DOI:
10.1039/C0NR00287A
(Minireview)
Nanoscale, 2010,
2, 1643-1649
Electrochemically powered self-propelled electrophoretic nanosubmarines†
Received
29th April 2010
, Accepted 21st May 2010
First published on
2nd August 2010
Abstract
In the past few years, we have witnessed rapid developments in the realization of the old nanotechnology dream, autonomous nanosubmarines. These nanomachines are self-powered, taking energy from their environment by electrocatalytic conversion of chemicals present in the solution, self-propelled by flux of the electrons within the submarine and the hydronium ions on the surface of the nanosub, powering it in the direction opposite to that of the flux of the hydronium. These nanosubmarines are responsive to external fields, able to follow complex magnetic patterns, navigate themselves in complex microfluidic channels, follow chemical gradients, carry cargo, and communicate with each other. This minireview focuses on a discussion of the fundamentals of the electrophoretic mechanism underlying the propulsion of this sort of nanosub, as well as a demonstration of the proof-of-concept capabilities of nanosubmarines.
Martin Pumera | Martin Pumera received his PhD at Charles University, Czech Republic, in 2001. Shortly thereafter, he joined Joseph Wang's SensoChip Laboratory in the USA as a post-doctoral researcher where he worked on the development of electrochemical lab-on-a-chip devices for space and security applications in cooperation with the Jet Propulsion Lab/Caltech. After receiving the Marie Curie Fellowship from the European Union, he joined the Autonomous University of Barcelona, Spain, in 2004, where he developed nanomaterial-based electrochemical biosensors. In the summer of 2006, he joined the National Institute for Materials Science, Japan, for a tenure-track arrangement and stayed there until Spring 2008 when he accepted a tenured position at NIMS. In 2009, Prof. Pumera received an ERC-StG grant to become group leader at EPFL, Switzerland. However, Prof. Pumera relinquished the ERC award and joined a faculty at the Nanyang Technological University, Singapore, in 2010. Prof. Pumera has broad interests in electrochemistry and electrophoresis, in the specific areas of microsystems and nanobiosystems. He is a member of the Editorial Boards of Electrophoresis and Journal of Ion Channels and an Associate Editor of Nanobioanalysis. |
1. Introduction
As nanotechnology moves from exploring a variety of new materials and their fabrication methods to functional nanomaterials, there is a pronounced interest in functional nanoarchitectonics.1 “Intelligent,” self-organizing nanomaterials, which follow the concept of sense-and-act, are at the forefront of current nanotechnology research.2 A very exciting area includes self-propelled autonomous sensing and actuating devices called nanosubmarines (also known as nanorobots, nanomotors, or nanorods).3 Such devices consist of a self-propelled body, which is equipped with sensing and/or actuating attachments, such as biorecognition elements, cargo loads, or electromagnetic field-responsive elements. The ultimate aim of research in this field is to create self-deployable nano/microdevices that are able to detect substances, decontaminate the environment, find and destroy malicious cells, perform targeted drug delivery, or engage in microsurgery. These devices can be propelled by several different mechanisms, such as self-electrophoresis,4 self-diffusiophoresis,5 or bubble ejection.6 There are excellent reviews that focus on the applications, movement control, and propulsion control of nanosystems.7 However, since the main propulsion mechanism of most nanosubmarines is self-electrophoresis (it means electrophoretic movement of nanosubmarine without applied external electric field), the topic has not been treated in detail from an electrophoretic point of view as would be required. We feel that a special minireview focused on self-electrophoresis mechanisms is needed in order to provide greater understanding of the topic and stimulate research in this area. It should be noted that other mechanisms, such as bubble recoil or diffusiophoresis are omitted from this review and we focus only on the self-electrophoretic mechanism.
2. Fundamentals of self-electrophoresis by electrochemically generated electroosmotic flow
The self-electrophoresis of nanosubmarines is based on electroosmotic flow on the surface of the nanodevice. The electroosmotic flow is generated by the device itself based on the electrochemical conversion of “fuel” present in the environment in which the nanosubmarine operates. Fig. 1 illustrates an example of such a transformation. For example, let's look at a Pt/Au bimetallic nanosubmarine. On the Pt/Au nanosub, the fuel, usually hydrogen peroxide, is oxidized to oxygen on the Pt segment, (electrons are withdrawn from the hydrogen peroxide to the rod) while on the Au segment the hydrogen peroxide is reduced to water (electrons are transferred from the Au section of the nanosub to H2O2).4 The total amount of electrons in the nanosub is, of course, balanced but there is movement of electrons from the Pt to the Au segment, meaning that the bimetallic nanorod acts as a short-circuited electrochemical cell. As the electrons move from one rod section to the other, their movement is compensated by the movement of protons (resp. hydrated protons, H3O+ ions) on the surface of the nanosub, which are also generated/consumed in the reaction with the rods. Movement of the hydroniums and/or other positive ions drags the liquid close to the layer via viscosity forces and creates an electroosmotic flow on the surface of the nanosubmarine. The nanosub consequently moves in the opposite direction. In this section, we first focus on a detailed description of the mechanism of the self-electrophoretic movement, on the factors that can influence such movement, and their implications.
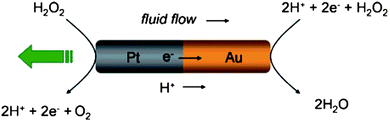 |
| Fig. 1 Electrochemical mechanism behind self-electrophoresis of nanosubs. Hydrogen peroxide is oxidized to generate protons in solution and electrons in the wire on the Pt end. The protons and electrons are then consumed with the reduction of H2O2 on the Au end. The resulting ion flux induces motion of the nanosub relative to the fluid, propelling the nanosub toward the platinum end with respect to the stationary fluid. Reprinted with permission from ref. 14. | |
2.1. Mobility of electroosmotic flow on the surface of a nanosubmarine
The velocity of the electroosmotic flow (veo) on the surface of the nanosub is directly related to the velocity of the nanosub in the opposite direction and is proportional to the mobility (μeo) of the electroosmotic flow and to the potential difference (E) between the ends of the nanosub according to eqn (1).8
Because the potential difference can be independently regulated by using segments of different compositions, we will discuss the mobility of electroosmotic flow instead of its velocity.
The mobility of electroosmotic flow is given by the von Smoluchowski equation9:
| 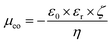 | (2) |
where
ε0 is the permittivity of vacuum,
εr is the relative permittivity of the medium,
η is its viscosity, and
ζ is the zeta potential on the surface. As can be seen, there are three variables on which electroosmotic mobility depends and which reflect the properties of the
solvent.
2.1.1. Influence of the medium.
Considering eqn (2), electroosmotic mobility should increase linearly with ε/η (considering a constant zeta potential). Table 1 lists dielectric constants and viscosities of commonly used media.10
Table 1 Relative permittivity and viscosity of various solvents.10
Therefore, the mobility of the electroosmotic flow on the surface of a nanosub in different solvents will increase in the following order:
EtOH < DMSO < PC < DMA < DMF < MeOH < H2O < ACN |
The aqueous solutions, which are commonly used as media for the operation of nanosubmarines, provide one of the highest electroosmotic flow mobilities.
2.1.2. Influence of viscosity.
The viscosity of the very same medium can be significantly influenced by the presence of other molecules. The presence of glucose, the use of which is suggested as an alternative biofuel for realistic nanosubmarine applications (because it is non-toxic when compared to the more commonly used hydrogen peroxide and which can be easily converted to H2O2 on demand of a nanosubmarine by enzymatic reaction), significantly increases the viscosity of the medium and decreases the mobility of the electroosmotic flow generated on the surface of the nanosubmarine, consequently decreasing the velocity of the nanosubmarine. See Table 2 for an example of how the wt% of D-glucose influences the viscosity of its aqueous solution. Fig. 2 shows the influence of the concentration of H2O2 in water on the viscosity of the solution. This will be considered when using new fuels and deploying nanosubs in new operational environments.
Table 2 Influence of D-glucose concentration in water on the viscosity of the solution.11
Mass (%) |
η (mPa s) |
0.5 |
1.010 |
1 |
1.021 |
5 |
1.145 |
10 |
1.330 |
20 |
1.904 |
30 |
2.998 |
40 |
5.493 |
50 |
11.884 |
60 |
37.445 |
In addition, the increased viscosity will influence the drag force – the resistance of the movement of the nanosub through the environment. The resistance (drag force) for objects moving at a low Reynolds number is given as13:
| Fd = 6 × π × η × r × v | (3) |
where
r is the diameter of the object (nanosubmarine),
η is the viscosity of the
solvent, and
v is the velocity of the object. In a medium with a higher viscosity, the nanosub will move at a lower velocity because the drag force increases. To calculate the magnitude of drag force for a typical nanosubmarine whose
r is 100 nm,
η of water is 1 × 10
−3 Pa s, and
v is 10 μm s
−1. Therefore, the drag force is 1.88 × 10
−14 N or 18.8 fN. The force generated by the electrochemical/self-electrophoretic mechanism should be higher than the drag force.
2.1.3. Influence of ionic strength.
The zeta potential in eqn (2) is the electric potential in the interfacial double layer of the nanosub. Because the thickness of the double layer depends on the ionic strength of the solution, the electroosmotic flow velocity depends on the ionic strength. With increasing ionic strength, the mobility of the flow will decrease. To illustrate, the thickness of a double layer is about 10 nm for an ionic strength of 1 mM and about 0.3 nm for an ionic strength of 1 M. Therefore, with increasing ionic strength the mobility of the nanosub will decrease as the mobility of the generated flow decreases. This effect was demonstrated experimentally by Mallouk and Sen et al. who showed that with increasing ionic strength (and thus increasing conductivity of the media), the axial velocity of nanosubmarines decreases.14
2.2. Tailoring the potential difference: the electrochemical mechanism
As can be seen from eqn (1), electroosmotic velocity depends on the potential difference between the two metals of which the nanosub consists. It is possible to predict the direction of the bimetallic nanosub based on the values of standard electrochemical potentials of the half reactions of the respective metal (see Table 3). For example, a Ni–Au rod will move toward the Ni segment while a Co–Au rod will move toward the Au segment.4 Similarly, movement toward the Pt segment would be expected (and was experimentally observed) in a Pt/Au bimetallic nanosub.
Table 3 Standard electrochemical potentials.15
Metal |
Standard potential (V) |
Zn |
−0.76 |
Cr |
−0.74 |
Fe |
−0.44 |
Ni |
−0.24 |
Sn |
−0.14 |
Pb |
−0.13 |
H |
0 (by definition) |
Cu |
+0.34 |
Ag |
+0.80 |
Hg |
+0.86 |
Pt |
+1.20 |
Au |
+1.40 |
Co |
+1.81 |
However, from standard electrochemical potentials, it is not possible to predict the magnitude of the potential difference and consequential velocity of the nanosub because the kinetics of the catalytic reaction play a major role. The potential difference for a particular reaction of a particular fuel (hydrazine, hydrogen peroxide) on the surface of a metallic nanosub must be measured and determined, e.g., using a Tafel plot. A Tafel plot can also be used to confirm/predict the direction of the movement of the nanosub. This is shown in Table 4.
Table 4 Tracking data for bimetallic nanorods in 5 wt% aqueous H2O2 solution. Adapted from ref. 4 with permission.
Bimetallic nanorod |
Speed (μm s−1) |
Leading end (observed) |
Leading end (predicted from Tafel plot measurements) |
Rh–Au |
23.8 ± 2.9 |
Rh |
Rh |
Pt–Au |
20.0 ± 3.8 |
Pt |
Pt |
Pd–Au |
15.3 ± 2.0 |
Pd |
Pd |
Pt–Ru |
30.2 ± 4.0 |
Pt |
Pt |
Au–Ru |
24.0 ± 2.0 |
Au |
Au |
Rh–Pt |
17.0 ± 3.0 |
Rh |
Rh |
Rh–Pd |
16.2 ± 1.8 |
Rh |
Rh |
Pt–Pd |
13.6 ± 2.3 |
Pt |
Pt |
Ni–Au |
4.75 ± 1.1 |
Ni |
Ni |
Ag– Au |
6.20 ± 1.2 |
Ag |
|
Au–Co |
7.10 ± 1.4 |
Au |
|
It has been shown that introduction of carbon nanotubes to a Pt segment as a dopant significantly increased the speed of nanosubmarines, presumably due to increasing the roughness of the Pt segment and increasing the rate of the oxidation of hydrogen peroxide on the element.16 Ozin et al. reported the surface modulation of bimetallic Au–Ni nanomotors and demonstrated that it is proportional to the surface area of the catalytic segment of the nanorods.17 The performance of a Au segment can be tailored by introducing silver as a dopant to a Au segment.18
3. Methods
3.1. Fabrication of nanosubmarines
Nanosubmarines are typically bimetallic rods with diameters of more than 100 nm and lengths of several micrometres. The method of preparation of such bimetallic nanorods is based on electrochemical deposition (electroplating) of a particular metal on a template membrane.19 The diameter of the nanosubmarine is thus defined by the diameter of the membrane pore (typically alumina membranes with pore diameters of 100–500 nm), the length of the nanosub is defined by the membrane thickness. Currently, nanosubs have solid interiors; the shapes of the ends have not been altered in order to investigate their influence upon mobility. What can be varied is the length of a particular segment and its composition. The segments can be monometallic (Au, Pt, Ag, Ni, etc.)4 or metal alloys (i.e., Au/Ag)18 at different ratios or even metal composites with carbon-based nanomaterials such as carbon nanotubes.16 The segments can also be polymeric with integrated biomolecules.4 The composition of the segments has a large influence on the generated electrochemical potential and, therefore, on the velocity of the nanosub.
3.2. Fuels and their composition
At present, the most common fuel for the propulsion of self-electrophoretic nanosubmarines is hydrogen peroxide because it can be electrochemically oxidized at one end of a nanosub and electrochemically reduced at the opposite end of the nanosub.4 Increasing the concentration of hydrogen peroxide raises the power output of the nanosubmarine and consequently the speed increases. The experimental rate of oxygen formation from 3.7% hydrogen peroxide was found to be approximately 1/2000 of the limit imposed by the hydrogen peroxide diffusion rate.20 Thus, the rate of oxygen formation is limited by the turnover rate of the catalyst which, in turn, depends on the surface area of the nanorod segment.20 Note that it has been proposed that the main reduction reaction is the reduction of dissolved oxygen (O2) to water.4 It has been demonstrated that the addition of hydrazine into the hydrogen peroxide fuel can improve the power output of nanosubmarines.18 Different hydrazines (methyl, dimethyl, etc.) can potentially be used for tailoring the electroosmotic flow speed, in a manner similar to that in bimetallic micropumps.21
Because hydrogen peroxide and hydrazine are toxic compounds, there is a strong need to create nanosubmarines that utilize biocompatible fuels, especially fuels that can be found in the potential environment of their application, i.e., in living organisms. Biocompatible fuels were employed in conjunction with biocatalysts – enzymes. For example, a conductive carbon fiber was functionalized at one end with glucose oxidase and at the other end with bilirubin oxidase (see Fig. 3).22 Movement of the carbon fiber was caused by catalytic transformation of glucose to gluconic acid at the end modified with glucose oxidase and the flux of the electrons inside the rod and hydronium outside the rod, according to the reaction:
β-D-glucose → δ-glucono-1,5-lactone + 2H+ + 2e− |
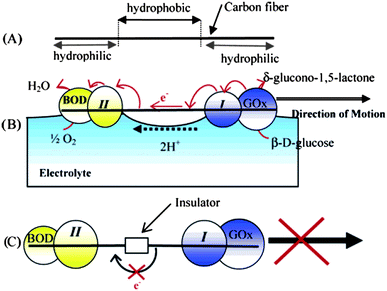 |
| Fig. 3 Use of biocompatible fuel – glucose – in conjunction with its transformation via an enzyme. (A) The two ends of a carbon fiber are made hydrophilic by exposure to a 1 Torr O2 plasma. (B) One end of the fiber is modified with the electrostatic adduct of glucose oxidase (GOx) and redox polymer I. The other end is modified with an electrostatic adduct of bilirubin oxidase (BOD) and redox polymer II. When the fiber is dipped in a pH 7 buffer solution containing 10 mM glucose, electrons flow along the path glucose → GOx → I → carbon fiber → II → BOD → O2, and the fiber is propelled at the solution–O2 interface by the ion flow that accompanies the flow of electrons. (C) When an insulator is introduced between the two electrocatalytic fiber ends, the fiber does not move because there is no flux of electrons inside the fiber and the protons on its surface. Reprinted with permission from ref. 22. | |
Glucose is one of the most promising biocompatible fuels because of its abundant presence in organisms. Glucose was used in a bubble recoil mechanism where enzymes glucose oxidase and catalase were attached to carbon nanotubes and they enabled the tandem catalytic conversion of glucose and H2O2 formed to power autonomous movement of the nanotubes.23 This mechanism could be in part used also for self-electrophoretic systems.
3.3. Modulation of output power
Here we describe how the output power can be modulated. We divide the modulation into two categories – static and dynamic – where static means that the modulation occurs before immersing the nanosubmarine in the solution and cannot be influenced after that. Dynamic modulation refers to the conditions where the output power can be changed while nanosubmarines are operating in the environment.
3.3.1. Static modulation.
Static variation of power output can be based on (a) different nanosub composition or (b) different fuel composition. Such variation leads to the potential differences noted above, and to a difference in propulsion force. The potential difference is easily measured electrochemically and visualized in the form of Tafel plot representation. (The Tafel plot shows electrode potential versus the logarithm of current density and provides information about the mechanism and rate of electrochemical reaction.)20 Such potential differences can be directly related to the velocity of the nanosubmarine. Notably, Sen et al.4 described the creation of bimetallic microrods with various metal combinations and measured the velocities and mixed potentials for different metals. The influence of the incorporation of carbon nanotubes in metallic half-rods and the fabrication of bimetallic alloy half-rods was studied by Wang et al.18 It was observed that incorporation of carbon nanotubes significantly increases the mobility of the nanosubs.
It has also been shown that the addition of silver ions to the solution increases the speed of nanosubmarines by a factor of five. Such an increase was attributed to the underpotential deposition of silver on the nanosub and an increase of the catalytic activity of such a segment.24
3.3.2. Dynamic modulation.
Fuel concentration can be dynamically varied electrochemically. The external electrode can locally replenish or deplenish the concentration of fuel (i.e., hydrogen peroxide) and consequently increase or decrease the speed of the nanosubmarine in close proximity to the electrode – within the diffusion layer of the electrode, which is typically several hundreds of μm.25 Another possible method is to vary the temperature of the fuel solution since, according to the Arrhenius equation, the rate of chemical reaction depends on temperature.26 With increased temperature, the rate of conversion of hydrogen peroxide increases and the speed of the nanosubmarine increases and vice versa.
3.4. Modulation of direction
The direction of the movement of self-propelled nanosubs can be modulated by the properties of the environment. It has been shown in various examples that when incorporating a Ni segment into the nanosub (i.e., between Au and Pt segments, see Fig. 4), such a nanosubmarine follows the orientation of an external magnetic field.27 It has also been shown that such self-propelled nanosubmarines with a Ni segment can be externally navigated in complex microfluidic channels (see Fig. 5).28
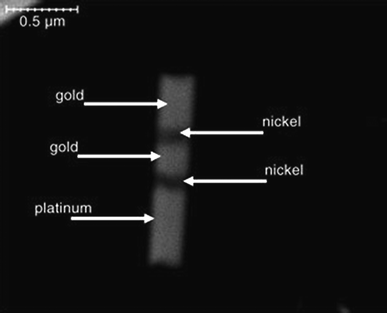 |
| Fig. 4 Incorporation of nickel segment into a nanosub for magnetic manipulation. SEM image at 35 000× magnification of 1.5 μm × 400 nm striped metallic rod. Respective segment sizes (nm): Au, 350; Ni, 100; Au, 200; Ni, 100; Pt, 550. Reprinted with permission from ref. 27. | |
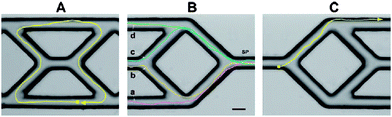 |
| Fig. 5 Navigating a nanosub in a complex microfluidic channel using an external magnetic field. Tracks of the directed nanosub movement within a microfabricated channel network. (A) Motion of an Au/Ni/Au/Pt-CNT nanomotor manoeuvring around the central portion of a PDMS microstructure; (B) Motion of the same nanowire over four different paths (a–d) beginning from the same starting point (SP); (C) Motion of a nanomotor in a mixed peroxide/hydrazine fuel. Fuels, 5 wt% H2O2 (A, B); 2.5 wt% H2O2/0.15 wt% hydrazine (C). Scale bar, 25 μm. Reprinted with permission from ref. 28. | |
Nanosubmarines can also navigate by themselves through materials with uniaxial magnetic anisotropy, biomimicking the navigation of magnetotactic bacteria (Magnetotacticum gryphiswaldense) (see Fig. 6).29 Such self-navigation (as opposed to externally guided navigation) offers great benefits for the automated movement of a large number of nanosubs.
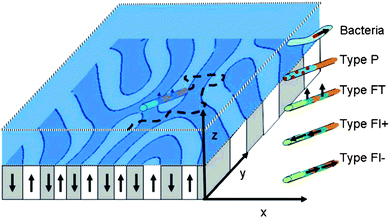 |
| Fig. 6 Self-navigation of nanosub on complex magnetic surface. Scheme of a magnetic garnet film with upward (white) and downward (gray) magnetized domains forming a labyrinth pattern. Autonomously moving navigators (i.e., magnetotactic bacteria, paramagnetic or ferromagnetic rods of type P, FT, FL+, and FL−) are placed in an aqueous solution above the garnet film where they autonomously move. The orientation of the navigators is partially governed by the magnetic heterogeneities of the film and by orientational fluctuations. Reprinted with permission from ref. 29. | |
3.5. Chemotaxis: follow the target
The ultimate goal of nanosubs is to sense-and-act: to find their target and perform an action. The sensing approach, contrary to the above-described modulation of direction by an external source (i.e., a magnetic field), relies on the ability of the nanosub to become oriented according to the inherent properties of its environment. This is an essential property for the autonomous behavior of nanosubs. The bimetallic nanosubmarines exhibit chemotaxis (movement) toward a gel soaked in 30% hydrogen peroxide (see Fig. 7).30 In another example, nanosubs were able to find the entrance of a capillary filled with concentrated fuel and climb vertically (the capillary had a wall thickness of 150 μm) up into the entrance of the capillary.30
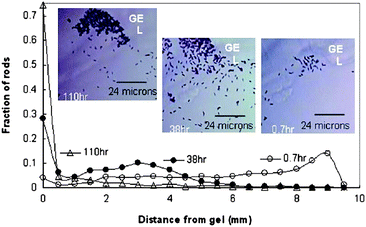 |
| Fig. 7 Chemotaxis of nanosubs. The changing distribution of Pt/Au nanosubs in a H2O2 concentration gradient. The gel (soaked in 30% H2O2) appears in the upper part. The images were taken at 0.7 h, 38 h, and 110 h. The fraction of rods was evaluated by dividing the number of nanosubs in a frame at a certain distance by the total number of nanosubs summed from the frames at all distances. The insets show the change in population of the Pt/Au rods near the gel, visualized under bright-field inverse microscopy at 50× magnification. Reprinted with permission from ref. 30. | |
3.6. Delivery
One of the targeted “actions” of nanosubs is delivery of functional cargo. The cargo can be attached to nanosubs covalently or by electrostatic interaction. Polystyrene beads functionalized with amino groups carrying an overall positive charge were electrostatically attached to a negatively charged polypyrrole (PPy) segment of Pt/Au/PPy nanowire.31 In a similar manner, streptavidin-functionalized polystyrene beads were attached to a biotin-functionalized gold segment of a Pt/Au nanowire (see Fig. 8).31 Such loaded nanosubmarines can perform chemotaxis towards hydrogen peroxide fuel. It was also demonstrated that the directed motion of catalytic nanosubmarines and their cargo transport and manipulation is possible along predetermined paths within microchannel networks.28 It can be expected that self-electrophoretic nanosubs will perform similarly complex actions as tubular bubble-jet engines, such as the directed assembly of nano/microstructures.32
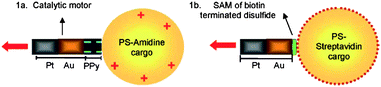 |
| Fig. 8 Nanosubs transporting cargo. Cargo attachment by (a) electrostatic interaction between the negative PPy end of a Pt-Au-PPy nanosub and a positively charged PS amidine microsphere and (b) biotin–streptavidin binding between the Au tips of a Pt-Au nanosub functionalized with a biotin-terminated disulfide and streptavidin-coated cargo. Reprinted with permission from ref. 31. | |
3.7. Collective behavior
For future applications, it is important to ensure that nanosubs communicate with themselves. While collective behavior is common in nature and has been demonstrated for chemo/phototaxing spheres,33 there is a limited number of examples of such behavior in the case of self-electrophoretic nanosystems. It has been demonstrated for fast rotating, self-electrophoretic nanosubs that two-co-rotating rods attract each other within a distance of around ∼1 μm and no collisions were observed while counter-rotating nanosubs often attract each other from much closer and collide (see Fig. 9).34 Further investigation of various possible collective behaviors is important for nanosub applications.
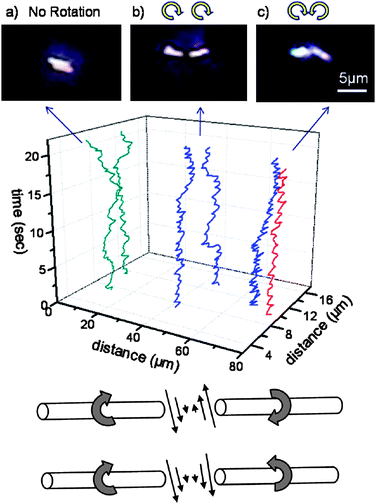 |
| Fig. 9 Collective behavior of nanosubs. (Top) Two-dimensional trajectories of nanosub pairs and corresponding optical images at their closest center-to-center distances: (a) unpowered nanosubs in deionized water; (b) nanosubs rotating in the same direction in 15% H2O2; (c) oppositely rotating rods in 15% H2O2. The z-axis represents time, and the x- and y-coordinates represent the position of the center of each rod. (Bottom) Schematic drawing of the fluid velocity distribution between the tips of the nanosubs. Reprinted with permission from ref. 34. | |
4. Conclusion and outlook
Electrochemically powered self-electrophoretic nanosubmarines have passed through the first stage of development as it was demonstrated how the composition of the nanosubs and the environment and influence of the external field can influence movement. However, it should be noted that many of these studies are proof-of-concept, simple systems, which are likely far simpler than what is needed for realistic applications.
Much remains to be done on basic, fundamental research on the movement of nanosubs and several questions remain. What is the influence of the nanosub shape on nanosub movement? Would the self-electrophoretic propulsion work for fully biofunctionalized nanosubs? Since the nanosub consists of two metals is there any dissolution of the metal components (galvanic nanocorrosion)? This is very important for assessing any potential nanotoxicity of nanosubs since nickel as well as silver is a toxic metal. Can nanosubs cooperate and show collective behavior and signaling?
From the application point of view, other equally important questions must be addressed. Is it possible to use nanosubs as a detection device for nanoanalytical chemistry? Can we create nanosubs which do find the analyte via analyte concentration gradient and report its concentration to us? Is it possible to employ nanosubs in the nanoassembly of inorganic and/or biologic building blocks? Can nanosubs operate within cells? What is the influence of the real media on the surface of nanosubs and on their mobility? Can we make nanosubs biocompatible and biodegradable? Can we employ alternative biofuels? Ultimately, can we integrate a nanobrain35 on nanosubs?
Acknowledgements
This work was partially supported by a NAP start-up fund (grant no. M58110066) provided by NTU. M. P. is grateful to Mr. Jiří Klíma (Czech Republic) for cover image design.
Notes and references
-
(a) G. M. Whitesides and D. J. Lipomi, Faraday Discuss., 2009, 143, 373 RSC;
(b) K. Ariga, X. Hu, S. Mandal and J. P. Hill, Nanoscale, 2010, 2, 198 RSC.
- Y. Hong, D. Velegol, N. Chaturvedic and A. Sen, Phys. Chem. Chem. Phys., 2010, 12, 1423 RSC.
- T. Mirkovic, N. S. Zacharia, G. D. Scholes and G. A. Ozin, Small, 2010, 6, 159 CrossRef CAS.
- Y. Wang, R. M. Hernandez, D. J. Bartlett, J. M. Bingham, T. R. Kline, A. Sen and T. E. Mallouk, Langmuir, 2006, 22, 10451 CrossRef CAS.
- J. R. Howse, R. A. L. Jones, A. J. Ryan, T. Gough, R. Vafabakhsh and R. Golestanian, Phys. Rev. Lett., 2007, 99, 048102 CrossRef.
- A. A. Solovev, Y. F. Mei, E. Bermudez Urena, G. Huang and O. G. Schmidt, Small, 2009, 5, 1688 CrossRef CAS.
-
(a) W. Paxton, S. Sundararajan, T. Mallouk and A. Sen, Angew. Chem., Int. Ed., 2006, 45, 5420 CrossRef CAS;
(b) S. Sanchez and M. Pumera, Chem.–Asian J., 2009, 4, 1402 CrossRef CAS;
(c) A. Sen, M. Ibele, Y. Hong and D. Velegol, Faraday Discuss., 2009, 143, 15 RSC;
(d) S. J. Ebbens and J. R. Howse, Soft Matter, 2010, 6, 726 RSC.
-
D. C. Harris, Quantitative Chemical Analysis, W.H. Freeman, New York, 2007, p. 607 Search PubMed.
- M. von Smoluchowski, Bull. Int. Acad. Sci. Cracowie, 1903, 194 Search PubMed.
- Adapted from:
(a)
Y. Marcus. Ion solvation, Wiley, Chichester, 1985 Search PubMed;
(b) J. Muzikář, T. van de Goor and E. Kenndler, Anal. Chem., 2002, 74, 434 CrossRef CAS;
(c) J. Wang and M. Pumera, Anal. Chem., 2003, 75, 341 CrossRef CAS.
-
Handbook of Chemistry and Physics, 90th Edition, CRC Press 2009, pp. 8–59 Search PubMed.
-
C. W. Jones, J. H. Clark, Applications of Hydrogen Peroxide and Derivatives, Royal Society of Chemistry, 1999, pp. 17 Search PubMed.
-
P. Atkins and J. de Paula, Atkins' Physical Chemistry, Oxford University Press, 2010, pp. 761 Search PubMed.
- W. F. Paxton, P. T. Baker, T. R. Kline, Y. Wang, T. E. Mallouk and A. Sen, J. Am. Chem. Soc., 2006, 128, 14881 CrossRef CAS.
-
P. Atkins and J. de Paula, Atkins' Physical Chemistry, Oxford University Press, 2010, pp. 929 Search PubMed.
- R. Laocharoensuk, J. Burdick and J. Wang, ACS Nano, 2008, 2, 1069 CrossRef CAS.
- N. S. Zacharia, Z. S. Sadeq and G. A. Ozin, Chem. Commun., 2009, 5856 RSC.
- U. K. Demirok, R. Laocharoensuk, K. M. Manesh and J. Wang, Angew. Chem., Int. Ed., 2008, 47, 9349 CrossRef CAS.
- B. R. Martin, D. J. Dermody, B. D. Reiss, M. Fang, L. A. Lyon, M. J. Natan and T. E. Mallouk, Adv. Mater., 1999, 11, 1021 CrossRef CAS.
- W. F. Paxton, K. C. Kistler, C. C. Olmeda, A. Sen, S. K. St. Angelo, Y. Cao, T. E. Mallouk, P. E. Lammert and V. H. Crespi, J. Am. Chem. Soc., 2004, 126, 13424 CrossRef CAS.
- M. E. Ibele, Y. Wang, T. R. Kline, T. E. Mallouk and A. Sen, J. Am. Chem. Soc., 2007, 129, 7762 CrossRef CAS.
- N. Mano and A. Heller, J. Am. Chem. Soc., 2005, 127, 11574 CrossRef CAS.
- D. Pantarotto, W. R. Browne and B. L. Feringa, Chem. Commun., 2008, 1533 RSC.
- D. Kagan, P. Calvo-Marzal, S. Balasubramanian, S. Sattayasamitsathit, K. M. Manesh, G.-U. Flechsig and J. Wang, J. Am. Chem. Soc., 2009, 131, 12082 CrossRef CAS.
- P. Calvo-Marzal, K. M. Manesh, D. Kagan, S. Balasubramanian, M. Cardona, G.-U. Flechsig, J. Posnerc and J. Wang, Chem. Commun., 2009, 4509 RSC.
- S. Balasubramanian, D. Kagan, K. M. Manesh, P. Calvo-Marzal, G.-U. Flechsig and J. Wang, Small, 2009, 5, 1569 CrossRef CAS.
- T. R. Kline, W. F. Paxton, T. E. Mallouk and A. Sen, Angew. Chem., Int. Ed., 2005, 44, 744 CrossRef CAS.
- J. Burdick, R. Laocharoensuk, P. M. Wheat, J. D. Posner and J. Wang, J. Am. Chem. Soc., 2008, 130, 8164 CrossRef CAS.
- P. Dhar, Y. Cao, T. Kline, P. Pal, C. Swayne, T. M. Fischer, B. Miller, T. E. Mallouk, A. Sen and T. H. Johansen, J. Phys. Chem. C, 2007, 111, 3607 CrossRef CAS.
- Y. Hong, N. M. K. Blackman, N. D. Kopp, A. Sen and D. Velegol, Phys. Rev. Lett., 2007, 99, 178103 CrossRef.
- S. Sundararajan, P. E. Lammert, A. W. Zudans, V. H. Crespi and A. Sen, Nano Lett., 2008, 8, 1271 CrossRef CAS.
- A. A. Solovev, S. Sanchez, M. Pumera, S. Schulze, Y. Mei and O. G. Schmidt, Adv. Funct. Mater., 2010 DOI:10.1002/adfm.200902376.
- M. Ibele, T. E. Mallouk and A. Sen, Angew. Chem., Int. Ed., 2009, 48, 3308 CrossRef CAS.
- Y. Wang, S. Fei, Y.-M. Byun, P. E. Lammert, V. H. Crespi, A. Sen and T. E. Mallouk, J. Am. Chem. Soc., 2009, 131, 9926 CrossRef CAS.
- A. Bandyopadhyay and S. Acharya, Proc. Natl. Acad. Sci. U. S. A., 2008, 105, 3668 CrossRef CAS.
Footnote |
† In memory of Karel Zeman, Czech animator, who encouraged thousands of young people into science and technology, on the occasion of the 100th anniversary of his birth. |
|
This journal is © The Royal Society of Chemistry 2010 |