DOI:
10.1039/C0NR00092B
(Review Article)
Nanoscale, 2010,
2, 1842-1848
Received
7th February 2010
, Accepted 21st May 2010
First published on
2nd August 2010
Abstract
Recent developments on biomineralization and biomaterials have demonstrated that proteins play an important role in the formation of biominerals in the body, which induce or inhibit mineralization of calcium phosphate together with modulation of the mineral phase structure. Many efforts have been made to understand the mechanism of this process and mimic the exquisite structure of biominerals in biomimetic methodologies. This review is focused on recent advances in the synthesis of calcium phosphate materials by taking advantage of protein assemblies. We try to review the examples of templates based on proteins and polypeptides that have been successfully employed to manufacture calcium phosphate nanomaterials.
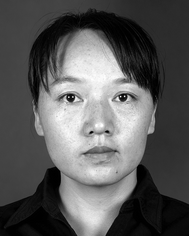 Yurong Cai | Yurong Cai studied materials science and engineering for her BSc degree in 1995 at Northeast University, China and completed her PhD on inorganic biomaterials (2004) from the Xi'an Jiaotong University under the supervision of Prof. Lian Zhou. She joined Prof. Ruikang Tang's group in Zhejiang University as a postdoctoral research associate. She has authored and co-authored 40 refereed journal publications, one book chapter, and four patents. She is currently an associate professor at College of Materials and Textile, Zhejiang Sci-Tech University. Her research focuses on the synthesis and assembly of nanostructured inorganic biomaterials and their related properties. |
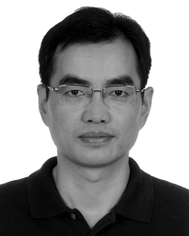 Juming Yao | Juming Yao obtained his PhD in 2003 from Department of Biotechnology, Tokyo University of Agriculture and Technology in Japan, followed by postdoctoral research on the synthesis and structural analysis of proteins at Venture Business Laboratory (TUAT). He returned to China in 2004 and he is now a professor in College of Materials and Textile, Zhejiang Sci-Tech University. His research interests include the design and biosynthesis of functional proteins and the protein-based nanocomposites. |
1. Introduction
Biomineralization is a rather old process, by which minerals with highly optimized properties formed under ambient environments.1 Organisms demonstrate their ability to find the most accessible methodology for the synthesis of functional materials after a rigorous process of natural selection,2,3 which has attracted a lot of attention recently in the cutting-edge areas of materials chemistry and its related fields, because it is not only important to gain an understanding of how mineral-rich tissues are created in vivo but also a great source of inspiration for the design of advanced materials.4 Although the mechanisms of in vivo crystallization processes are not yet known at a molecular level, it is believed that the organic templates play a key role in the formation of inorganic matter,5 by which so-called biomimetic synthetic or bioinspired research has been developed. During the past decades, many inorganic crystals or hybrid inorganic/organic materials with special sizes, shapes, organization, complex forms and hierarchies have been synthesized via bio-inspired methods with the assistance of various templates, such as synthetic polymers, self-assembling peptide, protein, and some low mass surfactant molecules.6–8 Several excellent reviews on the growth and assembly of inorganic crystal regulated by synthetic templates have been published from different viewpoints and focuses;9–13 for example, creating material structures using protein structured scaffolds, producing mesoporous structures materials via biomimetic mineralization, and summarizing experimental knowledge about the interactions of proteins with solid surfaces, etc.
Calcium phosphates, notably hydroxyapatite [HA, Ca10(PO4)6(OH)2], the main inorganic component of the hard tissues, exhibit many levels of hierarchical structures from the macro to nano scales.14 Despite their complicated hierarchical structures, the smallest building blocks in these biomaterials are generally on the nanometre size scale. For example, tens to hundreds of nanometre-sized calcium phosphate crystals in the collagen matrix combine into self-assembled apatite during bone and enamel formation. Recent advances suggest nanostructured materials provide the capability for specific interactions with proteins and hold optimal mechanical properties together with the organic components.15,16 It is believed that the formation of calcium phosphates with specific sizes, shapes and assembly methods has been regulated by two kinds of protein, i.e., structurally insoluble proteins and functionally soluble proteins, both of which are important for the modulation of the crystallization process.17 The former provides a scaffold for the subsequent mineralization process and the latter is usually used as the crystal modifier for controlled crystallization. The strategy has been used to design and fabricate novel biomedical materials with biomimetic structures and properties for therapeutic approaches in the repair of human tissues. So, this review is focused on recent advances in the synthesis and assembly of calcium phosphate nanomaterials mediated by insoluble and functional soluble proteins, including bone-related and tooth-related proteins, silk proteins and synthetic peptides. We also try to elucidate some concepts and mechanisms during the process.
Natural bone is of a complex nano-fibril system with an intricate hierarchical structure of mineralized collagen, which includes an orderly deposition of HA minerals within collagen matrix. More than 20 human collagens have been reported in the human body, among which type I collagen (Col) is the most abundant protein and provides much of the structural integrity for connective tissue, particularly in bones, tendons and ligaments. So, Col has been widely used as an organic composite to fabricate bone graft mimics because many researchers had assumed that a combination of HA and Col was the best for bone materials.18 Earlier studies focused on the simulation for the composition of bone and the improvement of distribution of HA phases in Col matrix. For example, Moreau et al.19 prepared a collagen-reinforced self-setting HA cement, and reported its high biocompatibility and mechanical properties. However, such HA/Col composite had only similar chemical composition to bone, which resulted in their poor bone metabolism and mechanical properties compared to biological bone. Recently, further effort has been done in order to simulate elaborate nanostructure of bone. For example, Kikuchi et al.20 prepared HA/Col composite by a simultaneous titration coprecipitation method. The composite showed a self-organized nanostructure, in which the c-axis of blade-shape HA nanocrystals with 50–100 nm in size were aligned along Col fibers up to 20 μm in length by the chemical interaction between HA and Col. Cui et al.21,22 found that the recombinant human-like type I collagen can direct the growth of HA nanocrystals in vitro. The mineralized collagen fibrils aligned parallel to each other to form mineralized collagen fibers. HA nanocrystals grew on the surface of these collagen fibrils with the c-axis of nanocrystals of HA orienting along the longitudinal axis of the fibrils, which was similar to the microstructure of natural bone at a higher level (Fig. 1). They have pointed out that the binding of calcium ions on the negatively charged carboxyl groups (–COOH) of collagen is the first-step in nucleation of HA crystals.23 In a neutral solution, more than 99% of the carboxyl groups of aspartyl and glutamyl ionize to COO−, which favors chelation of calcium ions and induced subsequent collagen mineralization in certain sites. The FTIR investigation and molecular modeling results have, furthermore, supported the above viewpoint.24
Recently, there is an increasing evidence that noncollagenous matrixes proteins play a significant role during the process of bone formation, including osteocalcin, fibronectin, and osteonectin etc. Though the precise controlled process about these proteins has not been completely understood, some consensus has been reached. For example, the osteocalcin influences bone mineralization in part through its ability to bind with high affinity to the mineral component of bone, HA, which is probably more important for remodeling.25,26 The effect of fibronectin on the bone mineralization could be used as both a nucleator and an inhibitor, which depended on the circumjacent environment. For example, Padrines et al.27 tested the mineralization potential of fibronectin and found that it could inhibit the precipitation of calcium phosphate crystals in solution but had no apparent effect in the gel state. If HA seeds are present in the gel, the fibronectin could favor the formation of apatite crystals. Similar results has been obtained by Daculsi et al.,28 who has obtained the precipitation of an apatite crystal using a solution of human fibronectin incubated in the presence of a calcium phosphate crystal, suggesting that the fibronectin may serve as a nucleating protein in early calcification in the presence of calcium phosphate crystals (secondary nucleation). The osteopontin-regulated precipitation of calcium phosphate has been performed in a vascular smooth muscle cell, an inhibitory role of osteopontin on the synthesis of calcium phosphate have been found.29,30
3. Tooth-related proteins
Amelogenin, the main secretory product of ameloblasts, typically consist of 180 amino acid residues, rich in proline, glutamine, leucine and histidine.31 The protein is mostly hydrophobic and can spontaneously form nanospheres with a hydrodynamic radius of approximately 10–25 nm under a wide variety of conditions.32–34 Although it presents in relatively large amounts in the early stages of enamel deposition and then is degraded and removed as the HA crystals develop, amelogenin is generally considered to be the most influential of the enamel matrix protein for mineral growth. A number of in vitro studies have been undertaken to elucidate the possible roles of amelogenin for the formation of enamel.35–37 For instance, according to the numbers of theoretical and experimental data, Du et al.38 discovered that the amelogenin nanospheres have been used as the monomers or oligomers to associate with one another to form aligned chains of a preferred length of 10–15 nanospheres. The structures further assemble into a ribbon structure with ordered domains, which facilitate the organization of inorganic nanostructures in developing enamel crystals through effecting initial mineral deposition and orienting nucleation of calcium phosphate crystals. Although there is no directly evidence that enamel mineralization in vivo occurs via heterogeneous sites of metastable calcium phosphate phases, Moradian-Oldak et al.39,40 found that amelogenin could control the basic habit of the initial crystals and subsequent growth morphology of calcium phosphate through the selective interaction with the (010) face, followed by the (001) and (100) faces. They suggested that the charged amino acids in the C-terminal domain are exposed at the nanosphere surface, promoting amelogenin–calcium phosphate interaction. Tarasevich et al.41 studied the effect of recombinant mouse amelogenin on the nucleation and growth of calcium phosphate in vitro. They found that the amelogenin promoted the heterogeneous nucleation of calcium phosphate from a supersaturated solution of calcium phosphate. The amount of precipitation depended on the solution supersaturation and protein concentration. Recently, Tang et al.42 studied the role of the amino acid for the formation of bionic materials. They found that the highly ordered enamel-like and bone-like apatites could be constructed in an hierarchical fashion in the presence of glycine and glutamate, respectively. The formation of enamel-liked apatites has been repeated; using bovine amelogenin showed dramatic acceleration, which was about 20-fold faster than with glycine even with an unusually low concentration. Similar results have been obtained by Wang et al. (Fig. 2).43 They studied the effect of amelogenin on HA nucleation kinetics in a constant composition system and found that in the absence of amelogenin, the induction time for HA nucleation was 778 ± 30 min and the HA nanocrystallies grow uncontrollably and aggregate randomly, however, in the presence of 5.0 μg mL−1 amelogenin, the induction times decreased to 255 ± 20 min and thicker apatite crystals were grown and they retained their elongated morphology. Their results implied that the amelogenin accelerated the HA nucleation kinetics, decreasing the induction time in a concentration-dependent manner in a near-physiological environment, which provided the direct experimental evidence that the amelogenin promotes apatite crystallization and organization.
In addition to the amelogenin, the extracellular matrix (ECM) of dentin contains numerous non-collagenous proteins (NCPs). These NCPs are believed to promote actively and control the mineralization of collagen fibers and crystal growth within the osteoid and predentin.44 Dentin matrix protein 1 (DMP1), a prominent member of one category of NCPs, has been paid lots of attention due to its unusually large number of acidic domains, which implicates it as a possible participant in regulating the mineralization.45,46 The effect of DMP1 on calcium phosphate has been proved by recent researches in vitro. For example, Gen et al.47 prepared plate-like HA regulated by DMP1 (Fig. 3). They found that the HA crystallization mediated by rDMP1 was a sequential or stepwise process. With increased of mineralization time, the morphology of precipitation changed from a lack of a distinct shape to a porous structure and then a plate-like structure accompanied with a process of crystal ripening. The controlled nucleation of HA in vitro begins by DMP1 binding calcium ions and initiating mineral deposition, among which the intermolecular assembly of acidic clusters of DMP1 into a β-sheet template was essential for the mineral nucleation.
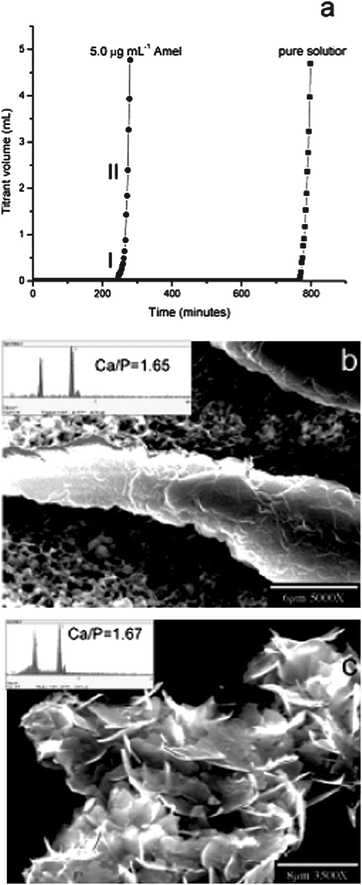 |
| Fig. 2 CC HA crystal growth. (a) Representative CC plots of titrant addition as a function of time for HA crystal growth in the absence and presence of 5.0 μg mL−1 amelogenin. SEM images of (b) an ordered and thickened crystal induced by 5.0 μg mL−1 amelogenin and (c) randomly aggregated HA crystallites in the absence of amelogenin collected from the bulk solution by filtration following the long induction periods. The inset in (b) is the EDS of the elongated crystals showing a HAP Ca/P ratio of 1.65. Reprinted with permission from ref. 43. | |
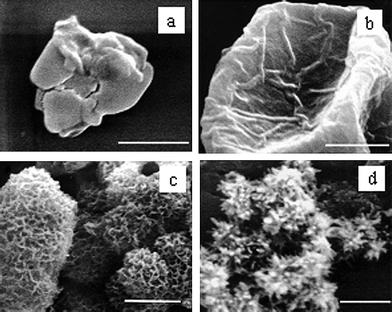 |
| Fig. 3 Sequential appearance of deposited particles accompanying the hydroxyapatite nucleation and growth process. (a) During the first several hours after the start of nucleation, only amorphous calcium phosphate was observed. (b, c) From day one to day three, a series of intermediate structures were observed. (d) After one week of incubation, only plate-shaped hydroxyapatite crystals were observed. Each scale bar = 2 μm. Reprinted with permission from ref. 44. | |
Subsequently, different effects of DMP1 with various functionalities and origin on the synthesis of calcium phosphate have been reported.48,49 For example, it was proved that the non-phosphorylated form of DMP1 made from prokaryotes could improve the nucleation of HA crystals, whereas the phosphorylated form had no apparent effect on the HA formation and growth.50 Tartaix et al.51 reported that the effects of DMP1 derived from eukaryotes were more complicated, i.e., the full-length bovine DMP1 made by human bone marrow stromal cells was a potent inhibitor of mineralization, whereas the DMP1-C-terminal (57 kDa) fragment isolated from rat bone was a HA nucleator. The hierarchical assembly of HA at the nanoscale level has been fabricated under the cooperative interaction of type I collagen and DMP1 by Gajjeraman et al.52 During the process of HA formation, the amorphous calcium phosphate has been captured as an intermediate phase and then the precipitates transformed into the crystalline HA structure with outstanding mechanical properties.
Though artificial composites of Col/HA possess a greater similarity to natural bone, collagen derived from animal tissues may cause many concerns related to the quality, purity and immunological reaction in susceptible patients due to their nonhuman protein composition.53 Some new organic proteins have been selected as substitutes for Col. For this purpose, Bombyx mori (B. mori) silk fibroin (SF) has been paid a lot of attentions because of its excellent intrinsic properties utilizable in the biotechnological and biomedical fields, such as sutures, artificial ligaments and substrates for cell culture, as well as the importance of silkworm silks in the manufacture of high quality textiles.54–56 Some studies on the mineralization of SF has been conducted.57,58 For example, SF was employed by Kong et al.59 to regulate the mineralization of HA nanocrystals. The strong chemical interaction between HA nanocrystals and SF has been proved by FTIR results, which employed the mineralization ability of SF. Recently, we used SF films pretreated with different methods as the template for the HA crystal growth in order to study the effect of SF's surface structure on protein mineralization. These results showed that the HA crystals grew in an anisotropic manner due to the different distribution of carboxyl groups and amino groups on the surface of SF films.60
The insoluble properties and good biocompatibility of SF has been utilized to fabricate tissue engineering scaffolds. Nano-sized HA/SF composite powders prepared by co-precipitation were used as the raw material, which were then blended with SF solution in order to decrease the aggregation of HA powder and the inorganic–organic phase separation. The results showed that the nano-sized HA/SF composite powders were uniformly dispersed in the SF matrix (Fig. 4), which provided the scaffolds with enhanced compressive properties and improved cell biocompatibility.61,62
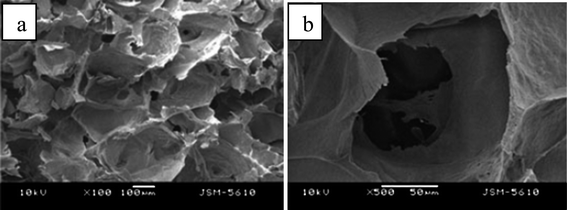 |
| Fig. 4 SEM images of modified HA/SF porous composite scaffolds with different magnification. Reprinted with permission from ref. 62. | |
Silk sericin (SS), a soluble protein in B. mori silkworm silk, has been applied widely in the cosmetic industry,63–65 such as a protective film for skin moisturizing and antiwrinkle treatments because of its excellent keratin-absorbing and water-absorbing properties. In particular, SS has been shown the ability to improve cell proliferation when used as an organic matrix or medium for cell growth,66–69 which offers the possibility to develop new applications of SS for biomedical materials with good biocompatibility. Recently, SS has been selected by us as an organic matrix to create an ordered structure of HA crystals in a biomimetic approach.70 The experimental results showed that higher SS concentration and longer mineralization time was helpful for the formation of larger HA crystals with higher crystallinity. In the presence of SS, the large HA crystals of 300–500 nm in length and 50–80 nm in diameter were assembled along the c-axis by small crystals of ca. 20 nm with the increase of reaction time, whose size and microstructure are similar to the natural enamel crystals (Fig. 5). The original spherical shape and subsequent conformational transition of SS may be two major reasons to modulate the enamel prism-like structure formation. In addition, the biocompatibility of the assembly was evaluated in vitro and its strong ability to promote cell differentiation and proliferation was proved.
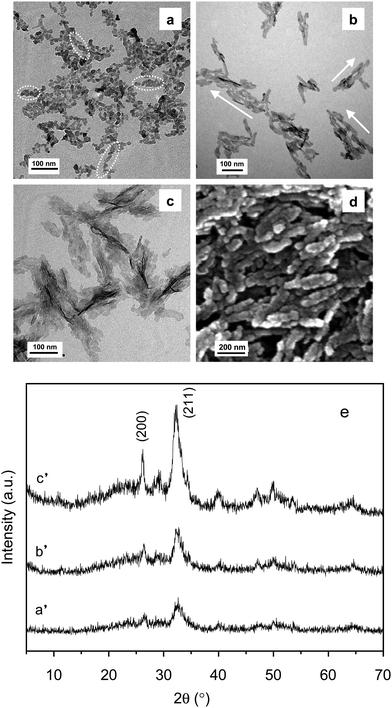 |
| Fig. 5 TEM images of HA crystals in the presence of SS after different mineralization times: (a) 0 min; (b) 20 min; (c) 60 min. (d) SEM images of sample (c); and (e) XRD patterns of the above-mentioned samples; a′, b′, c′ correspond to the a, b and c samples, respectively. Reprinted with permission from ref. 70. | |
During the process of protein-induced mineralization, some amino acid clusters and groups, such as carboxyl groups, or phosphorylated serine residues are known to strengthen the protein-regulated formation of minerals. Taking this inspiration, much attention has been given to developing self-assembling polypeptide amphiphiles, which could autonomously organize into certain patterns or structures without human intervention and allow the possibility of designing nanostructures that not only incorporate bioactive sequences but also specifically target mineralization.71 For the purpose, peptide amphiphiles (PAs), consists of a collagen sequence Gly-Val-Lys-Gly-Asp-Lys-Gly-Asn-Pro-Gly-Trp-Pro-Gly-Ala-Pro connected to a long-chain mono- or di-alkyl ester lipid, have been synthesized, which are known to self-assemble into sheets, spheres, rods, disks or channels depending on the shape, charge, and environment.72–74
Stupp et al.75–77 have developed nano-fiber forming PAs and obtained cylindrical micelle tubes (“nano-fibers”) similar to the architecture of collagen fibers. Subsequently, a bone biomimetic mineralization structure was prepared through introducing the PA nanofibers into a dilute solution of CaCl2 and Na2HPO4, which preferentially aligned with the long axis of the PA fibers, as in mammalian bone and dentin (Fig. 6). Their studies suggested that the anionic groups on the surface of organic templates are the nucleation sites of crystals and there is the similar correlation between the crystallographic orientation of HA and the organic scaffold.
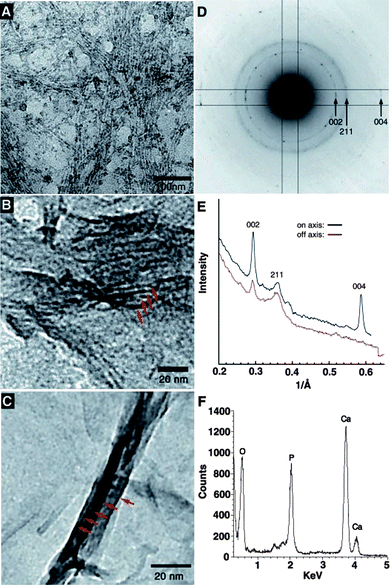 |
| Fig. 6 (A) TEM micrographs of the unstained, cross-linked peptide-amphiphile fibers incubated for 10 min in CaCl2 and Na2HPO4 solution. The fibers arranged in bundles are visible due to the high concentration of inorganic ions on their surface. (B) After 20 min, forming HA crystals (red arrows) are observed in parallel arrays on some of the PA fibers. (C) After 30 min, mature HA crystals (red arrows) completely cover the PA fibers. (D) Electron diffraction pattern taken from a mineralized bundle of PA fibers after 30 min of exposure to calcium and phosphate. The presence and orientation of the diffraction arcs corresponding to the 002 and 004 planes (whose intensities are enhanced with respect to the 211 family of reflections) indicate preferential alignment of the crystals with their c-axes along the long axis of the bundle. (E) Plot of intensity versus inverse angstroms reveals that the 002 and 004 peaks of hydroxyapatite are strongly enhanced along the peptide–amphiphile fiber axis. (F) EDS profile of mineral crystals after 30 min of incubation reveals a Ca/P ratio of 1.67 ± 0.08, as expected for HA. Reprinted with permission from ref. 75. | |
6 Conclusions
The synthesis and properties of calcium phosphate has always been paid a lot of attention due to its tight relationship to the health and life of human beings. Bio-inspired methodologies offer a great opportunity to produce calcium phosphate materials with improved properties. Both collagenous and noncollagenous matrix proteins including bone-related and tooth-related proteins have been introduced, all of which showed remarkable effects on the synthesis and assembly of calcium phosphate nanomaterials. As one of the most potential biomaterials, the silk proteins have been reviewed from the viewpoint of their effects on the synthesis and assembly of calcium phosphate materials. Self-assembling peptide amphiphiles have also been involved due to their increasing importance in the field. Recent developments demonstrated that these organics could be used as synthetic templates to regulate the nucleation and growth of calcium phosphate crystals. However, there is a lack of precise understanding on the nucleation, crystallization, self-assembly and growth mechanisms of complex structures, though the current trends and advances in the polypeptide design and synthesis promise a very high degree of control of product and further elucidation for the mechanism. The applications of protein-regulated calcium phosphate materials are sparse. Further multidisciplinary efforts are necessary to overcome the analytical difficulties for investigating a multistep reaction in the multicomponent system and observing the interface of inorganics/organics on a molecule level. In addition, the relationship between structural complexity and functional specialty of assembly materials should be explored in order to further expand the uses of materials into applications.
Acknowledgements
The work is financially supported by the Program for Changjiang Scholars and Innovative Research Team in University (IRT0654), Program for New Century Excellent Talents in University (NCET070763) and Zhejiang Natural Science Foundation of China (Y4090616).
References
-
S. Mann, Biomineralization: Principles and Concepts in Bioinorganic Materials Chemistry, Oxford University Press, New York, 2001 Search PubMed.
-
R. L. Reis and S. Weiner, Learning from Nature: How to Design New Implantable Biomaterials, Kluwer Academic Publishers. Netherlands, 2004 Search PubMed.
- S. Weiner, J. Struct. Biol., 2008, 163, 229 CrossRef CAS.
- S. H. Yu, Top. Curr. Chem., 2007, 271, 79 CAS.
- H. Cölfen, Top. Curr. Chem., 2007, 271, 1.
- T. Kato, T. Suzuki and T. Irie, Chem. Lett., 2000, 29, 186 CrossRef.
- C. Li and D. L. Kaplan, Curr. Opin. Solid State Mater. Sci., 2003, 7, 265 CrossRef CAS.
- S. Zhang, Nat. Biotechnol., 2003, 21, 1171 CrossRef CAS.
- L. C. Palmer, C. J. Newcomb, S. R. Kaltz, E. D. Spoerke and S. I. Stupp, Chem. Rev., 2008, 108, 4754 CrossRef CAS.
- F. Z. Cui, Y. Li and J. Ge, Mater. Sci. Eng., R, 2007, 57, 1 CrossRef.
- G. P. Whyburn, Y. J. Li and Y. Huang, J. Mater. Chem., 2008, 18, 3755 RSC.
-
J. H. Collier and P. B. Messersmith, Biomimetic Mineralization: Mesoporous Structures in Encyclopedia of Materials: Science and Technology, 2001, p. 602 Search PubMed.
- J. J. Gray, Curr. Opin. Struct. Biol., 2004, 14, 110 CrossRef CAS.
- A. P. Alivisatos, Science, 2000, 289, 736 CrossRef CAS.
- R. J. Narayan, P. N. Kumta, C. Sfeir, D. H. Lee, D. Olton and D. Chio, JOM, 2004, 56(10), 38 CrossRef CAS.
- H. J. Gao, B. H. Ji, I. L. Jager, E. Arzt and P. Fratzl, Proc. Natl. Acad. Sci. U. S. A., 2003, 100, 5597 CrossRef CAS.
- A. M. Belcher, X. H. Wu, R. J. Christensen, P. K. Hansma, G. D. Stucky and D. E. Morse, Nature, 1996, 381, 56 CrossRef CAS.
- D. Lickorish, J. A. M. Ramshaw, J. A. Werkmeister, V. Glattauer and C. R. Howlett, J. Biomed. Mater. Res., 2004, 68a, 19 Search PubMed.
- Jennifer L. Moreau, Michael D. Weir and Hockin H. K. Xu, J. Biomed. Mater. Res., Part A, 2009, 91a, 605 CrossRef.
- M. Kikuchi, T. Ikoma, S. Itoh, H. N. Matsumoto, Y. Koyama, K. Takakuda, K. Shinomiya and J. Tanaka, Compos. Sci. Technol., 2004, 64, 819 CrossRef CAS.
- L. J. Zhang, X. S. Feng, H. G. Liu, D. J. Qian, L. Zhang, X. L. Yu and F. Z. Cui, Mater. Lett., 2004, 58, 719 CrossRef CAS.
- W. Zhang, S. S. Liao and F. Z. Cui, Chem. Mater., 2003, 15, 3221 CrossRef CAS.
- B. Yang and F. Z. Cui, Curr. Appl. Phys., 2007, 7, e2 CrossRef.
- W. Zhang, Z. L. Huang, S. S. Liao and F. Z. Cui, J. Am. Ceram. Soc., 2003, 86, 1052 CrossRef CAS.
- K. Flade, C. Lau, M. Mertig and W. Pompe, Chem. Mater., 2001, 13, 3596 CrossRef CAS.
- M. Cozzzlino, A. S. Dusso and E. Slatopolsky, J. Am. Soc. Nephrol., 2001, 12, 2511.
- D. Couchourel, C. Escoffier, R. Rohanizadeh, S. Bohic, G. Daculsi, Y. Fortun and M. Padrines, J. Inorg. Biochem., 1999, 73, 129 CrossRef CAS.
- G. Daculsi, P. Pilet, M. Cottrel and G. Guicheux, J. Biomed. Mater. Res., 1999, 47, 228 CrossRef CAS.
- D. A. Pampena, K. A. Robertson, O. Litvinova, G. Lajoie, H. A. Goldberg and G. K. Hunter, Biochem. J., 2004, 378, 1083 CrossRef CAS.
- T. Wada, M. D. McKee, S. Steitz and C. M. Giachelli, Circ. Res., 1999, 84, 166 CAS.
- S. Habelitz, P. K. DenBesten, S. J. Marshall, G. W. Marshall and W. Li, Orthodont. Craniofacial Res., 2005, 8, 232 Search PubMed.
- H. H. Ravindranath, L. S. Chen, M. Z. David, R. Ishima and R. M. H. Ravindranath, Biochem. Biophys. Res. Commun., 2004, 323, 1075 CrossRef CAS.
- E. Beniash, J. P. Simmer and H. C. Margolis, J. Struct. Biol., 2005, 149, 182 CrossRef CAS.
- J. Moradian-Oldak, N. Bouropoulos, L. L. Wang and N. Gharakhanian, Matrix Biol., 2002, 21, 197 CrossRef CAS.
- X. D. He, L. B. Wu and S. Habelitz, J. Struct. Biol., 2008, 164, 314 CrossRef CAS.
- Y. Fan, Z. Sun, R. Wang, C. Abbott and J. Moradian-Oldak, Biomaterials, 2007, 28, 3034 CrossRef CAS.
- G. K. Hunter, H. A. Curtis, M. D. Grynpas, J. P. Simmer and A. G. Fincham, Calcif. Tissue Int., 1999, 65, 226 CrossRef CAS.
- C. Du, G. Falini, S. Fermani, C. Abbott and J. Moradian-Oldak, Science, 2005, 307, 1450 CrossRef CAS.
- M. Iijima and J. Moradian-Oldak, J. Mater. Chem., 2004, 14, 2189 RSC.
- N. Bouropoulos and J. Moradian-Oldak, Calcif. Tissue Int., 2003, 72, 599 CrossRef CAS.
- B. J. Tarasevich, C. J. Howard, J. L. Larson, M. L. Snead, J. P. Simmer, M. Paine and W. J. Shaw, J. Cryst. Growth, 2007, 304, 407 CrossRef CAS.
- J. H. Tao, H. H. Pan, Y. W. Zeng, X. R. Xu and R. K. Tang, J. Phys. Chem. B, 2007, 111, 13410 CrossRef CAS.
- L. J. Wang, X. Y. Guan, H. Y. Yin, J. Moradian-Oldak and G. H. Nancollas, J. Phys. Chem. C, 2008, 112, 5892 CrossRef CAS.
- A. George, B. Sabsay, P. A. Simnonian and A. Veis, J. Biol. Chem., 1993, 268, 12624 CAS.
- C. M. Li and D. L. Kaplan, Curr. Opin. Solid State Mater. Sci., 2003, 7, 265 CrossRef CAS.
- J. Huang, C. Wong, A. George and D. L. Kaplan, Biomaterials, 2007, 28, 2358 CrossRef CAS.
- G. He, T. Dahl, A. Veis and A. George, Nat. Mater., 2003, 2, 552 CrossRef CAS.
- Y. F. Ling, H. F. Rios, E. R. Myers, Y. B. Lu, J. Q. Feng and A. L. Boskey, J. Bone Miner. Res., 2005, 20, 2169 CrossRef CAS.
- M. L. Wallwork, J. Kirkham, H. Chen, S. X. Chang, C. Robinson, D. A. Smith and B. H. Clarkson, Calcif. Tissue Int., 2002, 71, 249 CrossRef CAS.
- C. Qin, R. D'Souza and J. Q. Feng, J. Dent. Res., 2007, 86, 1134 CrossRef CAS.
- P. H. Tartaix, M. Doulaverakis, A. George, L. W. Fisher, W. T. Butler, C. L. Qin, E. Salih, M. Tan, Y. Fujimoto, L. Spevak and A. L. Boskey, J. Biol. Chem., 2004, 279, 18115 CrossRef CAS.
- S. Gajjeraman, K. Narayanan, J. J. Hao, C. L. Qin and A. George, J. Biol. Chem., 2006, 282, 1193 CrossRef.
- Y. Zhai and F. Z. Cui, J. Cryst. Growth, 2006, 291, 202 CrossRef CAS.
- E. Rossitch Jr, North Carolina Med. J., 1987, 48, 669 Search PubMed.
- E. Rossitch Jr, D. E. Bullard and W. J. Oakes, Child’s Nervous System, 1987, 3, 375 Search PubMed.
- H. K. Soong and K. R. Kenyon, Ophthalmology, 1984, 91, 479 CAS.
- L. Meinel, R. Fajardo, S. Hofmann, R. Langer, J. Chene, B. Snyder, G. Vunjak-Novakovic and D. Kaplana, Bone, 2005, 37, 688 CrossRef CAS.
- C. Vepari and D. L. Kaplan, Prog. Polym. Sci., 2007, 32, 991 CrossRef CAS.
- X. D. Kong, F. Z. Cui, X. M. Wang, M. Zhang and W. Zhang, J. Cryst. Growth, 2004, 270, 197 CrossRef CAS.
- Y. C. Li, Y. Y. Cai, X. D. Kong and J. M. Yao, Appl. Surf. Sci., 2008, 255, 1681 CrossRef CAS.
- L. Liu, J. Y. Liu, M. Q. Wang, S. J. Min, Y. R. Cai, L. J. Zhu and J. M. Yao, J. Biomater. Sci., Polym. Ed., 2008, 19, 325 CrossRef CAS.
- L. Liu, J. Y. Liu, X. D. Kong, Y. R. Cai and J. M. Yao, Polym. Adv. Technol., 2009 DOI:10.1002/pat.1595.
- Y. Q. Zhang, Biotechnol. Adv., 2002, 20, 91 CrossRef CAS.
- C. Vepari and D. L. Kaplan, Prog. Polym. Sci., 2007, 32, 991 CrossRef CAS.
- R. Voegeli, J. Meier, R. Blust and R. Hofsteter, Cosmetics Toiletries, 1993, 108, 101 Search PubMed.
- N. Minoura, S. Aiba, Y. Gotoh, M. Tsukada and Y. Imai, J. Biomed. Mater. Res., 1995, 29, 1215 CrossRef CAS.
- K. Tsubouchi, Y. Igarashi, Y. Takasu and H. Yamada, Biosci., Biotechnol., Biochem., 2005, 69, 403 CrossRef CAS.
- S. Terada, T. Nishimura, M. Sasaki, H. Yamada and M. Miki, Cytotechnology, 2002, 40, 3 CrossRef CAS.
- S. Terada, M. Sasaki, K. Yanagihara and H. Yamada, J. Biosci. Bioeng., 2005, 100, 667 CrossRef CAS.
- Y. R. Cai, J. Jin, D. P. Mei, N. X. Xia and J. M. Yao, J. Mater. Chem., 2009, 19, 5751 RSC.
- P. X. Ma, Adv. Drug Delivery Rev., 2008, 60, 184 CrossRef CAS.
- Y. C. Yu, M. Tirrell and G. B. Fields, J. Am. Chem. Soc., 1998, 120, 9979 CrossRef CAS.
- P. Berndt, G. B. Fields and M. Tirrell, J. Am. Chem. Soc., 1995, 117, 9515 CrossRef CAS.
- Y. C. Yu, V. Roontga, V. A. Daragan, K. H. Mayo, M. Tirrell and G. B. Fields, Biochemistry, 1999, 38, 1659 CrossRef CAS.
- J. D. Hartgerink, E. Beniash and S. I. Stupp, Science, 2001, 294, 1684 CrossRef CAS.
- E. R. Zubarev, M. U. Pralle, E. D. Sone and S. I. Stupp, J. Am. Chem. Soc., 2001, 123, 4105 CrossRef.
- E. Beniash, J. D. Hartgerink, H. Storrie, J. C. Stendahl and S. I. Stupp, Acta Biomater., 2005, 1, 387 CrossRef.
|
This journal is © The Royal Society of Chemistry 2010 |
Click here to see how this site uses Cookies. View our privacy policy here.