DOI:
10.1039/B9NR00416E
(Paper)
Nanoscale, 2010,
2, 1244-1249
Glucose oxidase-doped magnetic silica nanostrutures as labels for localized signal amplification of electrochemical immunosensors
Received
(in Gainesville, FL, USA)
17th December 2009
, Accepted 15th February 2010
First published on
20th April 2010
Abstract
Herein, we report a novel glucose oxidase (GOD)-doped magnetic silica nanostructure and its possible application in the clinical immunoassays. The doped nanostructures were initially synthesized using the reverse micelle method, and ferritin antibodies (anti-Ft) were then labeled to the surface of the nanostructures, which were employed as signal antibodies for ultrasensitive detection of ferritin (Ft) in the sandwich-type electrochemical enzyme immunoassays. The doped nanostructures were characterized using transmission electron microscopy (TEM), UV-vis absorption spectrometry and vibrating sample magnetometer (VSM). The advantages of the doped nanostructures as labels were investigated in comparison with the conventional label method. Under the optimal conditions, the nanostructures-based immunoassay toward ferritin standards displays a wide dynamic range from 0.1 to 400 ng mL−1 with a low detection limit of 10 pg mL−1 ferritin (at 3σ), which is three-fold higher in the sensitivity than that of directly using GOD-labeled antibodies. The assay results for clinical serum samples with the developed method received in excellent accordance with results obtained from the referenced standard enzyme-linked immunosorbent assay (ELISA) method.
1. Introduction
Nanotechnology, the emerging research field of manipulating matter at the molecular or atomic level, has experienced tremendous growth on the successful development of new analytical tools and instrumentation for bioanalytical and biotechnological applications in recent years.1–4 The application of these nanobiotechnology in biosensing in place of conventional sensing technologies has led to improvements in sensitivity, selectivity, and multiplexing capacity.5–7 One major advantage using nanostructures is to control and tailor their properties to meet the needs of specific applications, such as good biocompatibility, high surface-to-volume ratio, and unique optical properties, in comparison with bulk materials.8 Recently, the critical role that dopants play in semiconductor devices has stimulated research on the properties and the potential applications of semiconductor nanostructures doped with intentional impurities.9 Because impurities could be used to alter the properties of nanoscale materials in desirable and controllable ways, doped nanostructures could address key problems in applications from solar cells to bioimaging.9
Various types of the doped nanostructures, such as luminophore-, magnetic particles-, semiconductor quantum dots and Raman active particles-doped nanostructures, have been preliminarily applied in bionanotechnology and biomedicine.10–14 Nanosized silica particles have been investigated intensively and proved to be an ideal protein host due to high chemical and thermal stability, large surface area, fine suspendability in aqueous solution, and good compatibility with the environment.15–17 The silica shell allows a wide variety of surface reactions to facilitate conjugation with biomolecules like proteins and DNAs.18–20 For the conventional sandwich-type enzyme immunoassays, the detection signal usually derives from the labelled enzyme conjugated with secondary antibodies. However, the sensitivity is usually low because of steric reasons usually a ratio of 1
:
1 for enzyme and detection antibody used.21 On the contrary, if more enzyme molecules are labeled to the detection antibodies, the sensitivity might be enhanced. Bioactive enzyme-doped silica nanoparticles possess several merits: high bioelectrocatalytic intensity, good biocompatibility, and good potential for surface modification with various biomolecules. Using this method, there are many enzyme molecules inside and outside of the synthesized bionanolabels. The carried enzyme molecules will be entered and participated in the catalytic reaction, suspecting, when one antibody among them reacts with the corresponding antigen.22 This high efficiency makes them especially suitable for ultrasensitive bioanalysis, and negates the need for additional reagents or signal amplification steps.23
Magnetic nanoparticles-doped nanostructures have attracted much research interest in the past decade due to their potential applications in magnetically guided site-specific drug and gene delivery systems, biolabelling, and bioseparation.24–26 These applications commonly require chemical stability and good dispersion of the magnetic nanoparticles in the liquid medium.27,28 Pure magnetic particles have some limitations, especially under in vivo conditions.29 For example, they tend to aggregate, they undergo biodegradation, which may be combined with magnetic property changes and finally, the metal can be toxic to the biological system.30,31 To overcome these shortcomings, the development of suitable biocompatible materials for surface coating of nanoparticles i.e. a core-shell structure is required. The inorganic core, e.g. iron oxide, can range from 4 to 10 nm is surrounded by an outer layer of shell wall. Magnetite-doped silica nanoparticles are considered one of the most ideal materials due to its reliable chemical stability in aqueous media, biocompatibility, and functionalities to be linked to biologically active species.32
The aim of this work is to synthesize a novel GOD-doped magnetic silica nanostructure, which was used for the electrochemical detection of ferritin (as a model) on the thionine/BSA-modified screen-printed carbon electrodes. The presence of magnetic silica nanostructures favors the rapid separation and purification of the doped nanostructures with the help of an external magnet. The silica shell was used for the doped substrate for the immobilization of the bioactive enzyme (GOD). The novel nanolabels were expected to enhance the sensitivity of the sandwich-type electrochemical immunoassays in this study.
2. Experimental details
2.1 Materials
Mouse monoclonal capture and signal anti-ferritin antibodies (anti-Ft, 100 μg mL−1) were purchased from Santa Cruz Biotechnology, Inc. (USA). Ferritin standard solutions were obtained from ELISA kits of ferritin. Magnetic Fe3O4 nanoparticles (particle size: 10 nm) in an aqueous suspension with a concentration of 25 mg mL−1 were obtained from Chemicell GmbH (Berlin, Germany). Glucose oxidase (GOD, EC 1.1.3.4, MW 160,000), thionine, glutaraldehyde, bovine serum albumin (BSA, 96%–99%), (3-glycidyloxypropyl) trimethoxysilane (C9H20O5Si, GPMS), and tetramethoxysilane (TEOS) were purchased from Sigma-Aldrich (St. Louis, MO, USA). Cyclohexane, n-hexanol, and ammonium hydroxide (25 wt.%) were purchased from Merck (Darmstadt, Germany). All other reagents were of analytical grade and were used without further purification. Deionized and distilled water was used throughout the study. Phosphate-buffered saline (PBS, 0.1 M) of various pHs were prepared by mixing of the stock solutions of Na2HPO4 and NaH2PO4. Graphite powder (No. 50870) was from Fluka. The solid paraffin substrates for screen-printed electrodes were from Chongqing Chem. Ltd. Co. Silver-based ink was from GEM-Gwent (Pontypool, UK).
2.2 Preparation of secondary antibodies-conjugated GOD-doped magnetic silica bionanocomposites
Prior to experiment, we synthesized the GOD-doped magnetic silica nanostructures at room temperature (RT) using a reverse micelle method as the following steps: (i) Fe3O4 aqueous solution (1.0 mL, 25 mg mL−1), Triton X-100 (5.34 g, original concentration), n-hexanol (5.13 mL, original concentration) and cyclohexane (22.5 mL, original concentration) were mixed into 50 mL of beaker flask, and vigorously stirred for 10 min; (ii) 1.0 mL of GOD aqueous solution (1.5 mg mL−1) was added in the mixture, and continuously stirred 10 min; (iii) 1.75 mL of TEOS and 2.35 mL of NH3·H2O (25 wt.%) were slowly dropped into the mixture, and vigorously stirred for 24 h; and (iv) 5.0 mL acetone was added, and the mixture was separated using an external magnet, and washed for 5 times with ethanol and water. The obtained GOD-doped magnetic silica nanoparticles were used for the label of secondary antibodies.
The conjugating process of the doped nanostructures with biomolecules was based on the epoxy-amino reaction. Prior to experiment, 1.0 g of the doped nanoparticles was dried in full automatic vacuum drying oven at 37 °C. Following that, 0.25 g of doped nanostructures was added in 5.0 mL of 5% GPMS (v/v) in dry toluene, and stirred with 750 rpm for 12 h at RT. The GMPS-modified doped nanoparticles were collected with an external magnet, and washed for 3–5 times with toluene and ethanol solution. To activate the epoxy groups on the nanoparticle surface, the purified nanoparticles were placed in an oven at 37 °C for 2 h with nitrogen atmosphere. Afterward, 1.0 mL of anti-Ft PBS solution (25 μg mL−1) was added the dried nanocomposites, and slightly stirred for 12 h at 4 °C to form the epoxy-amine bond. Finally, the antibody-conjugated magnetic silica nanoparticles (denoted as bionanocomposites) were separated and blocked for 1 h at RT with BSA (2%, w/v) + Tween 20 (0.05%, w/w). The synthesized bionanocomposites were stored in 5.0 mL pH 7.4 PBS at 4 °C when not in use. The bionanocomposites are schematically illustrated in Scheme 1.
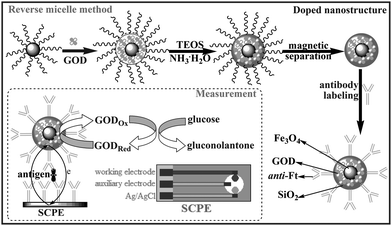 |
| Scheme 1 Synthesis and functionalization of the GOD-doped magnetic silica nanostructures, and measurement protocol. | |
2.3 Preparation and modification of screen-printed carbon electrode (SPCE)
The SPCE contained a graphite working electrode (2-mm in diameter), a Ag/AgCl reference electrode and a graphite auxiliary electrode, which was prepared using a DEK 1202 semi-automatic screen-printing machine with a 156 threads/in. polyester screen, a stainless steel flood blade, and a polyurethane squeegee (Weymouth, UK) according to the literature.33 The thickness of printed layer was about 0.5 mm. The counter electrode and the conducting track of working electrode were prepared from the graphite-CA ink via mixing 1.4 g of graphite powder in CA binder already dissolved in cyclohexanone (3.4 g of solution at 7%, w/w). The insulating layer printed around the working area constituted an electrochemical detection cell.
The electrochemical immunosensors were fabricated via cross-linking anti-Ft antibodies and thionine with BSA in a saturated glutaraldehyde vapour. First, a homogeneous mixture containing anti-Ft antibodies (100 μL, 100 μg mL−1), thionine (80 μL, 0.1 mM) and BSA (200 μL, 2%, w/v) was deposited on the sensitive area of the working electrode. Following that, the modified SPCE was placed into the saturated glutaraldehyde vapour. After 30 min, the SPCE was dried overnight at 4 °C. Subsequently, the resulting SPCE was washed 3 times with pH 7.4 M PBS containing 0.05% (w/v) Tween 20 to remove the physically absorbed biomolecules. The structure and functionalization of the SPCE are shown in Scheme 1.
2.4 Measurement protocol
Scheme 1 represents the sandwich-type immunoassay protocol and measurement method. All electrochemical measurements were carried out with a microAutoLab Type III system (Eco Chemie, The Netherlands). The measurement process contained the following steps: (i) 20 μL of standard solutions or serum samples with various concentrations was dropped to the SPCE surface, and incubated for 30 min at RT to form the antigen-antibody complex; (ii) after washing with pH 7.4 PBS, 20 μL of bionanocomposites containing secondary detection antibodies (50 mg mL−1) was added into the detection cell, and incubated for another 30 min at RT to construct a sandwich-type immunocomplex; and (iii) 50 μL of pH 6.2 PBS containing 10 mM glucose was injected into the cell, and differential pulse voltammetry (DPV) from 300 to –200 mV (vs Ag/AgCl) with a pulse amplitude of 50 mV and a pulse width of 50 ms was collected and registered as the sensor signals. Analyses are always made in triplicate.
3. Results and discussion
3.1 Characteristics of GOD-doped magnetic silica nanostructures
For the conventional sandwich-type enzyme-linked immunoassays, bioactive enzyme-labeled antibodies are usually employed as signal antibodies (i.e. detection antibodies). The detection signals mainly derive from the labeled enzyme. However, the amount of the labeled enzyme on each antibody is limited. In this study, using GOD-doped magnetic silica nanostructures for the label of secondary antibodies is expected to increase the amount of the labeled enzyme, and enhance the sensitivity of the immunoassay. Magnetic Fe3O4 nanoparticles are not only used as seeds for the growth of silica on the surface, but also favor the rapid separation and purification of the doped nanostructures. Significantly, the presence of Fe3O4 nanoparticles facilitates the GOD biomolecules to adhere on the surface of nanoparticles due to the strong interaction of protein and nanoparticles. The silica nanoparticles commendably cover the surface of Fe3O4 nanoparticles, and increase the stability of the doped GOD. Without the seeds of magnetic nanoparticles, the doped GOD molecules are mussily dispersed into the silica nanoparticles, and the exterior GOD molecules are easily leaked out during the washing.
To further monitor the synthesis of the GOD-doped magnetic silica nanostructures, we used different methods to characterize the nanostructures. First, we utilized transmission electron microscopy (TEM, H600, Hitachi Instrument, Japan) to investigate the sizes of Fe3O4 and GOD-doped magnetic silica nanostructures. Seen from Fig. 1, the mean sizes of the Fe3O4 and doped nanoparticles were 10 and 30 nm, respectively. Seen from Fig. 1b, the doped nanoparticles do not conglutinate, and still have the similar shape with the bare Fe3O4 nanoparticles. The reason might be the fact that the rich and well documented biocompatible chemistry of Fe3O4 nanoparticles may allow for practical implementation of magnetic nanoparticles during the synthesized process.
Second, we used UV–vis absorption spectrometry (Hitachi Instrument, Japan) to investigate the doped nanoparticles. Pure anti-Ft antibodies exhibit a 278 nm of absorption peak, which is the same as that of pure GOD molecules (data not shown). Almost no absorption spectra were observed for pure Fe3O4 and SiO2 colloids in the range of 600–200 nm (Fig. 2a–b). However, the GOD molecules were doped into the magnetic silica nanoparticles, a 278 nm of absorption peak was observed (Fig. 2c). Successfully, the absorption peak at 278 nm was stronger when anti-Ft antibodies were covalently bound to the GOD-doped magnetic silica nanoparticles (Fig. 2d). These results suggested that the doped nanostructures and bionanocomposites could be constructed via using the reverse micelle method and the epoxy-amino reaction.
Next, the magnetic properties of the doped nanostructures were evaluated using vibrating sample magneticmetry (VSM, model-155, Digital Measurement System Inc.) at room temperature (Fig. 3). Seen from Fig. 3, the saturation magnetizations were 59.1 and 38.9 emu g−1 for pure Fe3O4 nanoparticles and GOD-doped magnetic silica nanoparticles, respectively. The results indicated that the doped nanoparticles were still superparamagnetic, which could be used for the rapid magnetic separation and purification.
3.2 Signal comparison using various detection antibodies
To investigate the advantage of the GOD-doped magnetic silica nanoparticles in the developed immunoassays, we prepared two types of detection antibodies, i.e. GOD-conjugated anti-Ft antibodies and anti-Ft antibodies labeled with GOD-doped magnetic silica nanoparticles. During this process, we used the same SPCE immunosensors toward the same concentration of the ferritin. The measurement process is illustrated in the inset of Fig. 4. The cyclic voltammograms of the sandwich immunocomplex-modified SPCE were recorded as background currents in pH 6.2 PBS (Fig. 4a and a′), which exhibited a couple of redox peak at –10 mV and 80 mV, indicated the thionine, as a good mediator, could be immobilized on the surface of SPCE via a saturated glutaraldehyde vapour method. Upon the addition of 10 mM glucose in pH 6.2 PBS, an obvious redox reaction was appeared with a distinct decrease of the reduction current and an increase of the oxidation current (Fig. 4b and b′). Moreover, the oxidation current (ipa) increased with the increment of ferritin concentration in the sample. However, the increased currents (versus background current) using GOD-doped magnetic silica nanoparticles as detection antibodies were obviously higher than those of using GOD-labeled anti-Ft antibodies (Fig. 4). The results might be attributed to the following observation: (i) the synthesized bionanocomposites contains a large number of GOD molecules for each nanoparticle, which exhibited high catalytic efficiency; and (ii) the nanoparticles with high surface-to-volume ratio could bind more ferritin antibodies on the surface, which increased the chance of the antigen-antibody reaction. Thus, the doped nanostructures could obviously improve the sensitivity of the electrochemical immunosensors.
3.3 Optimization of external conditions
To acquire an optimal electrochemical signal, various experimental conditions, such as pH of detection solution, incubation time and incubation temperature of the antigen-antibody reaction, should be investigated. During the measurement, we should remain the bioactivity of the antigens and antibodies, and keep the high catalytic efficiency of the doped GOD. Taking 100 ng mL−1 ferritin for example, we investigated the current responses of the developed immunoassay in various pH values of the detection solution. Seen from Fig. 5a, the current responses increased with the pH increment of PBS from 2.5 to 6.0, and then decreased. So, pH 6.2 of PBS was used as the following experiments.
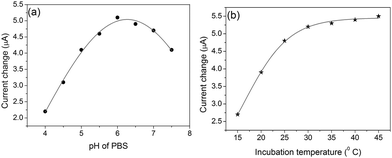 |
| Fig. 5 Effects of (a) pH of PBS and (b) incubation temperature on the current of the developed immunoassay in the presence of 100 ng mL−1 ferritin. | |
Considering the practical application of the newly developed method, the fast and sensitive analytical process is preferable. Usually, it takes some time for the antigens/antibodies in the sample to arrive at the surface of the electrode. The assay time could be often shorten via changing the incubation time, however. To adapt the common condition, all experiments were carried out at RT (25 ± 1.0 °C). At this temperature, we investigated the effect of the incubation time on the current response. As shown in Fig. 5b, the current increased with the increasing incubation time, and tended to level off after 30 min, and longer incubation time could not improve the response. Therefore, 30 min of incubation time was used for the detection of ferritin.
3.4 Analytical performance
Using the GOD-doped magnetic silica nanoparticles as detection antibodies and 10 mM glucose as enzyme substrate, we investigated the analytical performance of the developed electrochemical immunosensors based on a sandwich-type immunoassay format toward ferritin standards in pH 6.2 PBS. The currents increased with the increment of ferritin concentration in the sample (Fig. 6), which exhibits a wide linear dynamic range of 0.1 to 400 ng mL−1 and a lower detection limit (LOD) of 10 pg mL−1 ferritin at a signal to noise ratio of 3σ (where σ is the standard deviation of a blank solution, n = 13). The linear regression equation is ipa (μA) = 0.07 + 0.06 × C[ferritin] (ng mL−1, R2 = 0.996). Since the cutoff value of serum ferritin in diagnostic is 22 ng mL−1,34 the sensitivity of the developed immunoassay was enough to practical application.
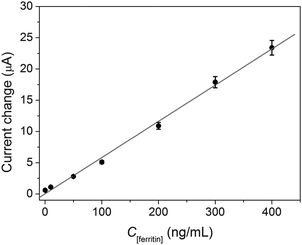 |
| Fig. 6 Calibration curves of the sandwich-type electrochemical immunoassays toward ferritin standards using GOD-doped magnetic silica nanostructures as labels in pH 6.0 PBS containing 10 mM glucose. | |
3.5 Precision and stability
The precision of the developed immunosensor was evaluated by using the variation coefficient (CV) of the intra- and inter-assays. Taking 1.0, 60 and 120 ng mL−1 ferritin for instance, the CVs of the intra-assay were 5.6%, 7.1%, and 6.2% at 1.0, 60 and 120 ng mL−1 ferritin, respectively. The inter-assay using different immunosensors and various detection antibodies with various batches were 8.1%, 7.3%, and 7.5% at 1.0, 60 and 120 ng mL−1 ferritin, respectively. Thus, the precision of the developed immunosensors and the synthesized bionanocomposites were acceptable.
When the modified SPCEs and the synthesized bionanocomposies were not in use, they could be stored at 4 °C. The detection signals were not obviously changed at the first 5-weeks, and the current was only 88.7% of the original current at 37th day. The reason might be the fact that magnetic silica nanoparticles could avoid the leakage of the doped GOD, and the ferritin antibodies could firmly immobilized on the nanoparticle surface via epoxy-amine reaction. Meanwhile, the modified method via cross-linking anti-Ft antibodies and thionine with BSA in a saturated glutaraldehyde vapour could improve the stability of the immunosensor.
3.6 Analysis of real samples
To further evaluate the possibility of the newly developed immunosensors, 10 clinical serum specimens, gifted from Chongqing Institute of Cancer Prevention and Cure, China, were assayed using the electrochemical immunosensors and referred ELISA method. The results are listed in Table 1. The relative errors of these results are less than 11.3%, which shown an acceptable agreement with these two methods. Thus, we might use the developed electrochemical immunosensor for the preliminary determination of ferritin in clinical diagnostics.
Table 1 Analytical results (ng mL−1) of clinical serum samples using the developed immunosensor and referenced ELISA method
Sample no. |
Immunosensor |
ELISA |
Mean ± SD |
% RSD |
1 |
35.6 |
41.2 |
38.4 ± 2.8 |
−7.3 |
2 |
234.6 |
221.5 |
228.1 ± 6.6 |
2.9 |
3 |
123.4 |
145.7 |
134.6 ± 11.2 |
−8.3 |
4 |
97.1 |
103.5 |
100.3 ± 3.2 |
−3.2 |
5 |
187.5 |
167.3 |
177.4 ± 10.1 |
5.7 |
6 |
19.2 |
17.8 |
18.5 ± 0.7 |
3.8 |
7 |
45.7 |
52.1 |
48.9 ± 3.2 |
−6.6 |
8 |
198.7 |
212.3 |
205.5 ± 6.8 |
−3.3 |
9 |
295.1 |
278.5 |
286.8 ± 8.3 |
2.9 |
10 |
77.9 |
69.7 |
73.8 ± 4.1 |
5.6 |
4. Conclusion
This study describes a novel nanoparticle-labeled method for secondary detection antibodies in the sandwich-type electrochemical immunoassay using GOD-doped magnetic silica nanostructures. The strategy could be conveniently used for ultrasensitive detection of ferritin in serum samples in combination with a modified SPCE electrode. The bionanoparticle labels and the substrate electrode could be synthesized and prepared using the reverse micelle method and the vapour-linked method, respectively. Compared with the conventional label method, the doped nanostructures greatly improved the sensitivity of the developed immunoassay. Meanwhile, the method displays good performance with a wide working range and a low detection limit, and acceptable stability, reproducibility and accuracy. Including, the doped nanoparticles could provide a new platform and a promising application in the nanostructures-based label methods.
Acknowledgements
This work was financially supported by the National Basic Research Program of China (2010CB732403), the National Natural Science Foundation of China (20877019 and 20735002), the Key Natural Sciences Foundation of Fujian Province, China (D0520001), the Key Program of Science and Technology Department of Fujian Province, China (2007Y0026), NTU-MOE Academic Research Funds (RG65/08).
Notes and references
- S. Gaueda, A. Podborska, W. Macyk and K. Owski, Nanoscale, 2009, 1, 299 RSC.
- A. Kaniyoor, R. Jafri, T. Arockiadoss and S. Ramaprabhu, Nanoscale, 2009, 1, 382 RSC.
- S. Pearton, F. Ren, Y. Wang, B. Chu, K. Chen, C. Chang, W. Lim and D. Norton, Prog. Mater. Sci., 2010, 55, 1 CrossRef.
- A. Barnard, Nanoscale, 2009, 1, 89 RSC.
- S. Roy and Z. Gao, Nano Today, 2009, 4, 318 CrossRef.
- J. Johnson, Y. Choi, A. Ural, W. Lim, J. Wright, B. Gila, F. Ren and S. Pearton, J. Electron. Mater., 2009, 38, 490 CrossRef CAS.
- K. Cotí, M. Belowich, M. Liong, M. Ambrogio, Y. Lau, H. Khatib, J. Zink, N. Khashab and J. Stoddart, Nanoscale, 2009, 1, 16 RSC.
- W. Lim, J. Wright, B. Gila, S. Pearton, F. Ren, W. Lai, L. Chen and K. Chen, Appl. Phys. Lett., 2008, 93 art. No. 202109.
- D. Norris, A. Efros and S. Erwin, Science, 2008, 319, 1776 CrossRef CAS.
- R. Davies, C. Abernathy, S. Pearton, D. Norton, M. Ivill and F. Ren, Chem. Eng. Commun., 2009, 196, 1030 CrossRef CAS.
- J. Wright, W. Lim, B. Gila, S. Pearton, J. Johnson, A. Ural and F. Ren, Sens. Actuators, B, 2009, 140, 196 CrossRef.
- A. Polyakov, A. Govorkov, N. Smirnov, A. Markov, I. Lee, J. Ju and S. Pearton, Appl. Phys. Lett., 2009, 94 art. No. 142103.
- X. Xie and W. Shen, Nanoscale, 2009, 1, 50 RSC.
- N. Sakamoto, H. Ohtsuka, T. Ikeda, K. Maeda, D. Lu, M. Kanehara, K. Teramura, T. Teranishi and K. Domen, Nanoscale, 2009, 1, 106 RSC.
- T. Pui, A. Agarwai, F. Ye, Z. Tou, Y. Huang and P. Chen, Nanoscale, 2009, 1, 159 RSC.
- R. Serda, S. Ferrati, B. Godin, E. Tasciotti, X. Liu and M. Ferrari, Nanoscale, 2009, 1, 250 RSC.
- Y. Jin, A. Li, S. Hazelton, S. Liang, C. John, P. Selid, D. Pierce and J. Zhao, Coord. Chem. Rev., 2009, 253, 2998 CrossRef CAS.
- Z. Santoyo-Gonzalez and M. Hernandez, Chem. Soc. Rev., 2009, 38, 3449 RSC.
- Ee. Benvenutti, C. Moro, T. Costa and M. Gallas, Quimica Nova, 2009, 32, 1926 Search PubMed.
- D. Knopp and D. Tang, Anal. Chim. Acta, 2009, 647, 14 CrossRef CAS.
- D. Tang, Z. Zhong, R. Niessner and D. Knopp, Analyst, 2009, 134, 1554 RSC.
- D. Tang and J. Ren, Anal. Chem., 2008, 80, 8064 CrossRef CAS.
- D. Tang, R. Yuan and Y. Chai, Anal. Chem., 2008, 80, 1582 CrossRef CAS.
- R. Davies, M. Ivill, J. Hilc, B. Gila, G. Thaler, C. Abernathy, S. Pearton and J. Zavada, Mater. Res. Soc. Symp. Proc., 2009, 1111, 77.
- J. Chen, C. Li, W. Zhou, Q. Yan, L. Archer and X. Lou, Nanoscale, 2009, 1, 280 RSC.
- D. Tang, J. Sauceda, Z. Lin, S. Ott, E. Basova, I. Goryacheva, S. Biselli, J. Lin, R. Niessner and D. Knopp, Biosens. Bioelectron., 2009, 25, 514 CrossRef CAS.
- S. Müller, Nanomed.: Nanotechnol., Biol. Med., 2009, 5, 387 CrossRef; G. Papaefthymiou, Nano Today, 2009, 4, 438 CrossRef CAS.
- D. Tang, R. Yuan, Y. Chai and H. An, Adv. Funct. Mater., 2007, 17, 976 CrossRef CAS.
- I. Koh and L. Josephson, Sensors, 2009, 9, 8130 CrossRef CAS.
- J. Corchero and A. Villaverde, Trends Biotechnol., 2009, 27, 468 CrossRef CAS.
- P. Bawa, V. Pillay, Y. Choonara and L. Du Toit, Biomed. Mater., 2009, 4, 022001 Search PubMed art. No. 022001.
- L. Wang, L. Li, Y. Xu, G. Cheng, P. He and Y. Fang, Talanta, 2009, 79, 557 CrossRef CAS.
- G. Lai, F. Yan and H. Ju, Anal. Chem., 2009, 81, 9730 CrossRef CAS.
- C. Choi, W. Cho, K. Park, I. Choi, J. Seo, B. Kim, S. Shin, Y. Kim, J. Kim and J. Lee, Ann. Hematol., 2005, 84, 358 CrossRef CAS.
|
This journal is © The Royal Society of Chemistry 2010 |