic, J. Am. Chem. Soc., 2004, 126, 8100–8101 CrossRef CAS; N. Hadei, E. A. B. Kantchev, C. J. O'Brien and M. G. Organ, Org. Lett., 2005, 7, 3805–3807 CrossRef CAS; M. G. Organ, S. Avola, I. Dubovyk, N. Hadei, E. A. B. Kantchev, C. J. O'Brien and C. Valente, Chem.–Eur. J., 2006, 12, 4749–4755 CrossRef CAS; J. Terao, Y. Naitoh, H. Kuniyasu and N. Kambe, Chem. Commun., 2007, 825–827 RSC; J. Terao, H. Todo, S. A. Begum, H. Kuniyasu and N. Kambe, Angew. Chem., Int. Ed., 2007, 46, 2086–2089 CrossRef CAS; J. Terao and N. Kambe, Acc. Chem. Res., 2008, 41, 1545–1554 CrossRef CAS; G. Cahiez, C. Chaboche, C. Duplais, A. Giulliani and A. Moyeux, Adv. Synth. Catal., 2008, 350, 1484–1488 CrossRef CAS; O. Vechorkin and X. L. Hu, Angew. Chem., Int. Ed., 2009, 48, 2937–2940 CrossRef CAS; O. Vechorkin, Z. Csok, R. Scopelliti and X. L. Hu, Chem.–Eur. J., 2009, 15, 3889–3899 CrossRef CAS; T. Thaler, B. Haag, A. Gavryushin, K. Schober, E. Hartmann, R. M. Gschwind, H. Zipse, P. Mayer and P. Knochel, Nat. Chem., 2010, 2, 125–130 Search PubMed.
- G. D. Jones, J. L. Martin, C. McFarland, O. R. Allen, R. E. Hall, A. D. Haley, R. J. Brandon, T. Konovalova, P. J. Desrochers, P. Pulay and D. A. Vicic, J. Am. Chem. Soc., 2006, 128, 13175–13183 CrossRef CAS.
- J. R. Zhou and G. C. Fu, J. Am. Chem. Soc., 2003, 125, 12527–12530 CrossRef CAS; J. R. Zhou and G. C. Fu, J. Am. Chem. Soc., 2003, 125, 14726–14727 CrossRef CAS.
- C. Fischer and G. C. Fu, J. Am. Chem. Soc., 2005, 127, 4594–4595 CrossRef CAS; F. O. Arp and G. C. Fu, J. Am. Chem. Soc., 2005, 127, 10482–10483 CrossRef CAS; B. Saito and G. C. Fu, J. Am. Chem. Soc., 2007, 129, 9602–9603 CrossRef CAS; S. W. Smith and G. C. Fu, J. Am. Chem. Soc., 2008, 130, 12645–12647 CrossRef CAS.
- Molecular modeling was carried out using the SPARTAN package:
W. J. Hehre, SPARTAN '06, 1.1, Wavefunction, Inc., Irvine, CA 92612, 2006 Search PubMed.
- A 1 M solution of bromide 2 in NMP was heated at 80 °C for 4 h in the presence of Zn (1.5 equiv.) and iodine (5 mol%). The resulting mixture was added to a solution of macrocycle 1 (1 equiv.), NiCl2·DME (1 equiv.) and bromide 2 (1 equiv.) in THF and stirred for 18 h at 80 °C. No rotaxane formation was observed.
- D. A. Everson, R. Shrestha and D. J. Weix, J. Am. Chem. Soc., 2010, 132, 920–921 CrossRef CAS.
- M. Durandetti, C. Gosmini and J. Périchon, Tetrahedron, 2007, 63, 1146–1153 CrossRef CAS; C. Gosmini, C. Bassene-Ernst and M. Durandetti, Tetrahedron, 2009, 65, 6141–6146 CrossRef CAS; M. Durandetti, L. Hardou, M. Clément and J. Maddaluno, Chem. Commun., 2009, 4753–4755 RSC.
- Elevated temperatures are usually required for the efficient formation of alkyl zinc species from unactivated alkyl bromides in aprotic polar solvents: S. Q. Huo, Org. Lett., 2003, 5, 423–425 Search PubMed.
- Vicic and co-workers have observed the homocoupling of alkyl iodides in the presence of stoichiometric Ni(COD)2 and 4,4′,4′′-tri-tert-butylterpyridine [ G. D. Jones, C. McFarland, T. J. Anderson and D. A. Vicic, Chem. Commun., 2005, 4211–4213 Search PubMed ]. This reaction may proceed through a mechanism similar to that shown in Scheme 1, with surplus Ni(0) acting as the reducing agent.
- T. T. Tsou and J. K. Kochi, J. Am. Chem. Soc., 1979, 101, 6319–6332 CrossRef CAS; T. T. Tsou and J. K. Kochi, J. Am. Chem. Soc., 1979, 101, 7547–7560 CrossRef CAS.
- M. Iyoda, H. Otsuka, K. Sato, N. Nisato and M. Oda, Bull. Chem. Soc. Jpn., 1990, 63, 80–87 CAS; W. M. Seganish, M. E. Mowery, S. Riggleman and P. DeShong, Tetrahedron, 2005, 61, 2117–2121 CrossRef CAS; Y. Yuan and Y. B. Bian, Appl. Organomet. Chem., 2008, 22, 15–18 CrossRef CAS.
- Homocouplings of alkyl halides using stoichiometric quantities of metal or electrochemical reduction have previously been reported: B. C. Ranu, P. Dutta and A. Sarkar, Tetrahedron Lett., 1998, 39, 9557–9558 Search PubMed; T. Nishino, T. Watanabe, M. Okada, Y. Nishiyama and N. Sonoda, J. Org. Chem., 2002, 67, 966–969 CrossRef CAS; P. Poizot, V. Jouikov and J. Simonet, Tetrahedron Lett., 2009, 50, 822–824 CrossRef CAS.
-
J. March, Advanced Organic Chemistry, 4th edn, Wiley-Interscience, New York, 1992, pp. 449–451 Search PubMed.
- R. H. Grubbs, Angew. Chem., Int. Ed., 2006, 45, 3760–3765 CrossRef CAS.
Footnote |
† Electronic supplementary information (ESI) available: Synthesis and characterisation of all compounds. CCDC reference number 774871. For ESI and crystallographic data in CIF or other electronic format see DOI: 10.1039/c0sc00279h |
|
This journal is © The Royal Society of Chemistry 2010 |