DOI:
10.1039/C0SC00272K
(Edge Article)
Chem. Sci., 2010,
1, 315-321
Temperature responsive channel uniformity impacts on highly guest-selective adsorption in a porous coordination polymer†
Received
20th April 2010
, Accepted 1st June 2010
First published on
25th June 2010
Abstract
Selective adsorption, so called “molecular sieving”, is one of the significant functions of porous materials because it can play an important role in separation processes to obtain highly pure gases or petroleum. One of the next outstanding challenges of porous materials is the achievement of controllable “molecular sieving” in response to external environments. Here, we show a new temperature responsive 1-dimensional porous compound (CPL-11) which changes its structural uniformity in response to ambient temperature, which gives rise to changes in the effective pore size. As a result of the structural responsiveness, the seeming adsorption properties of CPL-11 are apparently opposite to conventional porous compounds expected from thermodynamic law, resulting in a highly selective adsorption for O2 compared to Ar. This unexpected adsorption behavior indicates that the adsorption properties can be controlled by changing the channel uniformity of a porous framework depending on the ambient temperature.
Introduction
The recent advent of porous coordination polymers (PCPs) or metal–organic frameworks (MOFs) as new functional microporous adsorbents, has attracted the attention of chemists1–8 because of the great practicality of regular nano-sized spaces prepared by simply mixing their organic and inorganic molecular components which result in their finding superior functions such as in storage,9–24 catalysis25–28 and highly enantio-, size- and shape-selective adsorption.29–39 Furthermore, some PCPs possess highly soft frameworks that can transform from one to another in response to specific guest molecules.40–43 One of the next outstanding challenges of porous materials is the achievement of controllable “molecular sieving” in response to particular external environments, however, few examples of such systems are seen in conventional porous materials. This is because conventional porous materials such as zeolites or activated carbons have rigid frameworks, whose nature prevents structural responsiveness. Hence, there has been growing interest in new flexible and soft PCPs as the basis of a new class of practical porous materials, especially those that reversibly change their structures and porous properties in response to external stimuli.44
Adsorption properties of bulk porous compounds should be considered as the combination of (1) local crystalline characters originating from organic or inorganic building parts such as hydrophilicity, electrostatic nature, chirality, and pore size and shape and (2) global crystalline character originating from channel dimensionality and crystalline quality or uniformity, such as the amount of defects. Although most research has addressed only the relation between the adsorption properties and the local micropore character, less attention has been devoted to the global crystalline character for elucidating the adsorption properties of porous compounds (Fig. 1). Because the bulk crystalline solid and its adsorption properties have generally been regarded as an assembly of microscopic crystalline cells and each local pore’s character, respectively, it has been believed that a whole structural change would be essential to change the adsorption properties of a porous solid; in other words, the framework has to change from “one uniform structure A” to “another uniform structure B” to change the sorption properties. Therefore, to achieve the whole structural change, it is necessary to stimulate the whole crystal; otherwise the framework should have cooperative systems like dominos. However, we often meet problems in crystalline solids in that an external stimulus can only affect a few parts of the crystal and induce a partial structural transformation, making it a non-uniform structure, resulting in negligible changes of its bulk physical properties. With this view point, a new responsive porous system, in which even partial structural or non-uniform change can induce significant change of its porous properties, has been desired for promising controllable “molecular sieve” materials, and the global crystalline character has to be considered. In addition, channel dimensionality relates to guest diffusion; in particular a 1-dimensional channel is highly sensitive to local structural changes because each channel has only two entrances for guest molecules. In this work, we focused on a 1-dimensional nanochannel and the crystalline uniformity of a soft porous coordination polymer to realize controllable molecular sieving functions.
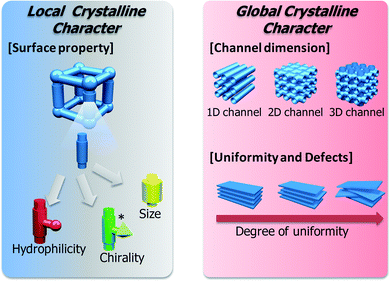 |
| Fig. 1 Local and global crystalline character of PCPs. | |
Experimental section
Syntheses of compounds
CPL-1 ([Cu2(pzdc)2pyz], (pzdc = pyrazine-2,3-dicarboxylate, pyz = pyrazine)) and CPL-2 ([Cu2(pzdc)2bpy] (bpy = 4,4′-bipyridine)) were synthesized using previously reported procedures.24,29,40
CPL-11 ([Cu2(pzdc)2bptz], (bptz = (3,6-bis(4-pyridyl)-1,2,4,5-tetrazine)): a water solution (50 mL) containing H2pzdc (84.1 mg, 0.50 mmol) was slowly added to a solution of Cu(ClO4)2·6H2O (135.3 mg, 0.37 mmol) and bptz (232.3 mg, 0.98 mmol) dissolved in H2O (500 mL) and EtOH (450 mL) at room temperature. After stirring for 1 day, the purple microcrystalline powder was filtered, washed with H2O and methanol, and dried under reduced pressure.
The adsorption isotherms shown in Fig. 3, 4A, 4B, 5A and 5B were volumetrically measured with BELSORP-18 and BELSORP-max volumetric adsorption equipment from Bel Japan, Inc. Measurement temperatures were precisely controlled by a liquid nitrogen bath, a constant temperature water bath or a closed helium cycle refrigerator based cryostat. All adsorbates of high purity (99.9999% for N2, O2, Ar, H2 and CO2, and 99.99% for Xe and CCl4, respectively) were used. All CPL host samples were obtained by treating under reduced pressure (<10−2 Pa) at 373 K for more than 10 h.
The powder samples for each compound were sealed in silica glass capillaries (0.4 mm inside diameter). XRPD patterns with good counting statistics were measured by synchrotron radiation using a large Debye–Scherrer camera with imaging plates as a detector on the BL02B2 beam line at the Super Photon Ring (SPring-8, Hyogo, Japan).45 All the XRPD patterns were obtained with a 0.01° step.
Structural determination by Rietveld analysis
Structure determinations were performed using high brilliance synchrotron powder diffraction data. The patterns were indexed by using the indexing program DICVOL91.46 Good quality unit cell refinements were obtained by using the structureless Le Bail fitting method.47 FOX software,48 which combines a rigid body and a Monte Carlo approach in real space, was used to find the initial positions of atoms for Rietveld analysis. The final structures were refined by the Rietveld technique.49,50 Soft constraints about bond angles and distances were adopted throughout the refinement. Hydrogen atoms were placed at calculated positions and their parameters were not refined. Crystallographic data and the values of Rwp and RI as reliability factors of Rietveld fitting for each compound are summarized in Table S1.†
Results and discussion
Design of soft 1-dimensional PCPs (CPLs)
Porous coordination pillared layer structures (CPLs) [Cu2(pzdc)2L] (pzdc = pyrazine-2,3-dicarboxylate, L = pillar ligand),24 which are constructed from both stiff and soft motifs, have guest responsive soft frameworks. In practice, the compound CPL-2 ([Cu2(pzdc)2bpy] (bpy = 4,4′-bipyridine)) shows a “guest shape responsive fitting” type of crystal-to-crystal transformation that gains high energetic benefit upon benzene accommodation.40 The structural dynamism in CPL-2 encouraged us to design a pore responsive to other external stimuli using CPL-type porous compounds. One of the options for controlling the network flexibility is tuning the shape and coordination ability of the ligand molecules: (1) a long ligand provides structural fragility because of a large open space, and (2) weak coordination promotes the enhancement of flexibility around the metal coordination moiety. In this respect, we designed a pillar ligand that meets the conditions. A long rod ligand, bptz (3,6-bis(4-pyridyl)-1,2,4,5-tetrazine), has pyridyl nitrogen donors at each end whose coordination ability is weaker than that of 4,4′-bipyridine because of a strong electron withdrawing effect of the central 1,2,4,5-tetrazine group. Advantageously, no bulky substituents are used for altering the basicity of the nitrogen donor. Then, we synthesized a new PCP compound, CPL-11 ([Cu2(pzdc)2bptz]), and also CPL-1 ([Cu2(pzdc)2pyz], (pyz = pyrazine)) and CPL-2 as control compounds.
The crystal structure of CPL-11 has a topologically similar framework to the previously reported CPL-1 and -2.29,40 In the CPL structures, two-dimensional layers of [Cu(pzdc)] form in the ac plane, which are connected by pillar ligands of pyz, bpy and bptz for CPL-1, -2, and -11, respectively, resulting in a 3D pillared layer structure. The CPL structures possess 1D channels along the a axis with cross sectional areas of 4 × 6, 7 × 6 and 10 × 6 Å2, for CPL-1, -2 and -11, respectively (Fig. 2).
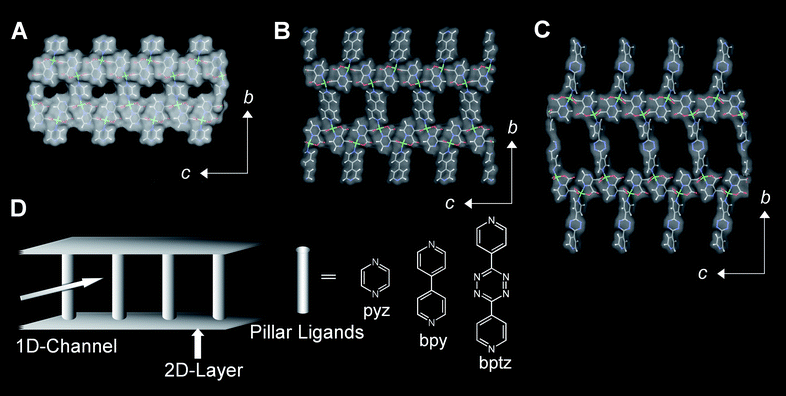 |
| Fig. 2 Representations of the crystal structures of CPLs (Cu, green; O, red; C, gray; N, blue; H, white). Orthographic views down the channel axis of CPL-1 (A), CPL-2 (B) and CPL-11 (C) displayed by stick and solvent accessible surface. Schematic representation of the CPL structure (D). | |
Adsorption properties of CPLs
First, to confirm the permanent porosity of CPL-11, the sorption isotherms of N2 at 77 K on CPL-11 were measured, and compared with those of CPL-1 and -2. The maximum amount of adsorption of CPL-2 is larger than that of CPL-1, which is consistent with the order of their pore volumes determined from their crystal structures. On the other hand, the isotherm of CPL-11 shows very little uptake of N2 compared with that expected from the large pore size (Fig. 3). When the size of the channel aperture is comparable with that of the adsorbate, the adsorbate cannot smoothly access the inner part of the channel and the amount adsorbed shows a much lower value than that expected from the pore volume, which is called the pore blocking effect.51–55 However, CPL-11 has a much larger aperture size than the dimension of a N2 molecule, and furthermore, the CPL-1 and -2, which are isostructural with CPL-11 with smaller channels, can adsorb N2. These results indicate that the nonporous behavior of CPL-11 is not simply caused by the pore blocking effect, so that further investigations are needed.
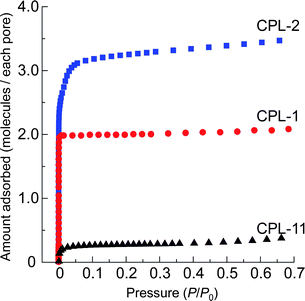 |
| Fig. 3 Adsorption isotherms for N2 at 77 K in the pressure range from 10−4 to 0.7 of P/P0. Adsorption isotherms on CPL-1, -2 and -11 are shown with red circles, blue squares and black triangles, respectively. | |
Fig. 4A and 4B show adsorption isotherms of CPL-11 for various adsorbates such as H2, CO2, Ar, O2, Xe and CCl4, whose kinetic diameters are 2.89, 3.3, 3.40, 3.46, 3.96 and 5.9 Å, respectively.56 Nonporous behavior for Ar, O2 and H2 at 77 K is observed as well as for N2 whereas CO2 and Xe at 195 K, and CCl4 at 298 K are readily adsorbed. It is surprising that H2 is hardly adsorbed in CPL-11 (3.3 mL (stp, standard temperature and pressure) g−1 at 85 kPa), whereas CCl4, whose size is more than twice as great as that of H2, can be adsorbed with no difficulty. In addition, this contrasts with the result that a large amount of H2 can be occluded in CPL-1 (56 mL(stp) g−1 at 85 kPa) (Fig. 4B). Consequently, CPL-11 shows completely nonporous behavior at 77 K for any guest molecules, and we could not understand these unusual adsorption phenomena of CPL-11 considering only the static metrics of the pore size and the guest molecules.
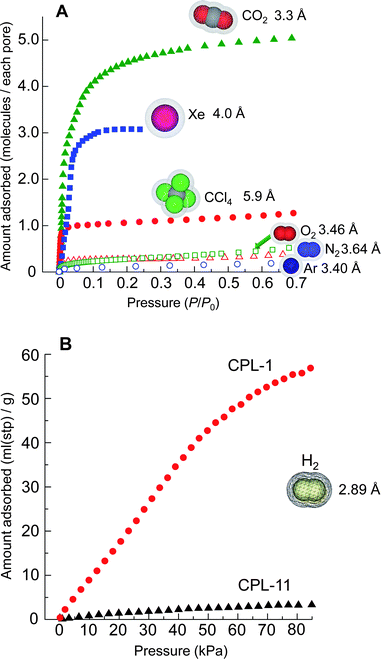 |
| Fig. 4 Adsorption isotherms. A, Adsorption isotherms on CPL-11 for CO2 (filled green triangles), Xe (blue filled squares), CCl4 (red filled circles), O2 (green open squares), N2 (red open triangles) and Ar (blue open circles) at temperatures of 195, 195, 298, 77, 77 and 77 K, respectively. The pressure range is from 10−4 to 0.7 of P/P0. B, Adsorption isotherms for H2 on CPL-1 (red circles) and CPL-11 (black triangles) at 77 K in the pressure range from 0 to 85 kPa. | |
The separation of O2 and Ar using an adsorbent is industrially important. However, usually it is difficult to find an apparent difference in adsorption of O2 and Ar using conventional adsorbents because these molecules are similar to one another in equilibrium sorption parameters, physicochemical properties, and molecular size and shape (Fig. S1†). We carried out adsorption measurements of Ar and O2 on CPL-11 at various temperatures (Fig. 5A and 5B). In general, increasing the temperature at constant relative pressure (P/P0) leads to a decreasing amount of adsorption according to the adsorption potential theory and Dubinin–Radushkevich equation.57 Surprisingly, there is a clear increase in the amount of O2 adsorbed with an increase in the measurement temperature, which is completely opposite to the tendency of the adsorption behaviors of usual porous compounds. On the other hand, little adsorption of Ar is found even at higher temperatures. These results point to the possibility that not only does CPL-11 show a highly selective O2 adsorption compared to Ar but also a temperature-responsive structural-transformation of the host framework from open to closed channels.
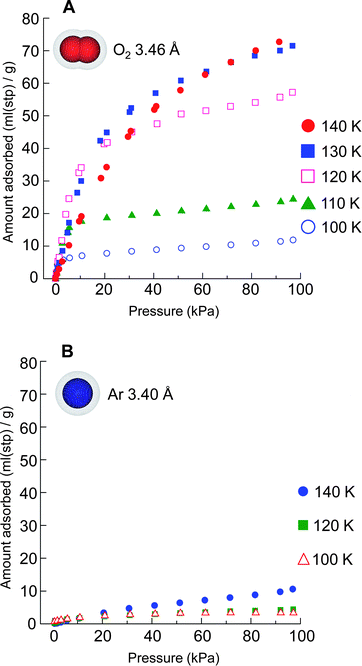 |
| Fig. 5 Adsorption isotherms. A, Adsorption isotherms for O2 on CPL-11 at temperatures of 140 (red filled circles), 130 (blue filled squares), 120 (pink open squares), 110 (green filled triangles) and 100 K (blue open circles). B, Adsorption isotherms for Ar on CPL-11 at the temperatures of 140 (blue filled circles), 120 (green filled squares) and 100 K (red open triangles). | |
Lattice strain of CPL-11 depending on temperature
Fig. 6A shows synchrotron in situ X-ray powder diffraction (XRPD) patterns of as-synthesized CPL-11 containing water molecules at 300 K and dehydrated CPL-11 with cooling from 300 to 77 K in a vacuum. During both the dehydration and cooling processes, no appreciable changes in the peak positions were observed, indicative of no drastic structural change. Despite the small changes in the peak positions during these processes, the peak shape undergoes considerable linewidth broadening. The XRPD line profile is attributable to the crystallite domain size and the lattice strain.58,59 In general, when the crystallite domain size is small or the lattice strain is large, the peak profile is broad; namely, the value of the full width at half maximum (FWHM) obtained from the line profile is large. If cracking of crystals occurs, resulting in a reduction in crystallite domain size through the adsorption/desorption or temperature increasing/decreasing processes, the line profile in the XRPD will irreversibly change during each process. In the case of CPL-11, however, we observed that the line profile of each peak changed reversibly, therefore, the change of line profile should only relate to the change of lattice strain.
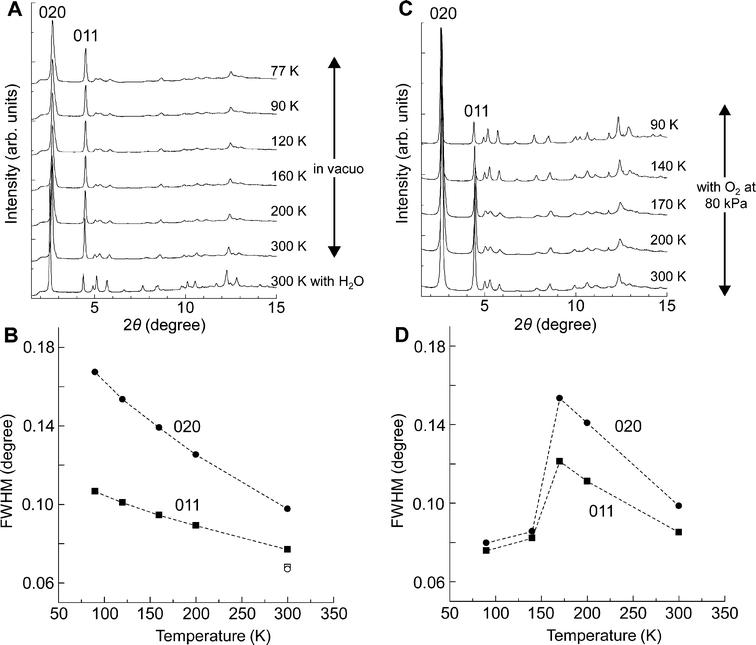 |
| Fig. 6
In situ synchrotron XRPD patterns of CPL-11 and the variation of the values of FWHM for the 020 and 011 peaks. The radiation wavelength is 0.80 Å. 2θ ranges from 3.5 to 15.0°. A, The XRPD patterns of as-synthesized CPL-11 and dried CPL-11 with cooling from 300 to 77 K. B, The variation of the values of FWHM for the 020 and 011 peaks shown in Fig. 6A. An open circle and an open square indicate the values of the 020 and 011 peaks of as-synthesized CPL-11 at 300 K, respectively. Solid circles and solid squares indicate the values of the 020 and 011 peaks of dried CPL-11, respectively. C, The XRPD patterns of dried CPL-11 with O2 at 80 kPa with cooling from 300 to 90 K. D, The variation of the values of FWHM for the 020 and 011 peaks shown in Fig. 6C. Solid circles and solid squares indicate the values of the 020 and 011 peaks, respectively. | |
The values of FWHM of the peaks 020 and 011 were estimated from each pattern (Fig. 6B): those from dehydrated CPL-11 at 300 K show larger values than those from hydrated CPL-11 at 300 K. In addition, the values increase continuously from 300 to 77 K, indicating that the lattice strain of CPL-11 increases through dehydration and decreasing temperature. On the other hand, other CPL compounds such as CPL-1 and -2 do not show any appreciable changes in line profile, indicating that this crystalline lattice softness is unique for CPL-11 (Fig. S2 and S3†). In the previous study, we found that CPL-2 showed a change of the coordination mode of the Cu ions between square pyramidal and square planar form upon guest adsorption/desorption. Although we need further investigation to obtain definite proof, the lattice strain could arise from some defects that may non-uniformly appear because of the labile coordination mode on copper ions so that the coordination ability of bptz ligands in CPL-11 is much weaker than bpy ligands in CPL-2.
The in situ XRPD patterns of dehydrated CPL-11 in an O2 atmosphere of 80 kPa show strong relations between the lattice strain and guest accommodation, shown in Fig. 6C. During the cooling process, drastic pattern changes and linewidth sharpening were observed between 170 and 140 K, which is due to O2 adsorption. The values of FWHM also suddenly decreased in this temperature range, indicating that O2 adsorption considerably reduces the lattice strain (Fig. 6D). The results can be explained as follows: the guest molecules filling the channel support the porous structure resulting in enhanced channel uniformity, which reduces lattice strain. On the other hand, under vacuum conditions, no support can be obtained and the lattice strain increases continuously upon cooling. Because the lattice strain should work to prevent the access of guest molecules into the pores, the decrease in the amount of O2 adsorbed with cooling, as shown in Fig. 5A, is consistent with the lattice strain tendency.
Conclusions
An unprecedented selective adsorption phenomenon has been achieved by a newly synthesized soft PCP, CPL-11. CPL-11 adsorbs large guest molecules at high temperature but does not adsorb any guest molecules at low temperature. In addition, cooling the host framework leads to a decrease in the amount adsorbed, which is obviously opposite to the tendency in usual porous materials. It has been shown that the unexpected adsorption behavior is strongly associated with the degree of structural uniformity, which can be controlled by changing the temperature, so that the adsorption capability of CPL-11 can be controlled by the ambient temperature. Most research into PCPs has been carried out based on the local character of individual pores and on the idea that the pore structure is almost perfect and uniform throughout the crystals. However, soft PCP crystals are not always uniform over the meso-domain and can provide different pores whose characteristics are controlled by external stimuli on demand. Such a soft porous compound is much more useful for selective guest adsorption than rigid porous compounds. In this work, we have shown the importance of the global crystalline character of a 1-dimensional porous compound, especially the structural uniformity, and based on this idea, we have also shown highly selective O2 adsorption as compared to Ar. We believe that control of the structural uniformity of a porous compound will open up a new field in porous materials, such as “external-stimuli-responsive pores”, being the best candidates for a porous material that can recognize and separate target molecules.
Acknowledgements
The authors thank Professor Dr T. C. Kobayashi, Professor Dr M. Miyahara and Dr R. Kitaura for their helpful discussions about the adsorption behaviour of CPL-11. The synchrotron radiation experiments were performed at the BL02B2 in SPring-8 with the approval of the Japan Synchrotron Radiation Research Institute (JASRI).
Notes and references
-
F. Schüth, K. S. W. Sing, J. Weitkamp, Handbook of Porous Solids, vol. 2, Wiley-VCH, Weinheim, 2002 Search PubMed.
- O. M. Yaghi, M. O'Keeffe, N. W. Ockwig, H. K. Chae, M. Eddaoudi and J. Kim, Nature, 2003, 423, 705–714 CrossRef CAS.
- G. Férey, Chem. Soc. Rev., 2008, 37, 191–214 RSC.
- M. J. Zaworotko, Nature, 2008, 451, 410–411 CrossRef CAS.
- C. Volkringer, D. Popov, T. Loiseau, N. Guillou, G. Férey, M. Haouas, F. Taulelle, C. Mellot-Draznieks, M. Burghammer and C. Riekel, Nat. Mater., 2007, 6, 760–764 CrossRef CAS.
- S. J. Dalgarno, N. P. Power and J. L. Atwood, Coord. Chem. Rev., 2008, 252, 825–841 CrossRef CAS.
- M. P. Suh, Y. E. Cheon and E. Y. Lee, Coord. Chem. Rev., 2008, 252, 1007–1026 CrossRef CAS.
- S. Kitagawa and R. Matsuda, Coord. Chem. Rev., 2007, 251, 2490–2509 CrossRef CAS.
- H. K. Chae, D. Y. Siberio-Perez, J. Kim, Y. B. Go, M. Eddaoudi, A. J. Matzger, M. O'Keeffe and O. M. Yaghi, Nature, 2004, 427, 523–527 CrossRef CAS.
- X. Zhao, B. Xiao, A. J. Fletcher, K. M. Thomas, D. Bradshaw and M. J. Rosseinsky, Science, 2004, 306, 1012–1015 CrossRef CAS.
- B. D. Chandler, G. D. Enright, K. A. Udachin, S. Pawsey, J. A. Ripmeester, D. T. Cramb and G. K. H. Shimizu, Nat. Mater., 2008, 7, 229–235 CrossRef CAS.
- H. J. Choi, M. Dincă and J. R. Long, J. Am. Chem. Soc., 2008, 130, 7848–7850 CrossRef CAS.
- J. A. R. Navarro, E. Barea, A. Rodriguez-Dieguez, J. M. Salas, C. O. Ania, J. B. Parra, N. Masciocchi, S. Galli and A. Sironi, J. Am. Chem. Soc., 2008, 130, 3978–3984 CrossRef CAS.
- K. L. Mulfort and J. T. Hupp, J. Am. Chem. Soc., 2007, 129, 9604–9605 CrossRef CAS.
- D. Li and K. Kaneko, J. Phys. Chem. B, 2000, 104, 8940–8945 CrossRef CAS.
- C. D. Wood, B. Tan, A. Trewin, F. Su, M. J. Rosseinsky, D. Bradshaw, Y. Sun, L. Zhou and A. I. Cooper, Adv. Mater., 2008, 20, 1916–1921 CrossRef CAS.
- Y. K. Park, S. B. Choi, H. Kim, K. Kim, B.-H. Won, K. Choi, J.-S. Choi, W.-S. Ahn, N. Won, S. Kim, D. H. Jung, S.-H. Choi, G.-H. Kim, S.-S. Cha, Y. H. Jhon and J. K. Yang, Angew. Chem., Int. Ed., 2007, 46, 8230–8233 CrossRef CAS.
- W. Yang, X. Lin, J. Jia, J. Blake Alexander, C. Wilson, P. Hubberstey, R. Champness Neil and M. Schröder, Chem. Commun., 2008, 359–361 RSC.
- F. Nouar, J. F. Eubank, T. Bousquet, L. Wojtas, M. J. Zaworotko and M. Eddaoudi, J. Am. Chem. Soc., 2008, 130, 1833–1835 CrossRef CAS.
- J. L. Belof, A. C. Stern, M. Eddaoudi and B. Space, J. Am. Chem. Soc., 2007, 129, 15202–15210 CrossRef CAS.
- E. Y. Lee, S. Y. Jang and M. P. Suh, J. Am. Chem. Soc., 2005, 127, 6374–6381 CrossRef CAS.
- S. Takamizawa, E.-i. Nakata, H. Yokoyama, K. Mochizuki and W. Mori, Angew. Chem., Int. Ed., 2003, 42, 4331–4334 CrossRef CAS.
- R. Kitaura, S. Kitagawa, Y. Kubota, T. C. Kobayashi, K. Kindo, Y. Mita, A. Matsuo, M. Kobayashi, H.-C. Chang, T. C. Ozawa, M. Suzuki, M. Sakata and M. Takata, Science, 2002, 298, 2358–2361 CrossRef CAS.
- M. Kondo, T. Okubo, A. Asami, S.-I. Noro, T. Yoshitomi, S. Kitagawa, T. Ishii, H. Matsuzaka and K. Seki, Angew. Chem., Int. Ed., 1999, 38, 140–143 CrossRef CAS.
- S. Horike, M. Dincă, K. Tamaki and J. R. Long, J. Am. Chem. Soc., 2008, 130, 5854–5855 CrossRef CAS.
- M. Fujita, J. Y. Kwon, S. Washizu and K. Ogura, J. Am. Chem. Soc., 1994, 116, 1151–1152 CrossRef CAS.
- J. S. Seo, D. Whang, H. Lee, S. I. Jun, J. Oh, Y. J. Jeon and K. Kim, Nature, 2000, 404, 982–986 CrossRef CAS.
- C.-D. Wu and W. Lin, Angew. Chem., Int. Ed., 2007, 46, 1075–1078 CrossRef CAS.
- R. Matsuda, R. Kitaura, S. Kitagawa, Y. Kubota, R. V. Belosludov, T. C. Kobayashi, H. Sakamoto, T. Chiba, M. Takata, Y. Kawazoe and Y. Mita, Nature, 2005, 436, 238–241 CrossRef CAS.
- R. Banerjee, A. Phan, B. Wang, C. Knobler, H. Furukawa, M. O'Keeffe and O. M. Yaghi, Science, 2008, 319, 939–943 CrossRef CAS.
- B. Wang, A. P. Cote, H. Furukawa, M. O'Keeffe and O. M. Yaghi, Nature, 2008, 453, 207–211 CrossRef CAS.
- S. Ma, X.-S. Wang, D. Yuan and H.-C. Zhou, Angew. Chem., Int. Ed., 2008, 47, 4130–4133 CrossRef CAS.
- M. E. Kosal, J.-H. Chou, S. R. Wilson and K. S. Suslick, Nat. Mater., 2002, 1, 118–121 CrossRef CAS.
- L. Pan, D. H. Olson, L. R. Ciemnolonski, R. Heddy and J. Li, Angew. Chem., Int. Ed., 2006, 45, 616–619 CrossRef CAS.
- A. Kondo, H. Noguchi, S. Ohnishi, H. Kajiro, A. Tohdoh, Y. Hattori, W.-C. Xu, H. Tanaka, H. Kanoh and K. Kaneko, Nano Lett., 2006, 6, 2581–2584 CrossRef CAS.
- M. J. Horner, K. T. Holman and M. D. Ward, J. Am. Chem. Soc., 2007, 129, 14640–14660 CrossRef CAS.
- G. J. Halder, C. J. Kepert, B. Moubaraki, K. S. Murray and J. D. Cashion, Science, 2002, 298, 1762–1765 CrossRef CAS.
- C.-D. Wu and W. Lin, Angew. Chem., Int. Ed., 2005, 44, 1958–1961 CrossRef CAS.
- T. K. Maji, R. Matsuda and S. Kitagawa, Nat. Mater., 2007, 6, 142–148 CrossRef CAS.
- R. Matsuda, R. Kitaura, S. Kitagawa, Y. Kubota, T. C. Kobayashi, S. Horike and M. Takata, J. Am. Chem. Soc., 2004, 126, 14063–14070 CrossRef CAS.
- D. Bradshaw, J. E. Warren and M. J. Rosseinsky, Science, 2007, 315, 977–980 CrossRef CAS.
- F. Millange, C. Serre, N. Guillou, G. Férey and R. I. Walton, Angew. Chem., Int. Ed., 2008, 47, 4100–4105 CrossRef CAS.
- C. Serre, S. Bourrelly, A. Vimont, N. A. Ramsahye, G. Maurin, P. L. Llewellyn, M. Daturi, Y. Filinchuk, O. Leynaud, P. Barnes and G. Férey, Adv. Mater., 2007, 19, 2246–2251 CrossRef.
- S. M. Kuznicki, V. A. Bell, S. Nair, H. W. Hillhouse, R. M. Jacubinas, C. M. Braunbarth, B. H. Toby and M. Tsapatsis, Nature, 2001, 412, 720–724 CrossRef CAS.
- E. Nishibori, M. Takata, K. Kato, M. Sakata, Y. Kubota, S. Aoyagi, Y. Kuroiwa, M. Yamakata and N. Ikeda, J. Phys. Chem. Solids, 2001, 62, 2095–2098 CrossRef CAS.
- A. Boultif and D. Louer, J. Appl. Crystallogr., 1991, 24, 987–993 CrossRef CAS.
- B. LeBail, H. Duroy and J. L. Fourquet, Mater. Res. Bull., 1988, 23, 447–452 CrossRef CAS.
- V. Favre-Nicolin and R. Cerny, J. Appl. Crystallogr., 2002, 35, 734–743 CrossRef CAS.
- H. M. Rietveld, J. Appl. Crystallogr., 1969, 2, 65–71 CrossRef CAS.
- F. Izumi and T. Ikeda, Mater. Sci. Forum, 2000, 198, 321–324.
- C. Martin, N. Tosi-Pellenq, J. Patarin and J. P. Coulomb, Langmuir, 1998, 14, 1774–1778 CrossRef CAS.
- C. Lastoskie, K. E. Gubbins and N. Quirke, J. Phys. Chem., 1993, 97, 4786–4796 CrossRef CAS.
- L. Pan, K. M. Adams, H. E. Hernandez, X. Wang, C. Zheng, Y. Hattori and K. Kaneko, J. Am. Chem. Soc., 2003, 125, 3062–3067 CrossRef CAS.
- M. Dincă and J. R. Long, J. Am. Chem. Soc., 2005, 127, 9376–9377 CrossRef CAS.
- E. Barea, G. Tagliabue, W.-G. Wang, M. Pérez-Mendoza, L. Mendez-Liñan, F. J. López-Garzon, S. Galli, N. Masciocchi and J. A. R. Navarro, Chem.–Eur. J., 2010, 16, 931–937 CAS.
-
D. W. Breck, Zeolite Molecular Sieves, Wiley & Sons, New York, 1984 Search PubMed.
- M. M. Dubinin, Chem. Rev., 1960, 60, 235–241 CrossRef CAS.
- P. Thompson, D. E. Cox and J. B. Hastings, J. Appl. Crystallogr., 1987, 20, 79–83 CrossRef CAS.
- K. Nomura, H. Uruno, S. Ono, H. Shinozuka and S. Suda, J. Less Common Met., 1985, 107, 221–230 CrossRef CAS.
|
This journal is © The Royal Society of Chemistry 2010 |
Click here to see how this site uses Cookies. View our privacy policy here.