DOI:
10.1039/C005387M
(Paper)
Metallomics, 2010,
2, 469-473
Free cadmium ions released from CdTe-based nanoparticles and their cytotoxicity on Phaeodactylum tricornutum†
Received
12th February 2010
, Accepted 26th May 2010
First published on
10th June 2010
Abstract
The risk of nanoparticles (NPs) to organisms and the environment has become more noticeable alongside their rapid applications in many fields. The release of Cd2+ from CdTe-based NPs (CdTe-NPs), an important class of engineered nanomaterials, is one of the possible factors responsible for the cytotoxicity of these NPs. Based on the same CdTe core, CdTe/CdS, CdTe/ZnS and CdTe/SiO2 NPs were synthesized and their Cd2+ release rates were carefully studied based on dialysis using inductively coupled plasma mass spectrometry (ICPMS). Results obtained indicated that the Cd2+ release rates of the CdTe-NPs decreased in the order CdTe (8.78 ng mL−1 mg−1 h−1) > CdTe/CdS (2.63) > CdTe/SiO2 (0.89) > CdTe/ZnS (0.72). Phaeodactylum tricornutum was used as a model diatom for evaluating the cytotoxicity of the CdTe-NPs. Results obtained from the CdTe-NPs exposure experiments together with ICPMS and fluorescence microscopy studies suggested that the cytotoxicity of the CdTe-NPs increased along with the increase in their Cd2+ release rates. Effective coating materials such as ZnS and SiO2 for the CdTe core significantly reduced the cytotoxicity of CdTe.
Introduction
Accompanying the rapid development of nanotechnology, more and diverse applications are found for nanoparticles (NPs) due to the ongoing-discovery of new properties, with all the concomitant worries concerning their potential adverse effects on organisms and the environment.1–5. Cadmium telluride (CdTe), as an example, is composed of semiconductor nanocrystals (2–100 nm).1 These NPs, also known as CdTe quantum dots (QDs), are preferred for potential medical, diagnostic and other basic research applications because of their unique intrinsic photophysical properties. Compared with traditional organic dyes, they are superior in many respects, such as their strong photostability and also fluorescence wavelength tunable by size.6,7 Although the QDs have been synthesized and their corresponding properties researched for more than twenty years, the cytotoxicity and potential adverse effects on organisms and the environment have only been of concern in recent years. Factors including components, concentration, size, charge, outer coating, oxidisability and stability are considered as comprehensive reasons for their toxicity.1,2 In the case of the CdTe-based NPs (CdTe-NPs), the release of free cadmium ions (Cd2+) is considered to be a major factor causing cytotoxicity. The possible toxicity mechanisms of Cd2+ include interference with DNA repair and Zn2+ substitution during protein metabolism and the induction of reactive oxygen species (ROS) formation. Moreover, this ion can be accumulated in the human body with a half-life exceeding 10 years, causing renal dysfunction and pulmonary emphysema, and it is also a suspected carcinogen.8,9 In order to reduce the cytotoxicity but enhance the stability and biocompatibility of CdTe-NPs, different coating methods for bare CdTe have been developed. However, the discrepancy among different kinds of core/shell structures in restricting the release of Cd2+ has not been systematically investigated and reported so far.
Recently, inductively coupled plasma mass spectrometry (ICPMS) has been used for analyzing impurities and the elemental composition in NPs, demonstrating its advantages of excellent sensitivity and precision.10–13 In our study, in order to evaluate the discrepancy in the Cd2+ release rate between CdTe-NPs with or without different coating shells, we synthesized CdTe, CdTe/CdS, CdTe/ZnS and CdTe/SiO2 NPs. Subsequently, the Cd2+ release rate of the CdTe-NPs was studied in a novel way based on the principle of dialysis and using ICPMS. Phaeodactylum tricornutum, a typical unicellular diatom, was used as a model organism to evaluate for the first time the cytotoxicity of the CdTe-NPs.
Experimental
Reagents and chemicals
Cadmium chloride (CdCl2·2.5 H2O, ≥98%), tellurium (Te powder, 99.8%) and zinc chloride (ZnCl2, ≥98%) were purchased from Sigma-Aldrich (St. Louis, MO, USA); sodium sulfide (Na2S, ≥ 98%), sodium borohydride (NaBH4, 96%) and thioglycolic acid (TGA, C2H4O2S, 98%) were purchased from Sinopharm Chemical Reagent Co. Ltd. (Shanghai, China); Triton X-100 (C34H62O11) was purchased from Shanghai Sangon Biological Engineering Technology & Services Co. Ltd. (Shanghai, China); and tetraethyl orthosilicate (C8H20O4Si, 98%) was purchased from Acros (Geel, Belgium). Nitric acid (HNO3, 65%, p.a. grade, Darmastadt, Germany) was used for sample digestion. Other reagents used were at least of analytical grade in this study. The solutions were all prepared with ultra-pure water (18.2 MΩ cm obtained from Pen-Tung Sah Micro-Electro-Mechanical Systems Research Center of Xiamen University, China).
Instrumentation
Absorption and fluorescence spectra were recorded on a Varian Cary 300 spectrophotometer and Hitachi F-4500 fluorescence spectrophotometer in a 1-cm quartz cell. The fluorescence spectra were measured with an excitation wavelength of 405 nm for the CdTe-NPs using a xenon lamp of 150 W, and the slits for the monochromators were 5 nm. The hydrodynamic diameters of the CdTe-NPs were measured using dynamic light scattering (DLS) with a Malvern Zetasizer Nano-ZS (Malvern Instruments Ltd., Worcestershire, UK). The fluorescence microscopy experiment was performed using a Leica TCS SP5 laser scanning confocal microscope (Leica Microsystems CMS GmbH, Wetzlar, Germany), equipped with an acousto-optical beam splitter. The CdTe-NPs were excited with the 405 nm laser line of a diode laser and detected using a bandpass of 470–630 nm.
For elemental analysis, samples were analyzed using an ELAN DRC II ICPMS (PerkinElmer, SCIEX, Canada). The instrument was equipped with an integral peristaltic pump, a concentric pneumatic nebulizer, and a cyclonic spray chamber. Standard solutions of Cd, Te, Zn and Si were used for calibration, and data acquisition and processing were performed using ELAN v3.3 software (PerkinElmer, SCIEX). Detailed instrumental settings for the ICPMS system are listed in Table S1, ESI.†
Preparation and analysis procedures
Synthesis of the water-soluble CdTe-NPs.
TGA-capped CdTe-NPs were prepared as described elsewhere.14 Briefly, fresh NaHTe solution was prepared with Te powder (0.1276 g) and NaBH4 (0.080 g) in 3 mL ultra-pure water under N2 gas protection. Then, the NaHTe solution was added to 100 mL N2-saturated solution (pH 10) containing Cd2+ (2 mmol L−1) and TGA (4.8 mmol L−1), yielding the precursor solution for the CdTe nanocrystals. The precursor solution was refluxed at 100 °C for 3 h to grow CdTe.
For coating the bare CdTe, shell synthesis methods were employed.15,16 Briefly, equal molar ZnCl2 and Na2S solutions were injected dropwise into the whisked CdTe solution, and the solution refluxed at 100 °C to grow for 1 h to obtain the CdTe/ZnS-NPs; equal molar CdCl2 and Na2S solutions were injected dropwise into the whisked CdTe solution, and the solution refluxed at 100 °C to grow for 1 h to obtain the CdTe/CdS-NPs; and 4 mL CdTe solution was added into a mixed solution of cyclohexane (37.5 mL), n-hexanol (9 mL), Triton X-100 (8.85 mL), tetraethyl orthosilicate (1 mL) and ammonia aqueous solution (0.25 mL) with stirring for 24 h at 25 °C to obtain the CdTe/SiO2-NPs.
Dialysis and elemental analysis.
Before dialysis, the synthesized CdTe-NPs were washed with ethanol and water three times via repeated centrifugation (5000 rpm, 10 min) and sonication (2 min). Then the CdTe-NPs were sonicated and resuspended in an appropriate volume of phosphate-buffered saline (PBS) at pH 7.4. The exact Cd concentrations of CdTe, CdTe/CdS, CdTe/ZnS and CdTe/SiO2 NPs solutions were measured using ICPMS after digestion with 65% HNO3.
The CdTe-NPs (2 mL, CCd = 0.1 g L−1), including CdTe, CdTe/CdS, CdTe/ZnS and CdTe/SiO2, were separately added into dialysis tubes (molecular-weight cutoff of 500 daltons, MWCO-500, Shanghai Sangon Biological Engineering Technology & Services Co. Ltd.). The dialysis tubes were placed in polypropylene beakers instead of glass beakers containing 400 mL PBS, thus avoiding the possible adsorption of metal ions, and 2 mL of each was taken every 12 h. The dialysis experiment lasted for 120 h, and each experiment was repeated 3 times for each of the CdTe-NPs synthesized in this study. Finally, all of the collected samples were analyzed using ICPMS.
Cytotoxicity evaluation and fluorescence microscopy study.
P. tricornutum was obtained from the Center for the Collection of Marine Bacteria and Phytoplankton in the State Key Laboratory of Marine Environmental Science at Xiamen University. It was cultured in natural seawater, with the addition of f/2 enrichment solution17 (f/2 solution contains major nutrients including nitrate and phosphate, essential trace elements, and vitamins) at 20 ± 1 °C under axenic conditions and illuminated using fluorescent daylight (3000 Lux) tubes. During the production process, daily shaking of each culture was performed to increase the growth rate of P. tricornutum. The CdTe-NPs exposure experiments were performed in natural seawater at pH 7.5 adjusted using 3-N-morpholino-propane-sulfonic acid (0.01 mol L−1) containing f/2 solution. Culture solutions (200 mL) were sterilized (autoclaving and 0.45 μm filtration) prior to use. Solutions of CdCl2, CdTe, CdTe/CdS, CdTe/ZnS and CdTe/SiO2 were added into the culture solutions and the initial Cd concentrations in the culture solutions were controlled (100, 200 and 300 ng mL−1). Each day, 4 mL of P. tricornutum cell suspensions was taken out of each culture solution and harvested using gentle centrifugation. These experiments continued for six days.
The optical density of the P. tricornutum suspensions was measured using a spectrophotometer at 665 nm. The P. tricornutum cells in the 4 mL suspensions were collected using centrifugation at 10000 rpm for 10 min. They were washed three times with seawater (pH 7.5) to remove any substrate. Then, the samples were digested with 1 mL concentrated HNO3 overnight, and diluted with 1 mL ultrapure water. Finally, the Cd content inside the cell was determined using ICPMS.
For the fluorescence microscopy experiment, P. tricornutum cells were placed on a glass microscope slide and covered with a glass slip. The red fluorescence of the CdTe-NPs was detected.
Results and discussion
Characterization of the water-soluble CdTe-NPs and their Cd2+ release rates
The fluorescence spectra, hydrodynamic diameters and elemental composition of the synthesized CdTe-NPs are illustrated in Fig. 1 and detailed data are listed in Table 1. The maximum emission wavelengths of the CdTe-NPs exhibited red shift for CdTe/CdS and CdTe/ZnS but a blue shift for CdTe/SiO2, which matched those reported previously.15,16 DLS studies indicated that their size increased in the order CdTe (5.88 nm) < CdTe/CdS (6.23 nm) < CdTe/ZnS (6.59 nm) < CdTe/SiO2 (13.54 nm). Their elemental composition measured using ICPMS indicated that the synthesized CdTe contained Cd 664.7 ± 7.2 and Te 322.3 ± 2.1 μg mg−1; the CdTe/CdS Cd 679.3 ± 5.9 and Te 303.2 ± 1.8 μg mg−1; the CdTe/ZnS Cd 624.3 ± 3.6, Te 297.6 ± 1.4 and Zn 14.86 ± 0.57 μg mg−1; and the CdTe/SiO2 Cd 584.7 ± 5.5, Te 286.2 ± 4.4 and Si 73.38 ± 1.6 μg mg−1. Cd contents among the CdTe-NPs were almost at the same level, suggesting that their CdTe cores were uniform. This is very important for studying and explaining the discrepancy in the Cd2+ release rate among differently shelled CdTe-NPs.
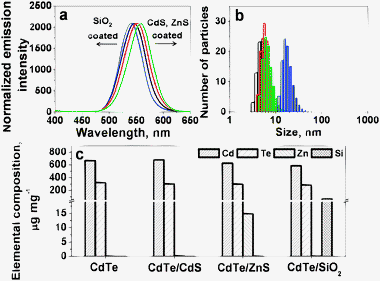 |
| Fig. 1 (a) Normalized fluorescence emission spectra of the CdTe-NPs synthesized, with an excitation wavelength of 405 nm; (b) and (c) are size distribution and elemental composition of the CdTe-NPs. In (a) and (b), black, red, green and blue represent CdTe, CdTe/CdS, CdTe/ZnS and CdTe/SiO2. | |
Table 1 Properties of CdTe-NPs and their Cd2+ release rates
Shell |
— |
CdS |
ZnS |
SiO2 |
Maximal emission/nm |
546 |
552 |
558 |
540 |
Hydrodynamic diameter/nm |
5.88 |
6.23 |
6.59 |
13.54 |
Release rate of Cd2+/ng mL−1 mg−1 h−1 |
8.78 |
2.63 |
0.72 |
0.89 |
In order to investigate the Cd2+ release rate of the CdTe-NPs, a dialysis membrane strategy was used which works on the principle of the diffusion of solutes and ultrafiltration of fluid across a semi-permeable membrane. The MWCO-500 used in this study ensured that the Cd2+ passed through the membrane while the CdTe-NPs were held inside the dialysis tube. After five days dialysis, no obvious aggregation occurred, suggesting that the CdTe-NPs were stable during the dialysis experiments.
The Cd2+ release rate was evaluated based on the Cd2+ concentration (ng mL−1) change in the dialysis buffer solution in one hour for 1 mg of the CdTe-NPs. The samples collected in each 12-hour interval were analyzed using ICPMS. The mechanism of Cd2+ release from Cd-based NPs is proposed to be the result of surface oxidation, and Cd release can be considered as a first order reaction.18,19 The results shown in Fig. 2(a) indicated that the release rate of Cd (8.78 ng mL−1 mg−1 h−1) from the bare CdTe-NPs is much greater than that for Te (0.12 ng mL−1 mg−1 h−1), and good linear relationships between Cd and Te concentration and the dialysis time were obtained for both Cd (R2 = 0.9980) and Te (R2 = 0.9659). The big difference between the release rates of Cd and Te might be attributed to the different oxidation mechanisms they undergo. It has been postulated that O2 molecules oxidize the chalcogenide atoms (S, Se, Te) located on the surface of the NPs to form oxides. In the case of CdTe-NPs, Cd forms Cd2+ while Te forms TeO2 on the surface.19 The Cd2+ ions formed may diffuse into the dialysis solution, but the TeO2 formed can hardly dissolve in the dialysis solution, resulting that in a much lower Te concentration and release rate compared with Cd, as demonstrated in Fig. 2(a). Furthermore, Fig. 2(b) demonstrates the linear relationship between the Cd concentration in the dialysis buffer solution and the dialysis time, that is, y = 10.10 + 4.83x (R2 = 0.9980) for CdTe; y = 1.74 + 0.10x (R2 = 0.9629) for CdTe/CdS; y = 0.55 + 0.03x (R2 = 0.9606) for CdTe/SiO2; and y = 1.23 + 0.02x (R2 = 0.9700) for CdTe/ZnS, suggesting that the corresponding Cd2+ release rate decreased in the order of CdTe (8.78 ng mL−1 mg−1 h−1) >CdTe/CdS (2.63) > CdTe/SiO2 (0.89) >CdTe/ZnS (0.72). The detailed values are also listed in Table 1.
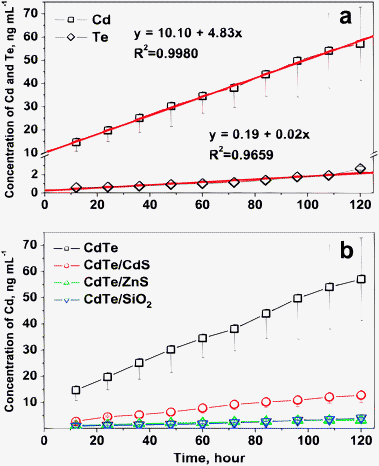 |
| Fig. 2 (a) The concentration changes of Cd and Te released from bare CdTe-NPs in dialysis solution over time; (b) Cd released from CdTe, CdTe/CdS, CdTe/ZnS and CdTe/SiO2 in dialysis solution over time. | |
Uptake of the CdTe-NPs and their cytotoxicity on P. tricornutum
Diatoms, which are found throughout marine and fresh water environments, belong to a family of unicellular algae. They are believed to be responsible for around one-fifth of the global carbon fixation on Earth, and are the initial link of the food chain in water. Representing one of two major classes of diatoms, P. tricornutum is the most widely utilized as a model for studying the ecology, physiology, biochemistry and molecular biology of diatoms.20,21 The tolerance of P. tricornutum to Cd2+ has been studied,22,23 and the results obtained suggest that P. tricornutum has a detoxifying mechanism for chelating Cd2+ by in vivo synthesized phytochelatins (PCs) and proteins similar to that of higher plants.24–26 However, the cytotoxicity of CdTe-NPs to this typical diatom has not been reported. P. tricornutum cells are encased within a unique cell wall composed of hydrated silicon dioxide. The cell walls are semipermeable, allowing the passage of small molecules while limiting the passage of larger molecules. The diameter of pores across the cell wall, which ranges from 5 to 20 nm, determines its sieving properties.4 After passing through the cell wall, the CdTe-NPs meet the cell membrane which is also semipermeable and composed primarily of proteins and lipids. Besides the possibility that CdTe-NPs might cross the cell membrane through embedded transport carrier proteins or ion channels, the potential entry routes of the CdTe-NPs through this lipid membrane are supposed to be endocytic pathways. During this process, the cell membrane forms a cavity-like structure that surrounds the CdTe-NPs and pulls them into the cell via endocytosis.4 The uptake of the four CdTe-NPs synthesized in this study into P. tricornutum cells were confirmed using fluorescence microscopy, as shown in Fig. 3 and Fig. S1, ESI.† The image (Fig. 3c) shows that the red fluorescence of the CdTe-NPs can only be observed in the cytoplasm of P. tricornutum cells, and no absorption can be observed on the surface of the cell walls. Obviously, the concentration of the CdTe-NPs inside the cells is much higher than that in the culture solution (Fig. 3b).
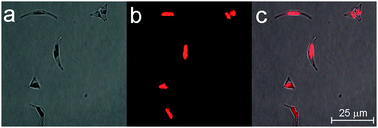 |
| Fig. 3 (a), (b) and (c) are the transmitted, fluorescence and merged microscopic images of P. tricornutum cells on the sixth day of exposure to the bare CdTe-NPs (300 ng mL−1). | |
The cytotoxicity of the CdTe-NPs on P. tricornutum was evaluated and compared with the control group (P. tricornutum cells were cultured in natural seawater but then treated in exactly the same way as the CdTe-NPs exposed groups). The growth curve of P. tricornutum was employed to indicate their cytotoxicity to this diatom. The cell number can be estimated from the specific absorption at 665 nm of chlorophyll a, in which the optical density and the cell number counted using microscopy are in good linear correlation (Fig. S2, ESI†). The optical densities of the control and exposed P. tricornutum suspensions were measured daily, and their growth curves were constructed and are compared in Fig. 4. To study the effect of CdTe-NPs concentration on the growth of P. tricornutum, the cells were exposed to different concentrations of the CdTe-NPs (corresponding to 100, 200 and 300 ng mL−1 Cd initially in the culture solutions), together with equal CdCl2 concentration exposed groups for comparison. Fig. 4 shows that there were obvious differences among their growth curves after the addition of CdCl2 and the CdTe-NPs. The cell numbers decreased 5.6, 28.6, 16.2, 4.5 and 11.8% for CdCl2, CdTe, CdTe/CdS, CdTe/ZnS and CdTe/SiO2 exposed groups (300 ng mL−1) on the sixth day, when compared with the control group. Based on these experimental results, we can conclude their cytotoxicity to P. tricornutum cells decreases in the order CdTe >CdTe/CdS >CdTe/SiO2 >CdCl2 >CdTe/ZnS. This result was in accordance with the Cd2+ release rates of the CdTe-NPs. Compared with the group exposed to CdCl2, the CdTe-NPs were more toxic to P. tricornutum cells, except for CdTe/ZnS. The fact that the latter has an effective core/shell structure that could inhibit Cd2+ release from the CdTe core and ROS formation on the nanoparticle surface27 as well as the possible positive effect of the Zn2+ released, might be responsible for this exception.
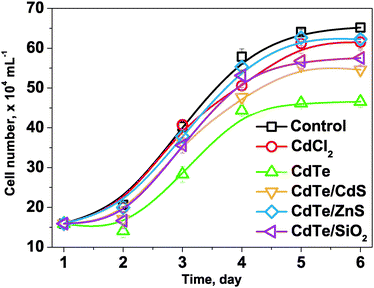 |
| Fig. 4 Growth curves of P. tricornutum cells in the control group and exposed groups (CdCl2, CdTe, CdTe/CdS, CdTe/ZnS and CdTe/SiO2) at the initial Cd concentration of 300 ng mL−1. | |
Subsequently, the uptake of CdTe-NPs in P. tricornutum cells was analyzed using ICPMS. The Cd contents per cell under different exposure conditions on the sixth day are illustrated in Fig. 5. In the case of the 300 ng mL−1 initial Cd exposure, the Cd contents reached 0.007 ± 0.001, 0.244 ± 0.021, 0.076 ± 0.004, 0.058 ± 0.003 and 0.043 ± 0.008 pg cell−1 for CdCl2, CdTe, CdTe/CdS, CdTe/ZnS and CdTe/SiO2. The Cd content inside the cell of the CdTe exposed group was much higher than that in the other groups, suggesting that the uncoated and smallest CdTe might more easily have been taken up by the cells, which in turn released more Cd2+ inside the cells than the others did. This was in accordance with the results observed in the cytotoxicity studies. Moreover, it should be noted that the uptake of Cd by P. tricornutum cells in the case of CdCl2 exposure was the lowest compared with those of the CdTe-NPs, suggesting that P. tricornutum take up Cd2+ and CdTe-NPs in different ways, in which that Cd2+ is taken up via regulation of the ion-channels on the cell membrane23 while CdTe-NPs are more easily taken up via cell endocytosis and release of Cd2+ inside the cells thus causing damage.
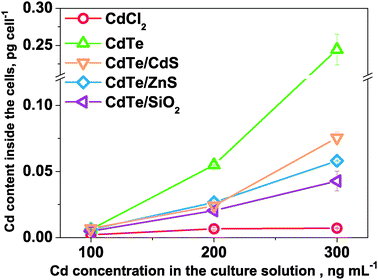 |
| Fig. 5 The Cd contents inside P. tricornutum cells with respect to the Cd concentration in the culture solutions on the sixth day. | |
Conclusions
Based on the same CdTe core, CdTe/CdS, CdTe/ZnS and CdTe/SiO2 NPs were synthesized, and the Cd2+ release rates of these four CdTe-NPs were carefully studied based on dialysis experiments using ICPMS. Although the uptake pathways of the CdTe-NPs need more intensive studies, their cytotoxicity to P. tricornutum clearly suggested positive relationship between the Cd2+ release rate and cytotoxicity, and that effective coating materials such as ZnS and SiO2 shells could significantly reduce the cytotoxicity of the CdTe core. Further studies concerning the sulfhydryl-riched peptides and proteins generated in vivo by the Cd2+ released from the CdTe-NPs, and their interactions, are ongoing in our laboratory.
Acknowledgements
This study was financially supported by the National Natural Science Foundation of China (20775062) and the National Basic Research Program of China (2009CB421605). Prof. John Hodgkiss is thanked for his assistance with the English.
References
- R. Hardman, Environ. Health Perspect., 2006, 114, 165–172 CrossRef.
- H. C. Li, W. R. Luo, T. Yong, Y. Wu, X. F. Lv, Q. F. Zhou and G. B. Jiang, Sci. China Chem., 2009, 52, 1683–1690 Search PubMed.
- B. Nowack and T. D. Bucheli, Environ. Pollut., 2007, 150, 5–22 CrossRef CAS.
- E. Navarro, A. Baun, R. Behra, N. B. Hartmann, J. Filser, A. Miao, A. Quigg, P. H. Santschi and L. Sigg, Ecotoxicology, 2008, 17, 372–386 CrossRef CAS.
- M. Farré, K. G. Schrantz, L. Kantiani and D. Barceló, Anal. Bioanal. Chem., 2009, 393, 81–95 CrossRef CAS.
- M. Jr. Bruchez, M. Moronne, P. Gin, S. Weiss and A. P. Alivisatos, Science, 2005, 307, 538–544 CrossRef CAS.
- X. Michalet, F. F. Pinaud, L. A. Bentolila, J. M. Tsay, S. Doose, J. J. Li, G. Sundaresan, A. M. Wu, S. S. Gambhir and S. Weiss, Science, 1998, 281, 2013–2016 CrossRef CAS.
- J. P. Buchet, R. Lauwerys, H. Roels, A. Bernard, P. Bruaux, F. Claeys, G. Ducoffre, P. de Plaen, J. Staessen, A. Amery, P. Lijnen, L. Thijs, D. Rondia, F. Sartor, A. Saint-Remy and L. Nick, Lancet, 1990, 336, 699–702 CrossRef CAS.
- J. H. Priester, P. K. Stoimenov, R. E. Mielke, S. M. Webb, C. Ehrhardt, J. P. Zhang, G. D. Stucky and P. A. Holden, Environ. Sci. Technol., 2009, 43, 2589–2594 CrossRef CAS.
- A. Helfrich, W. Brüchert and J. Bettmer, J. Anal. At. Spectrom., 2006, 21, 431–434 RSC.
- C. Engelhard, T. Vielhaber, A. Scheffer, M. Brocksieper, W. Buscher and U. Karst, J. Anal. At. Spectrom., 2008, 23, 407–411 RSC.
- C. C. Ge, F. Lao, W. Li, Y. F. Li, C. Y. Chen, X. Y. Mao, B. Li, Z. F. Chai and Y. L. Zhao, Anal. Chem., 2008, 80, 9426–9434 CrossRef CAS.
- A. R. M. Bustos, J. R. Encinar, M. T. Fernández-Argüelles, J. M. Costa-Fernández and A. Sanz-Medel, Chem. Commun., 2009, 3107–3109 RSC.
- H. Zhang, D. Wang, B. Yang and H. Mhwald, J. Am. Chem. Soc., 2006, 128, 10171–10180 CrossRef CAS.
- X. Wang, J. Huang, R. Jin, Z. Yang, Z. Shan and W. Yang, Acta. Chimica. Sinica., 2009, 67, 2025–2030 Search PubMed.
- J. Lin, D. Yu, Y. Liu, X. Zhao and J. Zhou, Talanta, 2008, 76, 1053–1057 CrossRef CAS.
-
R. R. L. Guillard, Culture of Marine In-vertebrate Animals, Plenum Press, New York, 1975, p. 26–60 Search PubMed.
- A. M. Derfus, W. C. W. Chan and S. N. Bhatia, Nano Lett., 2004, 4, 11–18 CrossRef CAS.
- X. Yi and J. J. Liou, Solid-State Electron., 1995, 38, 1151–1154 CrossRef CAS.
- K. E. Apt, P. G. Kroth-Pancic and A. R. Grossman, Mol. Gen. Genet., 1996, 252, 572–579 CAS.
- C. Bowler, A. E. Allen, J. H. Badger, J. Grimwood, K. Jabbari, A. Kuo, U. Maheswari, C. Martens, F. Maumus, R. P. Otillar, E. Rayko, A. Salamov, K. Vandepoele, B. Beszteri, A. Gruber, M. Heijde, M. Katinka, T. Mock, K. Valentin, F. Verret, J. A. Berges, C. Brownlee, J. P. Cadoret, A. Chiovitti, C. J. Choi, S. Coesel, A. De Martino, J. C. Detter, C. Durkin, A. Falciatore, J. Fournet, M. Haruta, M. J. Huysman, B. D. Jenkins, K. Jiroutova, R. E. Jorgensen, Y. Joubert, A. Kaplan, N. Kröger, P. G. Kroth, J. La Roche, E. Lindquist, M. Lommer, V. Martin-Jézéquel, P. J. Lopez, S. Lucas, M. Mangogna, K. McGinnis, L. K. Medlin, A. Montsant, M. P. Oudot-Le Secq, C. Napoli, M. Obornik, M. S. Parker, J. L. Petit, B. M. Porcel, N. Poulsen, M. Robison, L. Rychlewski, T. A. Rynearson, J. Schmutz, H. Shapiro, M. Siaut, M. Stanley, M. R. Sussman, A. R. Taylor, A. Vardi, P. von Dassow, W. Vyverman, A. Willis, L. S. Wyrwicz, D. S. Rokhsar, J. Weissenbach, E. V. Armbrust, B. R. Green, Y. Van de Peer and I. V. Grigoriev, Nature, 2008, 456, 239–244 CrossRef CAS.
- E. Torres, A. Cid, P. Fidalgo, C. Herrero and J. Abalde, Aquat. Toxicol., 1997, 39, 231–246 CrossRef CAS.
- D. F. Si, L. M. Yang, H. Yan and Q. Q. Wang, Sci. China Chem., 2009, 52, 2373–2380 Search PubMed.
- A. P. Navaza, M. Montes-Bayon, D. L. Leduc, N. Terry and A. Sanz-Medel, J. Mass Spectrom., 2006, 41, 323–331 CrossRef CAS.
- L. Q. Chen, Y. F. Guo, L. M. Yang and Q. Q. Wang, J. Anal. At. Spectrom., 2007, 22, 1403–1408 RSC.
- L. Q. Chen, Y. F. Guo, L. M. Yang and Q. Q. Wang, Chin. Sci. Bull., 2008, 53, 1503–1511 CrossRef CAS.
- B. I. Ipe, M. Lehnig and C. M. Niemeyer, Small, 2005, 1, 706–709 CrossRef CAS.
Footnote |
† Electronic supplementary information (ESI) available: Optimum operating parameters for ICPMS, fluorescence microscopic images of the P. tricornutum cells exposed to CdTe and the linear correlation between the optical density and the P. tricornutum cell number counted using microscopy. See DOI: 10.1039/c005387m |
|
This journal is © The Royal Society of Chemistry 2010 |
Click here to see how this site uses Cookies. View our privacy policy here.